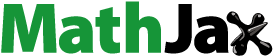
ABSTRACT
Introduction
Inhaled gene therapy programs targeting diseases of the lung have seen increasing interest in recent years, though as of yet no product has successfully entered the market. Preclinical research to support such programs is critically important in maximizing the chances of developing successful candidates.
Areas covered
Aspects of inhalation delivery of gene therapies are reviewed, with a focus on preclinical research in animal models. Various barriers to inhalation delivery of gene therapies are discussed, including aerosolization stresses, aerosol behavior in the respiratory tract, and disposition processes post-deposition. Important aspects of animal models are considered, including determinations of biologically relevant determinations of dose and issues related to translatability.
Expert opinion
Development of clinically-efficacious inhaled gene therapies has proven difficult owing to numerous challenges. Fit-for-purpose experimental and analytical methods are necessary for determinations of biologically relevant doses in preclinical animal models. Further developments in disease-specific animal models may aid in improving the translatability of results in future work, and we expect to see accelerated interests in inhalation gene therapies for various diseases. Sponsors, researchers, and regulators are encouraged to engage in early and frequent discussion regarding candidate therapies, and additional dissemination of preclinical methodologies would be of immense value in avoiding common pitfalls.
1. Introduction
An explosion of interest in gene therapy modalities has occurred over the past few decades as applications for treating disease are explored and refined [Citation1]. In 2019, the U.S. Food and Drug Administration (FDA) Center for Biologics Evaluation and Research (CBER) predicted the approval of 10 to 20 cell and gene therapy products per year by 2025 [Citation2]. There were, at the time of writing in April 2023, 28 FDA-approved cellular and gene therapy products in the United States [Citation3], and the FDA has recently modified organizational structures to better handle the increasing number of product reviews associated with expanded interest in cellular and gene therapies [Citation4]. The development and finalization of FDA guidance specific to gene therapies is likely to further accelerate efforts by sponsors, researchers, and regulators to successfully bring gene therapies from concept to clinic [Citation1].
The FDA considers gene therapies to be ‘all products that mediate their effects by transcription or translation of transferred genetic material or by altering host (human) genetic sequences’ [Citation5], a broad definition that encompasses a huge diversity in methods and technologies. With gene therapies, the aim is often to deliver a payload of genetic information into target cells to correct malfunctioning cellular mechanisms (e.g. correct the production of defective proteins) or express therapeutic genes (e.g. gene-addition to produce missing proteins). The genetic payload can be formulated in one of many ways depending on the mechanism of action, including as plasmid DNAs, messenger RNAs (mRNAs), antisense oligonucleotides (ASOs), small interfering RNAs (siRNAs), microRNAs (miRNAs), or aptamers [Citation6–13]. This payload is typically incorporated within an appropriate platform or carrier to facilitate uptake into target cells, for which viral and non-viral vectors have been developed. Viral vectors take advantage of evolutionary adaptations for efficient entry into target cells, and various viral platforms largely based on adenoviruses, lentiviruses, and adeno-associated viruses have been described [Citation14–16]. Viral vectors can be very efficient at delivering gene payloads into target cells and have provided the backbone of some successful clinical gene therapies, but this is balanced somewhat by a number of drawbacks including the potential for viral-induced immunogenicity [Citation7]. As an alternative, non-viral vectors consisting of natural or synthetic materials (including synthetic and biological polymers, lipids, and lipid- or polymer-based nanoparticles and nanocarriers) have been proposed, the advantages and challenges of which are discussed in the literature [Citation7,Citation17–20]. It may also be possible to deliver a gene therapy ‘naked’ (sans vector) though modifications of the genetic payload are typically required to improve stability and improve uptake to target cells [Citation9,Citation10].
In the U.S.A., the product development framework for gene therapies follows that of traditional drugs. After identification of a candidate therapy through basic research, preclinical study(s) must be undertaken to demonstrate proof-of-concept, determine safety/toxicology, and support an Investigational New Drug (IND) application. If the IND is successful, Phase 1, 2, and 3 clinical trials can be undertaken to support a Biologics License Application (BLA; essentially equivalent to a New Drug Application but specific to therapies involving biologics). Details of each step of this development framework for gene therapies are complex and product-specific, and further complications arise when utilizing nonstandard delivery modalities such as inhalation. Inhalation delivery for gene therapies is a relatively niche field, as most gene therapies are designed with alternative delivery routes in mind (e.g. intravenous, subcutaneous, intravitreal, etc.) [Citation6,Citation21]. Some notable exceptions exist, such as inhalation gene therapies for cystic fibrosis (CF). Various attempts at inhalation delivery of gene therapies for CF have been made over the past three decades [Citation22–24], but these approaches have thus far not yielded a successful marketed therapy [Citation15] (one very recent candidate, MRT5005, failed to demonstrate efficacy in a phase I/II clinical trial [Citation25], and development was subsequently halted). The challenges encountered with CF, what is considered almost an ideal target for an inhaled gene therapy [Citation15], point toward the difficulty in developing a successful inhalation gene therapy candidate even when preclinical data is promising [Citation24].
In the present work, we focus on in vivo preclinical study of inhalation gene therapies. Preclinical data supporting safety and efficacy are of critical importance for gene therapies, and an essential parameter of interest to be defined in preclinical work is the dose of therapeutic material delivered to the animal model [Citation26]. However, traditional and standardized preclinical testing methods ‘which were developed for drug development and device testing, are often not appropriate for evaluating the safety of (cellular and gene therapy) products’ (FDA [Citation27]). In other words, it is often unclear what sampling methods or assays are appropriate when performing preclinical work with gene therapies, and what has worked in the past for small molecules may not be sufficient in convincing researchers, sponsors, and regulators that a product is safe, efficacious, and worth the risk in moving into clinical investigations in humans. This paper therefore aims to highlight the importance of fit-for-purpose testing for gene therapies in in vivo preclinical drug development while also identifying current challenges in the field.
We first review general characteristics of inhalation delivery of therapeutics, followed by a discussion of various elements and biological barriers that influence inhalation delivery of gene therapies in the respiratory tract. Important considerations of animal models are reviewed, as well as sampling methods and assays to determine dose for gene therapies in the preclinical setting. Examples of preclinical programs for inhalation gene therapies undertaken at Lovelace Biomedical Research Institute are then reviewed to catalog experiences and lessons learned during preclinical work in this evolving field of therapeutics. Following convention for Expert Opinion on Drug Delivery, we close with an opinion summarizing our thoughts on the future of the field. The focus of this review is on in vivo preclinical animal models for inhaled gene therapies – other elements of preclinical work, including in vitro cell and lung-on-a-chip models, are reviewed elsewhere [Citation28–30].
2. Inhalation delivery
There is a long history of treating disease with inhaled aerosols [Citation31]. Pharmaceutical aerosols including bronchodilators and anti-inflammatories are well recognized as useful tools for treating lung diseases such as asthma, chronic obstructive pulmonary disease (COPD), and CF. In these cases, pharmaceutical aerosols allow for the delivery of medication directly to the site of intended action, which helps to reduce off-target dosing and associated side-effects while providing a convenient and noninvasive administration method for the patient. Pharmaceutical aerosols can also provide a pathway for systemic treatments, as the gas-exchange region (i.e. the alveolar region) of the lung provides a large surface area and profuse blood flow that can facilitate rapid absorption into systemic circulation. The advantages for aerosol delivery of therapeutics are frequently summarized as (i) delivery directly to the site of action and reduced off-target dosing/systemic toxicity for treatments of lung disease, (ii) rapid uptake, and (iii) safety and convenience for the patient, as inhalation is a noninvasive delivery route [Citation22]. Such advantages make the prospect of inhaled gene therapies attractive for treating genetic disorders like CF [Citation23,Citation24] and alpha-1 antitrypsin deficiency [Citation32] as well as acquired disorders including asthma [Citation33] and certain cancers of the lung [Citation34].
Advantages of inhalation delivery must be weighed against a number of associated challenges. For one, dosing to the lungs can be highly variable depending on the specifics of the device – formulation combination, often demonstrating considerable inter-subject variability and large differences between different patient groups (e.g. children vs. adults, healthy vs. diseased, etc.) [Citation35]. Respiratory tract deposition depends on many factors including anatomy, disease state, aerosol properties (e.g. particle size, hygroscopicity), and patient use characteristics (inhalation profile, coordination with the delivery device, etc.) [Citation35], and subsequent disposition of a deposited aerosol particle is governed by myriad factors including material properties and the action of natural defense mechanisms in the airways [Citation36–38]. Further challenges arise in formulating the suspensions, solutions, or powders that make up the stock to be aerosolized at the time of delivery. Stability is of particular concern with gene therapy formulations, as the gene therapy payload (and vector, if present) must maintain integrity through manufacturing and aerosolization for it to function as desired upon reaching target tissues – approaches for design and manufacture of gene therapies and vectors lie outside the scope of the present work but are reviewed elsewhere [Citation7,Citation16,Citation39–41].
2.1. Aerosol characteristics affecting inhalation drug delivery
A pharmaceutical aerosol is a gas-borne suspension of a therapeutic agent (or agents) distributed in solid particles or liquid droplets. One of the most important parameters influencing the behavior of a pharmaceutical aerosol is the aerodynamic diameter, , of the solid particles or liquid droplets. This aerodynamic diameter greatly influences the physical behavior of an aerosol and the relative importance of various deposition mechanisms in the respiratory tract, the most important of which are typically inertial impaction, gravitational settling, and Brownian motion [Citation42]. For pharmaceutical aerosol delivery to the human lung, it is generally thought that aerosols with a mass aerodynamic diameter between 1 and 5 µm are optimal, as this size provides a reasonable balance between (a) being small enough to mostly transit the extrathoracic airways and reach the lungs and (b) being large enough to deposit in the respiratory tract before being exhaled. Regional deposition within the lung, often characterized in vivo as central vs. peripheral deposition (roughly corresponding to the conducting airways vs. alveolar region) is also influenced by particle size, with a tendency for smaller particles to show deeper penetration into peripheral airspaces (though exceptions exist considering the myriad factors influencing deposition). As the physiology and cellular make-up of the respiratory tract changes as one transitions from the conducting to peripheral airways, the location of deposition can lead to important differences in disposition [Citation38]. Respiratory tract deposition in humans is well characterized, with various deposition models being presented in the literature [Citation43–46].
2.2. Barriers to inhalation delivery of gene therapies
Numerous physical and biological barriers exist that can complicate gene therapy via inhaled aerosols. Clinical trials for candidate cystic fibrosis gene therapies exemplify the difficulties – many promising candidates have failed to show clinical benefit due largely to inefficient gene transfer to target cells [Citation15,Citation47,Citation48]. Proper considerations of the various elements that can interfere with a gene therapy are therefore critical. Here, we focus on challenges arising from (i) aerosolization, (ii) respiratory tract anatomy and physiology, and (iii) processes influencing disposition (e.g. uptake) of deposited material in the respiratory tract. Other important ‘barriers’ for gene therapies, including the potential for immunogenicity, insertional mutagenesis, and off-target effects, are discussed elsewhere [Citation7,Citation14,Citation47].
2.2.1. Aerosolization
Common delivery devices for therapeutic aerosols include nebulizers, dry powder inhalers, and pressurized metered dose inhalers [Citation49]. In exploratory research, nebulizers are perhaps the most accessible delivery device as they allow for the direct aerosolization of an aqueous formulation containing the therapeutic agent [Citation50] – generally speaking, if an aqueous solution or suspension can be formulated with the therapeutic agent, the agent can be nebulized to create an inhalable aerosol. This simplicity contrasts with the non-trivial formulation processes that are required with dry powder inhalers (e.g. spray drying, spray freeze drying, micronization, etc.) [Citation51,Citation52] and pressurized metered dose inhalers (stability in propellants) [Citation53,Citation54], where the successful application of such processes requires special consideration of the somewhat fragile nature of genetic material relative to more traditional non-biological therapeutics – see discussions of challenges and approaches by Gomes dos Reis et al. [Citation41]. Nebulizers, then, are a reasonable starting point for aerosolization of gene therapies in the preclinical setting.
There are a few categories of nebulizers that differ in operating principles. Compressed jet nebulizers use a high-pressure steam of air to disrupt a stream of liquid formulation, leading to primary droplet generation as this liquid stream breaks apart. Typically, these primary droplets are too large to be inhaled, so jet nebulizers are often designed with internal baffles that help to break primary droplets into smaller secondary droplets via impaction. Only a small number of these secondary droplets are emitted from the nebulizer in a given nebulization ‘cycle,’ with considerable recycling of liquid back into the reservoir – formulation components are subjected to multiple nebulization cycles before exiting the device [Citation55]. Vibrating mesh nebulizers use rapid oscillations of a perforated mesh to extrude liquid and generate droplets directly. Impinging jet nebulizers generate droplets by colliding two streams of liquid jets, creating an unstable liquid sheet that subsequently fractures into droplets. Ultrasonic nebulizers use acoustic vibrations to generate surface waves at the air-liquid interface of a formulation, leading to droplet generation through wave amplification and breakup. The underlying fluid-mechanical processes that influence each category are described in the literature [Citation42]. Of the above, compressed jet nebulizers and vibrating mesh nebulizers are the most commonly used in preclinical research and clinical treatments – representative schematics of these categories are shown in .
Figure 1. Schematics of (a) a classic unvented jet nebulizer, often the basis for disposable nebulizers (e.g. Hudson RCI® micro Mist® and Hudson RCI® up-draft II® opti-Neb®; Teleflex Medical, U.S.A.), (b) a vented jet nebulizer wherein all inhaled air passes through the droplet production region (e.g. AeroEclipse II BAN; Trudell Medical, Canada, and PARI LC® Plus; PARI respiratory equipment, Inc, U.S.A.), and (c) a mesh nebulizer, wherein thousands of perforations in a rapidly vibrating diaphragm act to aerosolize droplets (e.g. Aerogen® Solo; Aerogen Ltd., Ireland, and PARI eFlow®; PARI Pharma GmbH, Germany). Schematics are reproduced from Finlay [Citation42] with permission of the publisher, Elsevier.
![Figure 1. Schematics of (a) a classic unvented jet nebulizer, often the basis for disposable nebulizers (e.g. Hudson RCI® micro Mist® and Hudson RCI® up-draft II® opti-Neb®; Teleflex Medical, U.S.A.), (b) a vented jet nebulizer wherein all inhaled air passes through the droplet production region (e.g. AeroEclipse II BAN; Trudell Medical, Canada, and PARI LC® Plus; PARI respiratory equipment, Inc, U.S.A.), and (c) a mesh nebulizer, wherein thousands of perforations in a rapidly vibrating diaphragm act to aerosolize droplets (e.g. Aerogen® Solo; Aerogen Ltd., Ireland, and PARI eFlow®; PARI Pharma GmbH, Germany). Schematics are reproduced from Finlay [Citation42] with permission of the publisher, Elsevier.](/cms/asset/c0f09b4f-3d8a-4314-b72d-625c049df2e0/iedd_a_2261369_f0001_b.gif)
Fundamentally, each category of nebulizer uses some approach to generate stresses in a liquid formulation to overcome surface tension at an air liquid interface, leading to the generation of droplets with a vast increase in surface free energy (associated with the orders of magnitude increase in surface area associated with aerosol droplets as opposed to bulk solution). As such, formulations undergoing nebulization are subject to stresses that can influence or degrade formulation components, and the magnitude of these stresses can vary with the design of the nebulizer [Citation56].
Historically, nebulization-induced degradation has been a major concern with gene therapies. Initial forays into the use of nebulizers with some gene therapy products suggested that considerable degradation of genetic material could be caused by the nebulization process itself [Citation56–61]. Deshpande et al. [Citation57] found significant degradation of naked plasmid DNA after nebulization with the AERx Pulmonary Delivery System (quantified via agarose gel electrophoresis on samples recovered from a cascade impactor) and then demonstrated that a change of formulation to incorporate cationic lipoplexes maintained DNA integrity. Arulmuthu et al. [Citation58] observed severe degradation of 20 kb naked plasmid DNA after nebulization with the MicroAIR NE-U22 vibrating mesh nebulizer using a combination of atomic force microscopy, agarose gel electrophoresis, and PicoGreen assay on aerosol condensate samples, but also observed that smaller 5.7 kb plasmids maintained stability. Arulmuthu further showed that complexation of the 20 kb plasmid with polyethylenimine (PEI) allowed for successful aerosolization without damage to the DNA. Gomes dos Reis et al. [Citation59] recently used a similar assay approach to investigate the roles of polyplexes and excipients in aerosol delivery of plasmid DNA in in vitro cell models, finding a small but acceptable degree of loss after nebulization with a partial optimization of formulation. Lentz et al. [Citation60] observed degradation of 5 kb and 9.8 kb plasmids in jet nebulizers, ultimately concluding that hydrodynamic shear was the mechanism responsible for degradation, and further showed that complexation of DNA with cationic agents could improve stability during aerosolization. A later study by the same group evaluated naked plasmid and cosmid DNA stability through aerosolization with multiple classes of nebulizers and found that the degree of degradation of non-complexed DNA occurring during aerosolization varied considerably between different devices and for different sizes of DNA [Citation56]. Catanese et al. [Citation61] investigated the stability of various DNA vectors ranging from 0.3 kb to 5.3 kb over time in the reservoir of an Aerotech II jet nebulizer, finding an almost threshold-like relation between DNA length and survivability – vectors smaller than 2 kb were relatively stable, while vectors longer than 3 kb showed considerable degradation in a timespan of minutes. One limitation of this study by Catanese et al. [Citation61] was the use of aliquots from the nebulizer reservoir to evaluate degradation rather than samples of the aerosol generated by the nebulizer – results of this study may not be fully representative of DNA stability post-nebulization.
Lengsfeld and Anchordoquy [Citation62] reviewed mechanisms of plasmid DNA degradation. Fundamentally, models of shear-induced degradation conclude that the tensional force imparted by a shear field will increase with the molecular weight of the plasmid, and increasing tensional force increases the likelihood of degradation (specifics vary depending on assumed mechanical behavior of DNA strands). A simple model, wherein a strand of DNA is treated as a rod, yields the following relation for the maximum force exerted on the strand based on the frictional force per unit length
, the length of the strand
, the orientation of the strand relative to streamlines
, and the strain rate
:
For a given flow field, this relation suggests that the force imposed on a strand of DNA increases with the square of its length – larger DNA molecules are increasingly susceptible to fragmentation, as indeed is observed in the literature [Citation61,Citation62]. The fragmentation process for plasmid DNAs, which in reality have much more complicated structures than a rod, is thought to include first a single-strand break that leads to a conformational change from the native supercoiled form into an open circle, then double-strand breaks causing transitions from open circles to linear structures and from linear structures into smaller and smaller fragments [Citation56]. In practice, EquationEquation 1(1)
(1) is not easily applied to forces generated during nebulization [Citation60]. Arulmuthu et al. [Citation58] describe an alteration of this model that allows for an estimation of forces experienced by DNA in a given flow field based on local strain rates,
where is the fluid viscosity,
is the elongational strain rate (determined by local velocity gradients), and
is a dimensionless constant whose value depends on the concentration of DNA and the intrinsic viscosity. This model is also complicated to apply to DNA considering unknown and shifting configurations, but it can be used to provide estimates of the maximum possible forces experienced by DNA (based on length scales measured through, e.g. atomic force microscopy, or theoretical supercoiled lengths) that seem to broadly agree with experimental data [Citation58].
Regardless of specifics, the observed relation between increasing DNA size and greater tendency for fragmentation leads to approaches that ‘condense’ plasmid DNA via, e.g. complexation with a cationic agent, thereby reducing the effective length scale (the hydrodynamic diameter) of the plasmid and resulting in reduced hydrodynamic stresses in a given flow field [Citation61,Citation62]. Arulmuthu et al. found that complexation of a 20 kb plasmid with PEI decreased its characteristic molecular dimension from 1250 nm to 400 nm, and as noted above this allowed for successful aerosolization via mesh nebulizer [Citation58]. Other studies have demonstrated the feasibility of this complexation approach for aerosolization of long plasmid DNA (see above), with additional works in this area reviewed by Gomes dos Reis et al. [Citation41]. One potential drawback of complexation with cationic agents for increased stability, as noted by Lentz et al., is that transcription in the nucleus requires removal of the cationic agent [Citation63], and if the DNA complex is too stable, it may not dissociate as required in the intracellular environment for effective gene expression [Citation56]. Importantly, transfection of naked plasmid DNA is regarded as challenging due to size- and electrical charge-related inefficiencies of naked DNAs in entering cell membranes and nuclear membranes [Citation17,Citation64], as well as degradation via nucleases within the cell during translocation to the nucleus [Citation65]. Some works that have evaluated DNA transfection in preclinical models have observed lower gene expression for naked plasmid DNA when compared to DNA-complexes or use of nonviral vectors [Citation66,Citation67]. Broadly speaking, transfection with naked plasmid DNA, while not impossible, is considered inefficient relative to more targeted approaches. Other nucleic acids like siRNA and mRNA are also of interest for gene therapies. Considering differences in mechanical properties of RNAs [Citation68] and DNAs [Citation69], a direct extension of the above framework (i.e. the suggestion that the generally smaller size of many RNAs as compared to DNAs necessarily leads to improved stability during aerosolization) is ill-advised absent experimental validation. Some have postulated that vectors may be required for protection of RNA from shear-induced degradation during nebulization and to improve stability in the liquid stock [Citation39], though successful aerosolization of naked siRNA with multiple nebulizer types in vitro [Citation70,Citation71] suggests this is not always the case. Though no published in-depth characterizations of RNA stability through nebulization have been performed [Citation13], we suspect a general trend of longer RNAs being more sensitive to degradation during aerosolization – this a topic for future work. Chow et al. have noted that while naked RNA can be transfected in the lung following pulmonary delivery, the exact mechanisms by which this occurs are not clear, and delivery vectors may significantly improve RNA transfection compared to naked RNA in the airways [Citation13].
It is also important to consider the effects of aerosolization stresses on viral and non-viral vectors that are often used as carriers for gene therapy payloads. Viral vectors can denature and unfold, aggregate, and precipitate under shear stress and exposure to air-liquid interfaces (see discussion by Srivastava et al. [Citation16]), leading to inhibition that may prevent effective uptake into target cells. We are not aware of published data that has directly evaluated the effect of nebulization on viral vectors for gene therapy in a manner allowing for biologically relevant characterization of losses akin to what has been performed for plasmid DNA (e.g. how much virus is inactivated during aerosolization, and how can these losses be mitigated?). Studies in other areas (e.g. viral vectors for vaccination [Citation72,Citation73] and inhalation delivery of bacteriophages [Citation55]) may provide a template for appropriate methods. Non-viral vectors can also degrade under aerosolization stresses [Citation17], causing damage to or fracturing of e.g. lipid nanoparticles [Citation74,Citation75] that in the context of gene therapies equates to a premature release of genetic material before entering target cells.
Ultimately, the diverse approaches for gene therapy payloads and viral/non-viral vectors complicate a priori determinations of suitable aerosolization techniques. An ideal aerosolization technique is one that (a) causes minimal degradation of formulation components, as this maximizes the amount of agent that will function as intended upon delivery, and (b) can be used effectively by the patient while maximizing the dose delivered to target tissues. The second factor is complicated in preclinical research in animal models for reasons that will be noted in Section 3. The first factor is perhaps best characterized in vitro through fit-for-purpose testing (as will be discussed in Section 4), though there is also potential to use a combination of analytical and computational techniques to estimate the magnitudes of stresses generated with different aerosolization techniques [Citation58,Citation60]. However, application of analytical and numerical approaches toward this topic is not trivial, and such approaches benefit from case-by-case experimental validations [Citation55].
2.2.2. Respiratory tract anatomy and physiology
The respiratory tract is typically categorized into three regions based on structure and function [Citation38,Citation42,Citation43,Citation76,Citation77]: (i) the extrathoracic region, consisting of the nose, mouth, and throat, (ii) the conducting region, containing the tracheobronchial airways from the trachea to the terminal bronchioles, and (iii) the gas-exchange region, containing the alveolated airspaces from the respiratory bronchioles to individual alveoli. The thoracic airways, containing the conducting and gas-exchange regions, can be thought of as a branching tree-like structure containing some two dozen generations of airways. Factors influencing aerosol behavior and deposition in the respiratory tract have been well-characterized [Citation42] such that only a brief review is outlined here.
The extrathoracic airways (either oropharyngeal or nasopharyngeal) act as a partial filter of inhaled aerosols, while also heating and humidifying inhaled air. Deposition in the nasal airways is greater than that occurring in the oral airways, so oral inhalation is the preferred route for pulmonary drug delivery in humans. Significant inter- and intra-subject variabilities are observed in extrathoracic deposition with many pharmaceutical aerosols that directly cause large differences in total lung doses [Citation78,Citation79]. Extrathoracic deposition can be a major hurdle to efficient lung delivery – in vivo imaging studies have shown average losses of anywhere from 20 to 80% (and even beyond) of inhaled pharmaceutical aerosol to deposition in the mouth-throat, depending on device, formulation, and patient population [Citation80–82]. The understanding of factors influencing extrathoracic deposition in humans has improved considerably over the past few decades [Citation83], and there is promising research into new formulations and devices that may largely mitigate extrathoracic deposition to improve delivery to the lungs [Citation84–86].
The conducting airways begin at the trachea and extend to the terminal bronchioles. They consist of approximately 15 generations of sequentially branching, narrowing, and shortening tubular airways, with the terminal bronchioles having diameters of ~0.5 to 1 mm. The conducting airways have some rigidity provided by smooth muscle and cartilage, as well as a ciliated epithelium that is covered by a relatively thick (~10 µm) lining of aqueous airway fluid (periciliary sol) and gel-like mucous. Hotspots of deposition can occur at the bifurcations between sequential airway generations due to impaction [Citation87,Citation88]. Mathematical deposition modeling based on in vitro data with typical pharmaceutical aerosols suggests that deposition in the conducting airways can range anywhere from 5 to 50% of the dose reaching the lungs (i.e. not lost to extrathoracic deposition) [Citation46,Citation89–91] for orally inhaled pharmaceutical aerosols, depending on multiple factors including breathing pattern and aerosol properties.
The gas-exchange region begins at the respiratory bronchioles, extends through alveolar ducts and alveolar sacs, and ends at individual alveoli. Functional units in this region, consisting of all daughter generations of a single terminal bronchiolus, are coined acinus. These peripheral airspaces expand and contract considerably during respiration, with a functional residual capacity of ~3 L and a total lung capacity of ~6 L in adults [Citation42]. Human adults have somewhere between 300 and 600 million alveoli, each with a diameter on the order of 250 µm when expanded, providing a massive surface area to facilitate efficient respiration [Citation92]. Both the size and number of alveoli increase during development from birth to adulthood, but in different ways: the number of alveoli multiplies considerably during the first 2 years after birth, then increase in size in a proportionate manner with the airways [Citation92]. Compared to the conducting airways, the airway surface liquid forms a much thinner (~0.1 µm) layer of pulmonary surfactant, and deposited material is removed partly through actions of alveolar macrophages [Citation38].
For inhaled therapies deposition is ideally targeted to the site of intended action as directly as possible, as off-target dosing represents wasted therapeutic agent and may be associated with deleterious side effects. With gene therapies, target cells may be present throughout the entire lung or distributed in higher amounts in specific regions depending on the disease. For example, CF is caused by mutations in the gene encoding CF transmembrane conductance regulator (CFTR) proteins. CF manifests in various organ systems but is of particular concern for its effects in the lungs, where dehydration of the airway surface layer impedes mucociliary clearance, obstructs the airways, and permits establishment of pathogenic infection [Citation93]. The various types of cells lining the human airways express different levels of CFTR, with a general rank order of CFTR expression in human superficial airway epithelium being secretory cells, basal cells, ionocytes, and ciliated cells, respectively [Citation23]. While a successful gene therapy for CF may need to restore CFTR function in multiple cell types, a major target appears to be the airway secretory cells (goblet cells and club cells) that are located in the tracheobronchial region [Citation23]. One can hypothesize that targeted delivery to the tracheobronchial airways (i.e. maximizing the deposited dose in this region [Citation94]) could then be of benefit for gene therapies of CF, but direct evidence for targeted regional deposition here will be difficult to obtain.
2.2.3. Disposition
Following deposition, an inhaled gene therapy must penetrate natural defense mechanisms to enter target cells. The nature of these mechanisms changes with depth into the lungs, as illustrated in , and depend on local histology and physiology.
Figure 2. Representations of (top) histology and structure and (bottom) clearance mechanisms acting on deposited material in the human respiratory tract. In the bottom figure, numbers indicate generalized disposition processes: (1) initial deposition, (2) crossing of the pulmonary epithelium, and (3) clearance. Figures reproduced from Hastedt et al. [Citation38] under open access license agreement, courtesy of the publisher, Springer.
![Figure 2. Representations of (top) histology and structure and (bottom) clearance mechanisms acting on deposited material in the human respiratory tract. In the bottom figure, numbers indicate generalized disposition processes: (1) initial deposition, (2) crossing of the pulmonary epithelium, and (3) clearance. Figures reproduced from Hastedt et al. [Citation38] under open access license agreement, courtesy of the publisher, Springer.](/cms/asset/62a760f8-6316-491c-b602-d25fd1c6bb1c/iedd_a_2261369_f0002_oc.jpg)
A major barrier in the conducting airways is mucociliary clearance and the mucous layer itself [Citation15]. Schürch et al. [Citation95] showed that particles depositing on top of the mucous layer may naturally be drawn into the layer itself through surface tension forces. In the conducting airways, deposited material drawn into the mucus layer can penetrate into the underlying periciliary sol [Citation96], coming into close contact with cilia and a diverse and active immunological defense system [Citation97]. Penetration through this mucous layer, which can be influenced by disease (consider the thickening and increased viscosity of the mucous layer that occurs with CF and chronic obstructive pulmonary disease [Citation98]), is a necessary condition for gene therapies targeting airway epithelial cells, and can be promoted through various strategies including the use of mucolytic agents or muco-penetrating nanoparticles [Citation39,Citation96]. In the alveolar region, deposited material appears to become completely wetted with a surfactant film [Citation95], and a similarly efficient innate defense system, in particular alveolar macrophages, acts to protect the lung again foreign matter [Citation99]. Interactions of surfactant with deposited material (e.g. formation of ‘protein coronas’ on nanoparticles) can further influence disposition [Citation39].
Following the above-mentioned extracellular barriers, the next obstacle for a gene therapy is the airway epithelium itself. Tight junctional complexes between adjacent cells regulate transfer of material between the apical and basolateral layers of the airway epithelium. A gene therapy must enter the cells of the epithelium to deliver the genetic payload, and many commonly used viral vectors target receptors that are present only on the basolateral surface, below the tight junction. In such cases, the tight junction prevents easy access to target receptors and causes reduced transduction efficiency [Citation15]. Strategies to improve uptake into target cells include passive and active targeting [Citation7]. Passive targeting of e.g. lipid nanoparticles has been proposed to occur due to coating of nanoparticle surfaces with endogenous biomolecules, altering the interactions of the nanoparticle with the immune system and target tissues [Citation7]. Such passive effects may be responsible for successful transduction of naked RNA in lung tissue that has been observed in some studies, though the exact mechanism for this process remains unclear [Citation13]. Active targeting may be achieved through incorporation of receptor-specific ligands, antibodies, or peptides to a gene therapy platform [Citation7,Citation41,Citation100–102].
3. Animal models
Most inhaled pharmaceutical aerosol delivery devices are designed specifically for oral inhalation in adult human patients. Animals are obviously not capable of using these devices as intended, so some liberties must be taken in modifying the administration method to accommodate aerosol delivery to animals. The animal model itself also influences the selection of an appropriate inhalation exposure system, with nose-only or whole-body exposure chambers often used with rodent species, and head-domes or facemasks used with larger mammalian species. Inhalation exposure systems for various animal models are well described in the literature [Citation26,Citation103–105], having been used for decades in studies of inhalation toxicology and pharmaceutical drug development. As such, the discussion here is limited to a few salient points relevant for gene therapy products.
3.1. Devices, delivery efficiency, and pulmonary dose
In Section 2.2.1, it was noted that nebulizers are a typical starting point for preclinical evaluations of gene therapy products, as nebulization requires fewer formulation steps compared to aerosol generation via e.g. dry powder inhalers. Nebulizers carry an additional advantage for preclinical work in that the same device that is used in human patients can be used directly to generate aerosols for many inhalation exposure systems, unlike powders where aerosolization for preclinical study is typically performed with equipment like rotating brush generators. Preclinical and clinical study with the same nebulizer–formulation combination allows for clinically relevant quantification of viability following nebulization, a major factor of concern for gene therapies.
Experimentalists must recognize that delivery efficiency, or the amount of drug that is available for inhalation by the animal (the delivered dose) relative to what loaded into the aerosol generation device, is typically very low for standard preclinical exposure systems. This is a consequence of various factors, including deposition losses on inner surfaces of system components and the necessity of maintaining adequate airflow to satisfy respiratory minute volumes (RMVs) of all animals on a system [Citation26]. This low delivery efficiency is compounded by differences in respiratory tract deposition between different animal species and humans. Extrathoracic deposition in obligate nose breathing animal species is typically considerably greater than that occurring for oral delivery in humans, and the corresponding dose reaching the lungs is much lower. Current practice for predicting pulmonary dose in an animal model is to apply a species-specific ‘deposition factor’ to the delivered dose [Citation26]. The pulmonary dose (, expressed in [mg/kg]) is then calculated as
where is the concentration of drug in the inhaled air in [mg/L], RMV is the respiratory minute volume in [L/min] which can be measured using e.g. plethysmography or estimated through allometric relations to the body weight [Citation26,Citation106],
is the duration of exposure in [min],
is the deposition factor [unitless], and
is the body weight in [kg]. Standard deposition factors and body weights for select animal models are provided in . Reported deposition fractions should be considered as approximations for qualitative scaling of pulmonary dose estimates between different species, rather than strict quantitative values.
Table 1. Standard values of various parameters used for predictions of pulmonary dose in animal models, based on Tepper et al. [Citation26] unless otherwise stated.
For gene therapies, it is important to quantify concentration in a biologically relevant manner (see discussion in Section 4) – gravimetric measurements alone, for example, will not provide information on the proportion of a therapeutic that may be inactivated or denatured in a test system. Dose for a gene therapy may be better expressed in units such as DNA or RNA copies, viral copies, or plaque forming units, rather than in milligrams of test article, with the units of concentration in EquationEquation 2(2)
(2) adjusted appropriately. In addition, while body weight is a typical normalization factor used to calculate dose in an animal-specific manner, alternative normalization factors – including lung weight, surface areas corresponding to the body, the conducting airways, the pulmonary airspaces, or the nares, and airway surface liquid volume – may be more relevant for certain therapies. This is particularly important when a measure of ‘local dose’ is desired for quantifying toxicity in certain tissues (e.g. dose depositing in the nares during nose-only inhalations is better defined via the nasal surface area than via body or lung weight). The determination of appropriate expressions for dose is a program-specific process, and multiple quantifications of dose may be required to evaluate safety and toxicity.
The amount of test article required to achieve a specific dose with an inhalation exposure system is greater than the amount required for other routes of administration (often by a factor of 10 or more) [Citation26]. The low efficiency is a known problem that has been demonstrated in the literature: Nadithe et al. [Citation108], for example, showed that the average dose obtained in mice in nose-only exposure chambers was 0.108% of that added to the nebulizer, with only 0.0087% depositing in the lungs (equal to 8.19% of the dose presented to the mouse). The general requirement for large amounts of test article relative to other routes of exposure is a largely unsolved problem with in vivo preclinical inhalation studies, particularly in the context of gene therapies for which manufacturing is not straightforward.
3.2. Translatability
Animal species used in preclinical study of a gene therapy should ‘demonstrate a biological response to the investigational (cellular and gene therapy) product similar to that expected in humans in order to generate data to guide clinical trial design’ (FDA [Citation27]). This can be a challenge, as many targets for gene therapy may not have analogous genotypes or phenotypes in traditional animal models. In some cases, including with CF [Citation109], disease-specific animal models can be developed to better emulate human disease in preclinical settings. CF ferret models have seen increased attention in recent work [Citation110,Citation111], and we include ferret-specific estimates of parameters useful for translatability of preclinical data in to address a lack of such data in the literature [Citation26].
A non-exhaustive list of published animal models employed in studies on pulmonary delivery of gene therapies includes mice [Citation75,Citation112–118], rats [Citation119–121], rabbits [Citation122], pigs [Citation123–125], non-human primates, sheep [Citation126], and horses [Citation127]. Many of these models have utilized intratracheal administration techniques including instillation (via pipette) or sprays (via e.g. Microsprayer, from Penn Century) [Citation28]. Neither technique is considered an equivalent route of exposure as inhalation owing to considerable differences in pulmonary deposition and disposition that occur when a material is administered directly to the lungs as a liquid (via instillation) or as a spray of large droplets [Citation28,Citation128–130]. Some data suggests that such intratracheal bolus delivery systems may require administration of higher doses relative to inhalation to achieve similar pulmonary gene expression with certain therapies – preliminary work by Bush et al. [Citation131] in a CF rat model found that nose-only inhalation of a novel RNA-based epithelium sodium channel (ENaC) inhibitor required one-tenth of the dose delivered via intratracheal administration to achieve equivalent knockdown in whole-lung expression of αENaC, perhaps due to high heterogeneity of pulmonary deposition obtained with bolus delivery. Intratracheal bolus administration methods are often used in early proof-of-concept studies, but the inherent differences to inhalation administration should be borne in mind. The translation and interpretation of results in such preclinical programs of gene therapies is not straightforward – good discussion of the challenges is presented in the literature [Citation7,Citation21,Citation30,Citation132]. Cheever and colleagues stress the importance of fit-for-purpose study designs: ‘in vivo models should be used to test specific hypothesis or answer specific questions that the model can provide information on’ (Cheever et al. [Citation132]). Further elements of animal models, including in-depth analyses of biodistribution via pathology, allow for a characterization of off-target dosing effects that cannot be replicated in human trials. However, biodistribution must be carefully considered with regard to tropism. As reviewed by Ramamurthy et al. [Citation30], vector tropism is governed by serotype-specific interactions that may differ considerably between different species. Thus, while preclinical study remains critical for evaluating gene therapy safety, great care must be taken in interpreting results [Citation132]. Shedding data may also help evaluate risks during preclinical programs, particularly for novel therapies that have not been used in humans, but for similar reasons as above shedding in animal models is not a substitute for study of shedding in humans [Citation133]. Ultimately, there remains a need for improved understanding and characterization of translatability of non-clinical data for gene therapy products, particularly in newly developed animal models in which inhaled aerosol deposition and disposition remains to be evaluated.
4. Analytical methods
Traditional aerosol therapeutics benefit from well-established analytical methods for sampling and assaying that are described in e.g. the United States Pharmacopeia (USP). Gene therapy aerosols, on the other hand, frequently require fit for purpose development for collection and analysis. Analytical methods in inhalation exposures for preclinical research aim to accurately characterize the concentration of therapeutic agent available at the breathing zone of the animal model (parameter in EquationEquation 2
(2)
(2) ). EquationEquation 2
(2)
(2) then allows for an estimation of the dose delivered to the model as described previously. As every gene therapy program is unique, details below are provided for what has been used in the past and what has been adapted by experts in the field to support the characterization of gene therapy aerosols.
4.1. Sample collection
Non-clinical aerosol delivery systems are typically characterized for two primary end points: aerosol concentration and particle size distribution. The methods to collect standard aerosols have been well described [Citation104]. For aerosol concentration, filter media are typically used to collect a fraction of the aerosol from the exposure system at the breathing zone of the animal model. Filters can then be analyzed by nonspecific means (i.e. differential weight analysis) or specific means (e.g. extraction with an appropriate solvent, followed by high-performance liquid chromatography for particular compounds) – such methods are similar to those used in chemistry and manufacturing controls (CMC) testing as described in pharmacopoeia [Citation134,Citation135]. An ideal filter will capture 100% of particles during sampling, facilitate recovery of material for chemical/biological assay, and avoid generation of large pressure drops for sample durations of a reasonable length (on the order of minutes). In reality, there is a balance between these factors that varies for filter media of different materials (e.g. fiber filters made of borosilicate glass, quartz, cellulose, or other materials; membrane filters made of polyethersulfone (PES), polytetrafluoroethylene (PTFE), Teflon, or other materials). Occasionally, liquid collection systems (e.g. glass frit impingers, biosamplers, etc.) are used for collection of biologically active aerosols including bacteria, viruses, and spores, as collection directly in liquid media avoids desiccation of biological material and associated loss of viability that can occur with filters. Reduced desiccation via collection in liquid media should, however, be considered against the introduction of additional shear forces through e.g. impinging jet effects, turbulent motion in collection media, or prolonged and repeated exposure to dynamic air–water interfaces (‘bubbling’) – such effects may be evaluated through spike recovery tests. In additional settings, the utilization of condensate may be required for some analytical end points. Methods similar to those previously published by Cipolla et al. can be adapted to the non-clinical delivery systems to ensure that the analysis does not impose an artifact on the analysis [Citation136].
For particle size distributions, cascade impactor measurements are the standard from a regulatory perspective, being well described in the literature [Citation104] and facilitating regular performance verification and validation. Standard practice for cascade impactor measurements of nebulized aerosols is specified in pharmacopoeia [Citation135,Citation137]. These methods concern measurements of droplet size at the exit of the mouthpiece and recommend the use of the Next Generation Impactor at a constant flowrate of 15 L/min, cooled to approximately 5°C to reduce artificial biasing of measured droplet sizes via evaporation during transit through the impactor itself. Appropriate methods for cascade impactor sizing of nebulized aerosols in preclinical exposure systems may vary, depending on appropriate flowrates and the nature of the aerosol at the breathing zone of the animal. Other particle sizing techniques (time-of-flight, light scattering, etc.) are frequently not designed nor capable of meeting regulatory needs for non-clinical studies, relegating their use to supplemental rather than primary analysis. A typical target for particle sizes in preclinical work is an MMAD between 1 and 5 µm [Citation26] – a somewhat forgiving range of sizes that is relatively easy to obtain at the breathing zone of exposure systems using modern nebulizers (though the particle size range for a program should be and is refined to a range that matches the needs of the program). Most of the challenge instead lies in defining and measuring the aerosol concentration in a biologically relevant manner.
4.2. Sample analysis
Within any aerosol delivery program, the characterization of aerosol concentration is a critical end point, however, specific to biological aerosol delivery (including gene therapy) the aerosol must also be considered as both the total amount of material and the activity of the material. When the gene therapy is delivered within a vector (viral, non-viral or other) the aerosol should also be characterized for encapsulation or integrity post aerosol generation to evaluate whether the technique used for aerosolization results in unacceptably high losses.
Genetic material can be quantified in various manners, with common approaches being UV methods (HPLC/UPLC), polymerase chain reaction (PCR; variations of which include real-time quantitative PCR and droplet digital PCR), fluorescence assays (e.g. PicoGreen and RiboGreen), and gel electrophoresis. The selection of the most appropriate method is influenced by the range required, specificity required and often the equipment facilities have access to.
For viral vectors, virus quantification methods (e.g. TCID50, plaque assay) can quantify functional virus, with complementary PCR methods providing estimates of the total copies of viral genomes present in a sample. The ratio of these quantities can be used to evaluate influences of e.g. aerosolization on vector activity.
With nonviral vectors, a determination of encapsulation efficiency should be performed to evaluate integrity of carrier particles and premature release of genetic payload. For LNP-mRNA therapies, fluorescence-based assays can be used in conjunction with surfactants (e.g. Triton-X100) to evaluate encapsulation efficiency by comparing RNA content (or an appropriate tracer) in surfactant-treated and untreated samples [Citation74,Citation138]. In this approach, it is assumed that RNA content measured in untreated samples represents free (unencapsulated) RNA, while the RNA content in surfactant-treated samples represents the total (unencapsulated and encapsulated) RNA.
4.3. Example inhalation programs for gene therapy drug development
In this section, we highlight high-level details of a select preclinical programs performed at Lovelace Biomedical to support inhaled gene therapies that have yet to be fully published in the literature. Given the largely proprietary nature of much work in this evolving field, we are limited in how fully we can discuss each program. We aim here to identify techniques that have shown promise in characterizing preclinical inhalation delivery of gene therapies to encourage continued developments and open discussion on best-practices. Additionally, these details highlight the importance of ensuring that each system has the appropriate aerosol generation, aerosol characterization and quantitative methods that are based on method development data.
4.3.1. Mouse model with a novel viral vector
A study in a mouse model of inhalation delivery of a replication-deficient HSV-1 vector with a payload targeting CFTR deficiency was performed to evaluate safety. For inhalation exposures, test article was aerosolized using an Aerogen Solo vibrating mesh nebulizer into a nose-only exposure chamber. Two types of filters were used to sample aerosols from the breathing zone of the exposure system, (a) glass fiber and (b) PES membrane. Glass fiber filters provided measures of total aerosol concentration via gravimetry but were unsuited for further assay of the test article owing to poor extraction. PES membrane filters yielded satisfactory recovery of test article for further characterization of viability via qPCR and viral titer plaque assay. The combination of two filters allowed the simple (low resistance) glass fiber filter to provide rapid total aerosol concentration and the PES to provide the API concentration and activity analysis. This combination allowed the quantitative determination that there was a ~ 30% loss of viability through aerosolization, transit in the exposure system, and recovery from the PES membrane filters. These methods were fit for purpose for validation and applied to both non-GLP and GLP studies.
4.3.2. Ferret model with an AAV-vector
A study was undertaken in ferrets to evaluate pharmacology and safety of a novel AAV-based vector with a payload targeting CFTR expression via nose-only inhalation. For the AAV-vector, Aerogen Solo vibrating mesh nebulizers were used to generate test article aerosols into standard non-clinical nose only inhalation exposure systems. During method development, the effect of this nebulization process on the vector (i.e. vector titer, integrity, aggregation and infectivity) was quantified by nebulizing aerosol directly into 15 mL Falcon-style tubes cooled by submersion in ice – resulting liquid samples (often referred to as condensate, though strictly speaking condensate implies the sample has condensed from a vapor to a liquid – a different physical process than the deposition of aerosol particles or droplets against the inner walls of a cooled collection tube) were characterized via a cell-based potency assay for viability, ddPCR for genome titer, and size-exclusion chromatography for aggregation by independent third-party laboratories. Other methods for directly capturing post-nebulization samples, including copper and glass condensers and filter collections, showed insufficient recovery with the analytical method. On-study, aerosol mass concentration was measured using glass fiber filters, while genome titers of AAV in the aerosol were characterized via ddPCR on glass-frit impinger samples, captured from the breathing zone as shown in .
Figure 3. Schematic detailing a nose-only inhalation exposure chamber for ferrets. The exposure chamber contains multiple identical ports, most of which are used to connect directly to animal exposure tubes. Remaining ports are used to measure characteristics of the aerosol that are representative of that provided to the breathing zone of each animal on the system. The use of two glass frit impingers in series allows for a quantification of capture efficiency of test article.

This fit for purpose exposure system and analytical methods were utilized in a series of non GLP and GLP pharmacology and toxicology studies. The methods were viable for validation with ranges defined by the method development.
4.3.3. Non-human primate model with an AAV-vector
A study to evaluate safety and transgene expression of an AAV-based viral vector and CFTR transgene payload was undertaken in nonhuman primates. A key component of this program was the limited availability of the test article, which suggested the use of a system designed for direct delivery to the lungs in a non human primate. To this end, an endotracheal tube ventilation system was used in conjunction with a commercially available jet nebulizer for the delivery of test article to anesthetized animals. A small pilot study demonstrated the feasibility of pulmonary delivery with this system using Evans blue dye as a surrogate aerosol tracer, showing that dye was distributed broadly in each lung lobe throughout both the conducting airways and peripheral airspaces. The unique nature of this setup precluded simultaneous aerosol sampling and exposure. Rather, pre- and posttest gravimetric measures and qPCR analysis of rinsates from all components of the delivery system were performed to estimate the dose delivered to the animal during exposure via conservation of mass. This approach yielded ~45% of the nominal amount loaded into the nebulizer reaching the lungs of the animal – a substantial improvement over typical preclinical inhalation exposure systems. This system and method(s) were validated to support GLP toxicology in support of regulatory filings.
5. Conclusions
The present review has focused on inhalation delivery of gene therapies, with particular attention directed toward preclinical research. General characteristics of inhalation delivery were reviewed to identify advantages and challenges over other routes of exposure: inhalation delivery allows for improved on-target dosing of therapeutics for diseases manifesting in the lungs but is associated with considerable variability in dosing as well as challenges in formulation. Barriers to inhalation delivery of gene therapies, biological and non-biological in nature, include the anatomy of the respiratory tract itself, aerosolization stresses that can influence the integrity of vectors and genetic payloads, and the natural defense mechanisms of the respiratory tract including mucociliary clearance and alveolar macrophages. Preclinical research in animal models of inhalation, a critical component of establishing safety and efficacy prior to use in humans, benefits from a long history of works in inhalation toxicology and pharmaceuticals in which well-characterized exposure systems are described. One disadvantage of such systems is that delivery efficiency is typically low compared to other routes of exposure, necessitating greater amounts of test article than is often assumed.
Standardized methods for estimating the dose delivered to an animal model are available, though defining the ‘dose’ for a gene therapy requires careful consideration of biological relevance. Translatability remains an important area of discussion for gene therapy programs, and at present one cannot over-stress the importance of fit-for-purpose study designs. An important aspect of preclinical research lies in the establishment of appropriate analytical methods for characterizing biologically relevant doses in disparate animal models. Methods for characterizing DNA or RNA purity, viral vector infectivity, and nonviral vector integrity are invaluable here in determining where losses may be occurring, and whether these can be addressed by e.g. use of a different aerosolization technique, sample collection method, or analytical procedure. The wide variety of approaches in genetic payload design and (if present) vector characteristics means there is no one-size-fits-all analytical method that will satisfy the requirements of every gene therapy program. Rather, careful a priori study-specific method development can improve the quality of data obtained from preclinical study and reduce the risks of a failed Phase I, II, or III trial.
6. Expert opinion
Gene therapy approaches have an exciting potential to greatly improve our understanding and treatment of disease. An increasing number of gene therapy programs have arisen over the past several years, and the recent finalization of regulator guidance specific to gene therapies may help to accelerate gene therapy programs to successfully bring products to market [Citation1]. Regulators have encouraged sponsors to engage in early and regular dialogue through programs such as INTERACT (Initial Targeted Engagement for Regulatory Advice on CBER producTs) and Pre-IND (or Pre-BLA) meetings, and there is a strong appetite from researchers, sponsors, and regulators alike for successful developments in gene therapy programs, inhalation or otherwise. In treating diseases of the respiratory tract, inhalation delivery of therapeutic aerosols allows for delivery directly to the site(s) of intended action, reducing off-target dosing and associated deleterious side effects. Potential disease targets for gene therapies here include cystic fibrosis (CF), alpha-1 antitrypsin deficiency, asthma, and certain cancers of the lung. CF, in particular, has been the target of numerous inhaled gene therapy programs over the past few decades. Unfortunately, the development of gene therapies targeting inhalation delivery can be more complicated than other routes of exposure. Despite enormous effects, candidate inhaled gene therapies for CF have thus far failed to enter the market, often due to disappointing results in human trials despite promising preclinical work [Citation24].
The challenges encountered with CF point toward the numerous barriers, biological and otherwise, to treating disease via gene therapy aerosols. Major considerations include aerosolization stresses than can degrade therapy components, the effects of respiratory tract structure on aerosol deposition that can limit the amount of dose reaching particular regions of interest, and the natural defense mechanisms of the respiratory tract (including mucociliary clearance in the conducting airways and alveolar macrophages in the peripheral airspaces) that act to remove or break down foreign material landing in the lungs. Further complicating matters are the various approaches toward genetic payload design and (if present) vector characteristics.
A number of in vitro methods have been described that can quantify the extent of degradation occurring during aerosolization. Numerous programs have successfully nebulized gene therapy products with minimal losses, and early method development work can identify whether a particular formulation – device combination maintains a desired degree of integrity for a gene therapy. For particularly sensitive formulations, inhalation therapy would benefit from future optimization of nebulizers and other delivery devices to reduce aerosolization stresses.
Regarding respiratory tract anatomy and aerosol deposition, regional deposition is well characterized in humans, but less so in many animal models. At present, best practice uses species-dependent deposition fractions to estimate pulmonary deposition without consideration of nuanced effects of particle size, with the understanding that this is a somewhat coarse but transparent and repeatable approach. It may be possible to improve the estimation of pulmonary deposition in animal models by incorporating numerical deposition modeling with on-study measurements of particle sizes and animal-specific measurements of e.g. respiratory minute volumes and body weights, but this remains a topic for future work. It is also important to recognize differences between pulmonary delivery of a gene therapy through intratracheal bolus methods (i.e. intratracheal liquid injection or intratracheal sprays of large droplets) versus classical inhalation of respirable aerosols – important differences in regional deposition and disposition that may influence observations in preclinical study can occur with these different methods of administration. Following deposition, a gene therapy must overcome various biological barriers to enter target cells. Continued refinement of measures for overcoming biological barriers of the respiratory tract (via, e.g. mucolytic agents, muco-penetrating nanoparticles, passive and active cell targeting, etc.) will be important steps for many candidate therapies. Considering tropism, translatability between preclinical observations in animal models and expected behavior in human patients remains an extremely challenging task, and alternative methods (e.g. lung on a chip, in vitro cell models) could provide invaluable complementary information. Disease-specific animal models, e.g. CF ferrets and pigs, may also aid in improving the translatability of preclinical research for various gene therapies.
Preclinical research allows for in-depth characterization of aerosol characteristics during exposures, with the primary consideration being biologically relevant measurements of therapeutic agent concentration presented to the breathing zone of the animal. Analytical methods should thus aim to evaluate the amount of a gene therapy that is in the ideal configuration versus the amount that is denatured due to e.g. aerosolization stresses. Various methods for characterizing DNA or RNA purity, viral vector infectivity, and nonviral vector integrity or encapsulation efficiency have been described in the literature. At present, the nascent nature of inhalation gene therapy as a field of drug development means that individual gene therapy programs benefit from fit-for-purpose method development to establish appropriate techniques for aerosolization, sampling, and sample analysis. Successful methods developed for particular platforms have, in our experience, often worked for later studies on similar therapies iterating on the same vector, suggesting some potential for standardized pharmacopoeial-type methods in the future, at least for certain sub-categories of gene therapy products (e.g. determination of encapsulation efficiency for LNP platforms).
Regrettably, preclinical research for inhalation gene delivery remains somewhat under-described in the literature, perhaps due to the nascent nature of the field. We encourage researchers and sponsors to publish methods and techniques used during preclinical evaluations of inhalation gene therapy products to aid in refining experimental setups and analysis for future inhaled gene therapy programs. We expect to see accelerating interest in gene therapy products targeting inhalation delivery in coming years, and increased identification of promising approaches or unfortunate dead-ends may greatly increase the chances of bringing a successful therapy to market while avoiding costly pitfalls and redundant study.
Article highlights
Delivery directly to the site of intended action is an attractive feature for inhalation delivery of gene therapies targeting diseases of the lung, but numerous challenges complicate the development of successful candidates.
Preclinical studies are of critical importance in demonstrating safety and efficacy prior to use in clinical trials, but there are various complicating factors related to e.g. translatability.
Fit-for-purpose methods are required for individual programs to evaluate biologically relevant doses delivered to animal models during inhalation studies. The wide disparity of approaches for gene therapies (with respect to payload design and, if present, vector configuration) precludes the use of a single standardized approach for all programs.
For biological relevance of measurements on-study, analytical methods should characterize the proportion of a therapy in the aerosol that is present in the desired configuration versus the amount that may be denatured or damaged due to e.g. aerosolization stresses. Such methods may evaluate DNA or RNA purity, viral vector infectivity, or nonviral vector integrity and encapsulation efficiency.
Declaration of interest
Lovelace Biomedical Research Institute, the employer of the authors, is a nonprofit contract research organization that has received funding for preclinical research on gene therapy programs from various sponsors. The authors have no other relevant affiliations or financial involvement with any organization or entity with a financial interest in or financial conflict with the subject matter or materials discussed in the manuscript apart from those disclosed.
Reviewer disclosures
Peer reviewers on this manuscript have no relevant financial or other relationships to disclose.
Acknowledgments
The authors would like to acknowledge Krystal Biotech, Inc., Spirovant, and 4D Molecular Therapeutics for their contributions to the Example programs.
Additional information
Funding
References
- High KA. Turning genes into medicines—what have we learned from gene therapy drug development in the past decade? Nat Commun. 2020;11(1):5821. doi: 10.1038/s41467-020-19507-0
- Gottlieb S. Statement from FDA commissioner Scott Gottlieb, M.D. and Peter Marks, M.D., Ph.D., director of the center for biologics Evaluation and research on new policies to advance development of safe and effective cell and gene therapies [Internet]. FDA; 2019 [cited 2023 Apr 25]. Available from: https://www.fda.gov/news-events/press-announcements/statement-fda-commissioner-scott-gottlieb-md-and-peter-marks-md-phd-director-center-biologics
- FDA Office of Tissues and Advanced Therapies (OTAT). Approved cellular and gene therapy products [Internet]. FDA. FDA; 2023 [cited 2023 Apr 25]. Available from: https://www.fda.gov/vaccines-blood-biologics/cellular-gene-therapy-products/approved-cellular-and-gene-therapy-products
- Becerra X. Food and Drug administration: statement of organization, functions, and delegations of authority. Fed Regist. 2022;87:58806–58807.
- Chemistry, Manufacturing, and Control (CMC) Information for Human Gene Therapy Investigational New Drug Applications (INDs). Guidance for industry. Docket Number 2008-D-0205. [Internet]. U.S. Departent of Health and Human Services, Food and Drug Adminstration. Center for Biologics Evaluation and Research; 2020 [cited 2023 Apr 18]. Available from: https://www.fda.gov/regulatory-information/search-fda-guidance-documents/chemistry-manufacturing-and-control-cmc-information-human-gene-therapy-investigational-new-drug
- Zogg H, Singh R, Ro S. Current advances in RNA therapeutics for human diseases. Int J Mol Sci. 2022;23(5):2736. doi: 10.3390/ijms23052736
- Paunovska K, Loughrey D, Dahlman JE. Drug delivery systems for RNA therapeutics. Nat Rev Genet. 2022;23:265–280.
- Scholz C, Wagner E. Therapeutic plasmid DNA versus siRNA delivery: common and different tasks for synthetic carriers. Drug Deliv Res Eur. 2012;161:554–565. doi: 10.1016/j.jconrel.2011.11.014
- Roberts TC, Langer R, Wood MJA. Advances in oligonucleotide drug delivery. Nat Rev Drug Discov. 2020;19(10):673–694. doi: 10.1038/s41573-020-0075-7
- Hu B, Zhong L, Weng Y, et al. Therapeutic siRNA: state of the art. Signal Transduct Target Ther. 2020;5(1):101. doi: 10.1038/s41392-020-0207-x
- Zhou J, Rossi J. Aptamers as targeted therapeutics: current potential and challenges. Nat Rev Drug Discov. 2017;16(3):181–202. doi: 10.1038/nrd.2016.199
- Rupaimoole R, Slack FJ. MicroRNA therapeutics: towards a new era for the management of cancer and other diseases. Nat Rev Drug Discov. 2017;16(3):203–222. doi: 10.1038/nrd.2016.246
- Chow MYT, Qiu Y, Lam JKW. Inhaled RNA therapy: from promise to reality. Trends Pharmacol Sci. 2020;41:715–729.
- Bulcha JT, Wang Y, Ma H, et al. Viral vector platforms within the gene therapy landscape. Signal Transduct Target Ther. 2021;6(1):53. doi: 10.1038/s41392-021-00487-6
- McCarron A, Cmielewski P, Drysdale V, et al. Effective viral-mediated lung gene therapy: is airway surface preparation necessary? Gene Ther. 2023;30(6):469–477. doi: 10.1038/s41434-022-00332-7
- Srivastava A, Mallela KMG, Deorkar N, et al. Manufacturing challenges and rational formulation development for AAV viral vectors. J Pharm Sci. 2021;110(7):2609–2624. doi: 10.1016/j.xphs.2021.03.024
- Wang H, Qin L, Zhang X, et al. Mechanisms and challenges of nanocarriers as non-viral vectors of therapeutic genes for enhanced pulmonary delivery. J Controlled Release. 2022;352:970–993. doi: 10.1016/j.jconrel.2022.10.061
- Huang T, Gao J, Cai L, et al. Treating pulmonary fibrosis with non-viral gene therapy: from bench to bedside. Pharmaceutics. 2022;14(4):813. doi: 10.3390/pharmaceutics14040813
- Chuan D, Jin T, Fan R, et al. Chitosan for gene delivery: methods for improvement and applications. Adv Colloid Interface Sci. 2019;268:25–38. doi: 10.1016/j.cis.2019.03.007
- Hopkins C, Javius-Jones K, Wang Y, et al. Combinations of chemo-, immuno-, and gene therapies using nanocarriers as a multifunctional drug platform. Expert Opin Drug Deliv. 2022;19(10):1337–1349. doi: 10.1080/17425247.2022.2112569
- Burdett T, Nuseibeh S. Changing trends in the development of AAV-based gene therapies: a meta-analysis of past and present therapies. Gene Ther. 2023;30(3–4):323–335. doi: 10.1038/s41434-022-00363-0
- Gautam A, Waldrep JC, Densmore CL. Aerosol gene therapy. Mol Biotechnol. 2003;23:51–60. doi: 10.1385/MB:23:1:51
- Sui H, Xu X, Su Y, et al. Gene therapy for cystic fibrosis: challenges and prospects. Front Pharmacol. 2022;13:1015926. doi: 10.3389/fphar.2022.1015926
- Cooney A, McCray P, Sinn P. Cystic fibrosis gene therapy: looking back, looking forward. Genes. 2018;9(11):538. doi: 10.3390/genes9110538
- Rowe SM, Zuckerman JB, Dorgan D, et al. Inhaled mRNA therapy for treatment of cystic fibrosis: interim results of a randomized, double-blind, placebo-controlled phase 1/2 clinical study. J Cyst Fibros Off J Eur Cyst Fibros Soc. 2023;22(4):656–664. S1569-1993(23)00112-1. doi: 10.1016/j.jcf.2023.04.008
- Tepper JS, Kuehl PJ, Cracknell S, et al. Symposium summary: “breathe in, breathe out, its easy: what you need to know about developing inhaled drugs. Int J Toxicol. 2016;35(4):376–392. doi: 10.1177/1091581815624080
- Preclinical Assessment of Investigational Cellular and Gene Therapy Products. Docket number FDA-2012-D-1038. [Internet]. U.S. Departent of Health and Human Services, Food and Drug Adminstration. Center for Biologics Evaluation and Research; 2019 [cited 2023 May 1]. Available from: https://www.fda.gov/regulatory-information/search-fda-guidance-documents/preclinical-assessment-investigational-cellular-and-gene-therapy-products
- Ehrmann S, Schmid O, Darquenne C, et al. Innovative preclinical models for pulmonary drug delivery research. Expert Opin Drug Deliv. 2020;17(4):463–478. doi: 10.1080/17425247.2020.1730807
- Selo MA, Sake JA, Kim KJ, et al. In vitro and ex vivo models in inhalation biopharmaceutical research — advances, challenges and future perspectives. Adv Drug Deliv Rev. 2021;177:113862. doi: 10.1016/j.addr.2021.113862
- Ramamurthy RM, Atala A, Porada CD, et al. Organoids and microphysiological systems: promising models for accelerating AAV gene therapy studies. Front Immunol. 2022;13:1011143. doi: 10.3389/fimmu.2022.1011143
- Stein SW, Thiel CG. The history of therapeutic aerosols: a chronological review. J Aerosol Med Pulm Drug Deliv. 2016;30:20–41. doi: 10.1089/jamp.2016.1297
- Pires Ferreira D, Gruntman AM, Flotte TR. Gene therapy for alpha-1 antitrypsin deficiency: an update. Expert Opin Biol Ther. 2023;23(3):283–291. doi: 10.1080/14712598.2023.2183771
- El-Husseini ZW, Gosens R, Dekker F, et al. The genetics of asthma and the promise of genomics-guided drug target discovery. Lancet Respir Med. 2020;8(10):1045–1056. doi: 10.1016/S2213-2600(20)30363-5
- Lee WH, Loo CY, Ghadiri M, et al. The potential to treat lung cancer via inhalation of repurposed drugs. Adv Drug Deliv Rev. 2018;133:107–130. doi: 10.1016/j.addr.2018.08.012
- Martin AR, Moore CP, Finlay WH. Models of deposition, pharmacokinetics, and intersubject variability in respiratory drug delivery. Expert Opin Drug Deliv. 2018;15(12):1175–1188. doi: 10.1080/17425247.2018.1544616
- Ruge CC, Kirch J, Lehr CM. Pulmonary drug delivery: from generating aerosols to overcoming biological barriers-therapeutic possibilities and technological challenges. Lancet Respir Med. 2013;1:402–413. doi: 10.1016/S2213-2600(13)70072-9
- Loira-Pastoriza C, Todoroff J, Vanbever R. Delivery strategies for sustained drug release in the lungs. Adv Drug Deliv Rev. 2014;75:81–91. doi: 10.1016/j.addr.2014.05.017
- Hastedt JE, Bäckman P, Clark AR, et al. Scope and relevance of a pulmonary biopharmaceutical classification system AAPS/FDA/USP workshop March 16-17th, 2015 in Baltimore, MD. Am Assoc Pharm Sci Open. 2016;2:1. doi: 10.1186/s41120-016-0005-2
- Kubczak M, Michlewska S, Bryszewska M, et al. Nanoparticles for local delivery of siRNA in lung therapy. Adv Drug Deliv Rev. 2021;179:114038. doi: 10.1016/j.addr.2021.114038
- Chow MYT, Chang RYK, Chan H-K. Inhalation delivery technology for genome-editing of respiratory diseases. Adv Drug Deliv Rev. 2021;168:217–228. doi: 10.1016/j.addr.2020.06.001
- Gomes Dos Reis L, Svolos M, Hartwig B, et al. Inhaled gene delivery: a formulation and delivery approach. Expert Opin Drug Deliv. 2017;14:319–330. doi: 10.1080/17425247.2016.1214569
- Finlay WH. The mechanics of inhaled pharmaceutical aerosols : an introduction. San Diego: Academic Press; 2019. doi: 10.1016/B978-0-08-102749-3.00001-4
- International Commission on Radiological Protection. Human respiratory tract model for radiological protection: a report of a task group of the International Commission on Radiological protection. Oxford, Eng : Tarrytown (N.Y): published for the International Commission on Radiological Protection by Pergamon; 1994.
- Hofmann W. Regional deposition: deposition models. J Aerosol Med Pulm Drug Deliv. 2020;33(5):239–248. doi: 10.1089/jamp.2020.29031.wh
- Miller FJ, Asgharian B, Schroeter JD, et al. Improvements and additions to the multiple path particle dosimetry model. J Aerosol Sci. 2016;99:14–26. doi: 10.1016/j.jaerosci.2016.01.018
- Ruzycki CA, Pawluski D, Wong EYL, et al. Provocative dose determination for methacholine challenge test aerosols through in vitro – in silico methods. J Aerosol Sci. 2023;171:106184. doi: 10.1016/j.jaerosci.2023.106184
- Kim N, Duncan GA, Hanes J, et al. Barriers to inhaled gene therapy of obstructive lung diseases: a review. J Controlled Release. 2016;240:465–488. doi: 10.1016/j.jconrel.2016.05.031
- Griesenbach U, Alton EWFW. Gene transfer to the lung: lessons learned from more than 2 decades of CF gene therapy. Adv Drug Deliv Rev. 2009;61:128–139. doi: 10.1016/j.addr.2008.09.010
- Ari A, Fink JB. Recent advances in aerosol devices for the delivery of inhaled medications. Expert Opin Drug Deliv. 2020;17(2):133–144. doi: 10.1080/17425247.2020.1712356
- Martin AR, Finlay WH. Nebulizers for drug delivery to the lungs. Expert Opin Drug Deliv. 2015;12(6):889–900. doi: 10.1517/17425247.2015.995087
- Weers JG, Miller DP. Formulation design of dry powders for inhalation. J Pharm Sci. 2015;104(10):3259–3288. doi: 10.1002/jps.24574
- Vehring R, Snyder H, Lechuga-Ballesteros D. Spray Drying. In: Ohtake S, Izutsu K-i, and Lechuga-Ballesteros D, editors. Drying Technologies for Biotechnology and Pharmaceutical Applicatoins. Weinheim, Germany: Wiley-VCH; 2020. p.179–216. doi: 10.1002/9783527802104.ch7
- Myrdal PB, Sheth P, Stein SW. Advances in metered dose inhaler technology: formulation development. AAPS Pharm Sci Tech. 2014;15(2):434–455. doi: 10.1208/s12249-013-0063-x
- Conti DS, Bharatwaj B, Brewer D, et al. Propellant-based inhalers for the non-invasive delivery of genes via oral inhalation. J Controlled Release. 2012;157(3):406–417. doi: 10.1016/j.jconrel.2011.09.089
- Carrigy NB, Chang RY, Leung SSY, et al. Anti-tuberculosis bacteriophage D29 delivery with a vibrating mesh nebulizer, Jet nebulizer, and soft mist inhaler. Pharm Res. 2017;34(10):2084–2096. doi: 10.1007/s11095-017-2213-4
- Lentz YK, Anchordoquy TJ, Lengsfeld CS. Rationale for the selection of an aerosol delivery system for gene delivery. J Aerosol Med. 2006; 19(3):372–384. doi: 10.1089/jam.2006.19.372
- Deshpande D, Blanchard J, Srinivasan S, et al. Aerosolization of lipoplexes using AERx® pulmonary delivery system. AAPS PharmSci. 2002;4:12–21. doi: 10.1208/ps040313
- Arulmuthu ER, Williams DJ, Baldascini H, et al. Studies on aerosol delivery of plasmid DNA using a mesh nebulizer. Biotechnol Bioeng. 2007;98(5):939–955. doi: 10.1002/bit.21493
- Gomes Dos Reis L, Svolos M, Moir LM, et al. Delivery of pDNA Polyplexes to bronchial and alveolar epithelial cells using a mesh nebulizer. Pharm Res. 2019;36:14. doi: 10.1007/s11095-018-2542-y
- Lentz YK, Worden LR, Anchordoquy TJ, et al. Effect of jet nebulization on DNA: identifying the dominant degradation mechanism and mitigation methods. J Aerosol Sci. 2005;36(8):973–990. doi: 10.1016/j.jaerosci.2004.11.017
- Catanese DJ, Fogg JM, Schrock DE, et al. Supercoiled minivector DNA resists shear forces associated with gene therapy delivery. Gene Ther. 2012;19(1):94–100. doi: 10.1038/gt.2011.77
- Lengsfeld CS, Anchordoquy TJ. Shear‐induced degradation of plasmid DNA. J Pharm Sci. 2002;91(7):1581–1589. doi: 10.1002/jps.10140
- Zabner J, Fasbender AJ, Moninger T, et al. Cellular and molecular barriers to gene transfer by a cationic lipid. J Biol Chem. 1995;270(32):18997–19007. doi: 10.1074/jbc.270.32.18997
- Ibraheem D, Elaissari A, Fessi H. Gene therapy and DNA delivery systems. Int J Pharm. 2014;459(1–2):70–83. doi: 10.1016/j.ijpharm.2013.11.041
- Banks GA, Roselli RJ, Chen R, et al. A model for the analysis of nonviral gene therapy. Gene Ther. 2003;10(20):1766–1775. doi: 10.1038/sj.gt.3302076
- Emerson M, Renwick L, Tate S, et al. Transfection efficiency and toxicity following delivery of naked plasmid DNA and cationic lipid–DNA complexes to ovine lung segments. Mol Ther. 2003;8(4):646–653. doi: 10.1016/S1525-0016(03)00233-8
- Ichikawa M, Muramatsu N, Matsunaga W, et al. Effects of inhalable gene transfection as a novel gene therapy for non-small cell lung cancer and malignant pleural mesothelioma. Sci Rep. 2022;12(1):8634. doi: 10.1038/s41598-022-12624-4
- Woodside MT, García-García C, Block SM. Folding and unfolding single RNA molecules under tension. Curr Opin Chem Biol. 2008;12(6):640–646. doi: 10.1016/j.cbpa.2008.08.011
- Bustamante C, Smith SB, Liphardt J, et al. Single-molecule studies of DNA mechanics. Curr Opin Struct Biol. 2000;10(3):279–285. doi: 10.1016/S0959-440X(00)00085-3
- Tahara K, Hashimoto W, Takeuchi H. Inhalation properties and stability of nebulized naked siRNA solution for pulmonary therapy. Chem Pharm Bull (Tokyo). 2016;64:63–67. doi: 10.1248/cpb.c15-00615
- Cortez-Jugo C, Masoumi S, Chan PPY, et al. Nebulization of siRNA for inhalation therapy based on a microfluidic surface acoustic wave platform. Ultrason Sonochem. 2022;88:106088. doi: 10.1016/j.ultsonch.2022.106088
- Morgan BA, Manser M, Jeyanathan M, et al. Effect of shear stresses on adenovirus activity and aggregation during atomization to produce thermally stable vaccines by spray drying. ACS Biomater Sci Eng. 2020;6(7):4304–4313. doi: 10.1021/acsbiomaterials.0c00317
- Roy CJ, Ault A, Sivasubramani SK, et al. Aerosolized adenovirus-vectored vaccine as an alternative vaccine delivery method. Respir Res. 2011;12(1):153. doi: 10.1186/1465-9921-12-153
- Klein DM, Poortinga A, Verhoeven FM, et al. Degradation of lipid based drug delivery formulations during nebulization. Chem Phys. 2021;547:111192. doi: 10.1016/j.chemphys.2021.111192
- Kim J, Jozic A, Lin Y, et al. Engineering lipid nanoparticles for enhanced intracellular delivery of mRNA through inhalation. ACS Nano. 2022;16(9):14792–14806. doi: 10.1021/acsnano.2c05647
- Phalen RF, Yeh HC, Prasad SB. Morphology of the respiratory tract. In: McClellan R Henderson R, editors. Concepts in inhalation toxicology. 2nd ed. Washington DC: Taylor & Francis; 1995. p. 129–149. doi: 10.1201/b14404-9
- Harkema JR, Plopper CG, Pinkerton KE. Comparative structure of the respiratory tract: airway architecture in humans and animals. In: Cohen M, Zelikoff J, and Schlesinger R, editors. Pulmonary Immunotoxicology. Boston (MA): Springer US; 2000. p. 1–59. doi: 10.1007/978-1-4615-4535-4_1
- Borgström L, Olsson B, Thorsson L. Degree of throat deposition can explain the variability in lung deposition of inhaled drugs. J Aerosol Med Off. 2006;19(4):473–483. doi: 10.1089/jam.2006.19.473
- Ruzycki CA, Yang M, Chan H-K, et al. Improved prediction of intersubject variability in extrathoracic aerosol deposition using algebraic correlations. Aerosol Sci Technol. 2017;51(6):667–673. doi: 10.1080/02786826.2017.1306640
- Yang MY, Ruzycki CA, Verschuer J, et al. Examining the ability of empirical correlations to predict subject specific in vivo extrathoracic aerosol deposition during tidal breathing. Aerosol Sci Technol. 2017;51(3):363–376. doi: 10.1080/02786826.2016.1262532
- Usmani OS, Roche N, Jenkins M, et al. Consistent pulmonary Drug delivery with whole lung deposition using the aerosphere inhaler: a review of the evidence. Int J Chron Obstruct Pulmon Dis. 2021;16:113–124. doi: 10.2147/COPD.S274846
- Delvadia RR, Hindle M, Longest PW, et al. In vitro tests for aerosol deposition II: IVIVCs for different dry powder inhalers in normal adults. J Aerosol Med Pulm Drug Deliv. 2012;26:138–144. doi: 10.1089/jamp.2012.0975
- Ruzycki CA, Finlay WH, Martin AR. Estimating clinically relevant measures of inhaled pharmaceutical aerosol performance with advanced in vitro and in silico methods. In: Narang A Mahato R, editors. Organ specific drug delivery and targeting to the lungs. CRC Press; 2022. p. 3–46. doi: 10.1201/9781003182566-2
- Weers JG, Miller DP, Tarara TE. Improved targeting of dry powder formulations of inhaled corticosteroids to children. Respir Drug Deliv. 2022;2022:1–8.
- Weers JG, Son YJ, Glusker M, et al. Idealhalers versus realhalers: is it possible to bypass deposition in the upper respiratory tract? J Aerosol Med Pulm Drug Deliv. 2019;32(2):55–69. doi: 10.1089/jamp.2018.1497
- Farkas D, Thomas ML, Hassan A, et al. Near elimination of in vitro Predicted extrathoracic aerosol deposition in children using a spray-Dried Antibiotic formulation and pediatric air-jet DPI. Pharm Res. 2023;40(5):1193–1207. doi: 10.1007/s11095-022-03316-9
- Holbrook LT, Longest PW. Validating CFD predictions of highly localized aerosol deposition in airway models: in vitro data and effects of surface properties. J Aerosol Sci. 2013;59:6–21. doi: 10.1016/j.jaerosci.2013.01.008
- Lizal F, Elcner J, Jedelsky J, et al. The effect of oral and nasal breathing on the deposition of inhaled particles in upper and tracheobronchial airways. J Aerosol Sci. 2020;150:105649. doi: 10.1016/j.jaerosci.2020.105649
- Ruzycki CA, Murphy B, Nathoo H, et al. Combined in vitro-in silico approach to predict deposition and pharmacokinetics of budesonide dry powder inhalers. Pharm Res. 2020;37(10):209. doi: 10.1007/s11095-020-02924-7
- Tavernini S, Farina DJ, Martin AR, et al. Using filters to estimate regional lung deposition with dry powder inhalers. Pharm Res. 2021;38(9):1601–1613. doi: 10.1007/s11095-021-03082-0
- Finlay WH, Farina DJ, Tavernini S, et al. In vitro estimation of tracheobronchial and alveolar doses using filters. Front Drug Deliv. 2022;2:1–7. doi: 10.3389/fddev.2022.901289
- Stocks J, Hislop AA. Structure and function of the respiratory system: developmental aspects and their relevance to aerosol therapy. In: Bisgaard H, O’Callaghan C Smaldone G, editors. Drug delivery to the lung. New York (NY): Marcel Dekker; 2002. p. 47–104. doi: 10.1201/b14022-4
- Cohen-Cymberknoh M, Kerem E, Ferkol T, et al. Airway inflammation in cystic fibrosis: molecular mechanisms and clinical implications. Thorax. 2013;68(12):1157–1162. doi: 10.1136/thoraxjnl-2013-203204
- Martin AR. Regional deposition: targeting. J Aerosol Med Pulm Drug Deliv. 2021;34(1):1–10. doi: 10.1089/jamp.2021.29033.am
- Schürch S, Gehr P, Im Hof V, et al. Surfactant displaces particles toward the epithelium in airways and alveoli. Respir Physiol. 1990;80(1):17–32. doi: 10.1016/0034-5687(90)90003-H
- Pangeni R, Meng T, Poudel S, et al. Airway mucus in pulmonary diseases: muco-adhesive and muco-penetrating particles to overcome the airway mucus barriers. Int J Pharm. 2023;634:122661. doi: 10.1016/j.ijpharm.2023.122661
- Hewitt RJ, Lloyd CM. Regulation of immune responses by the airway epithelial cell landscape. Nat Rev Immunol. 2021; 21(6):347–362. doi: 10.1038/s41577-020-00477-9
- Laube BL. Aerosolized medications for gene and peptide therapy. Respir Care. 2015;60(6):806–824. doi: 10.4187/respcare.03554
- Huffnagle GB, Dickson RP, Lukacs NW. The respiratory tract microbiome and lung inflammation: a two-way street. Mucosal Immunol. 2017; 10(2):299–306. doi: 10.1038/mi.2016.108
- Capasso C, Hirvinen M, Cerullo V. Beyond gene delivery: strategies to engineer the surfaces of viral vectors. Biomedicines. 2013;1(1):3–16. doi: 10.3390/biomedicines1010003
- Lee AY, Cho M-H, Kim S. Recent advances in aerosol gene delivery systems using non-viral vectors for lung cancer therapy. Expert Opin Drug Deliv. 2019;16(7):757–772. doi: 10.1080/17425247.2019.1641083
- Manunta MDI, McAnulty RJ, Tagalakis AD, et al. Nebulisation of receptor-targeted nanocomplexes for gene delivery to the airway epithelium. PLoS One. 2011;6(10):e26768. doi: 10.1371/journal.pone.0026768
- Wolff RK, Dorato MA. Toxicologic testing of inhaled pharmaceutical aerosols. Crit Rev Toxicol. 1993;23(4):343–369. doi: 10.3109/10408449309104076
- In: McClellan RO, Henderson RF, editors. Concepts in inhalation toxicology. 2nd ed. Washington DC: Taylor & Francis; 1995. doi: 10.1201/b14404
- Wolff RK. Toxicology studies for inhaled and nasal delivery. Mol Pharm. 2015;12(8):2688–2696. doi: 10.1021/acs.molpharmaceut.5b00146
- Alexander DJ, Collins CJ, Coombs DW, et al. Association of Inhalation Toxicologists (AIT) working party recommendation for standard delivered dose calculation and expression in non-clinical aerosol inhalation toxicology studies with pharmaceuticals. Inhal Toxicol. 2008;20(13):1179–1189. doi: 10.1080/08958370802207318
- Kuehl PJ, Chand R, McDonald JD, et al. Pulmonary and regional deposition of nebulized and dry powder aerosols in ferrets. AAPS Pharm Sci Tech. 2019;20(6):242. doi: 10.1208/s12249-019-1382-3
- Nadithe V, Rahamatalla M, Finlay WH, et al. Evaluation of nose-only aerosol inhalation chamber and comparison of experimental results with mathematical simulation of aerosol deposition in mouse lungs. J Pharm Sci. 2003;92(5):1066–1076. doi: 10.1002/jps.10379
- McCarron A, Parsons D, Donnelley M. Animal and cell culture models for cystic fibrosis. Am J Pathol. 2021;191(2):228–242. doi: 10.1016/j.ajpath.2020.10.017
- Yan Z, Stewart ZA, Sinn PL, et al. Ferret and pig models of cystic fibrosis: prospects and promise for gene therapy. Hum Gene Ther Clin Dev. 2015;26(1):38–49. doi: 10.1089/humc.2014.154
- Sun X, Yi Y, Yan Z, et al. In utero and postnatal VX-770 administration rescues multiorgan disease in a ferret model of cystic fibrosis. Sci Transl Med. 2019;11(485):11. doi: 10.1126/scitranslmed.aau7531
- Gautam A, Densmore C, Waldrep J. Pulmonary cytokine responses associated with PEI–DNA aerosol gene therapy. Gene Ther. 2001;8(3):254–257. doi: 10.1038/sj.gt.3301369
- Densmore CL, Orson FM, Xu B, et al. Aerosol delivery of robust polyethyleneimine– DNA complexes for gene therapy and genetic immunization. Mol Ther. 2000;1(2):180–188. doi: 10.1006/mthe.1999.0021
- Bai X, Zhao G, Chen Q, et al. Inhaled siRNA nanoparticles targeting IL11 inhibit lung fibrosis and improve pulmonary function post-bleomycin challenge. Sci Adv. 2022;8(25):eabn7162. doi: 10.1126/sciadv.abn7162
- Lokugamage MP, Vanover D, Beyersdorf J, et al. Optimization of lipid nanoparticles for the delivery of nebulized therapeutic mRNA to the lungs. Nat Biomed Eng. 2021;5(9):1059–1068. doi: 10.1038/s41551-021-00786-x
- Patel AK, Kaczmarek JC, Bose S, et al. Inhaled nanoformulated mRNA polyplexes for protein production in lung epithelium. Adv Mater. 2019;31(8):1805116. doi: 10.1002/adma.201805116
- Le Gall T, Berchel M, Davies L, et al. Aerosol-mediated non-viral lung gene therapy: the potential of aminoglycoside-based cationic liposomes. Pharmaceutics. 2021;14(1):25. doi: 10.3390/pharmaceutics14010025
- Zamora-Avila DE, Zapata-Benavides P, Franco-Molina MA, et al. WT1 gene silencing by aerosol delivery of PEI–RNAi complexes inhibits B16-F10 lung metastases growth. Cancer Gene Ther. 2009;16(12):892–899. doi: 10.1038/cgt.2009.35
- McIntyre C, Donnelley M, Rout-Pitt N, et al. Lobe-specific gene vector delivery to rat lungs using a miniature bronchoscope. Hum Gene Ther Methods. 2018;29(5):228–235. doi: 10.1089/hgtb.2018.050
- Weiss DJ, Mutlu GM, Bonneau L, et al. Comparison of surfactant and perfluorochemical liquid enhanced adenovirus-mediated gene transfer in normal rat lung. Mol Ther J Am Soc Gene Ther. 2002;6(1):43–49. doi: 10.1006/mthe.2002.0632
- Bisserier M, Mathiyalagan P, Zhang S, et al. Regulation of the methylation and expression levels of the BMPR2 gene by SIN3a as a novel therapeutic mechanism in pulmonary arterial hypertension. Circulation. 2021;144(1):52–73. doi: 10.1161/CIRCULATIONAHA.120.047978
- Koehler DR, Frndova H, Leung K, et al. Aerosol delivery of an enhanced helper-dependent adenovirus formulation to rabbit lung using an intratracheal catheter. J Gene Med. 2005;7(11):1409–1420. doi: 10.1002/jgm.797
- Aguero J, Ishikawa K, Hadri L, et al. Intratracheal gene delivery of SERCA2a ameliorates chronic post-capillary pulmonary hypertension. J Am Coll Cardiol. 2016;67(17):2032–2046. doi: 10.1016/j.jacc.2016.02.049
- Cooney AL, Sinn PL. Intratracheal aerosolization of viral vectors to newborn pig airways. Biotechniques. 2020;68(5):235–239. doi: 10.2144/btn-2019-0150
- Cao H, Machuca TN, Yeung JC, et al. Efficient gene delivery to pig airway epithelia and submucosal glands using helper-dependent adenoviral vectors. Mol Ther Nucleic Acids. 2013;2:e127. doi: 10.1038/mtna.2013.55
- McLachlan G, Baker A, Tennant P, et al. Optimizing aerosol gene delivery and expression in the ovine lung. Mol Ther. 2007;15(2):348–354. doi: 10.1038/sj.mt.6300058
- Legere RM, Cohen ND, Poveda C, et al. Safe and effective aerosolization of in vitro transcribed mRNA to the respiratory tract epithelium of horses without a transfection agent. Sci Rep. 2021;11(1):371. doi: 10.1038/s41598-020-79855-1
- Driscoll KE. Intratracheal instillation as an exposure technique for the evaluation of respiratory tract toxicity: uses and limitations. Toxicol Sci. 2000;55(1):24–35. doi: 10.1093/toxsci/55.1.24
- Osier M, Oberdorster G. Intratracheal inhalation vs intratracheal instillation: differences in particle effects. Fundam Appl Toxicol. 1997;40:220–227. doi: 10.1006/faat.1997.2390
- Yang L, Feuchtinger A, Möller W, et al. Three-dimensional quantitative co-mapping of pulmonary morphology and nanoparticle distribution with cellular resolution in nondissected murine lungs. ACS Nano. 2019;13(2):1029–1041. doi: 10.1021/acsnano.8b07524
- Bush E, Nicholas A, Pei T, et al. Targeting aEnac with an epithelial RNAi trigger delivery platform for the treatment of cystic fibrosis. Eur Respir J. 2018;52:p. OA514.
- Cheever TR, Berkley D, Braun S, et al. Perspectives on best practices for gene therapy programs. Hum Gene Ther. 2015;26:127–133. doi: 10.1089/hum.2014.147
- Design and Analysis of Shedding Studies for Virus or Bacteria-Based Gene Therapy and Oncolytic Products. Docket Number FDA-2014-D-0852. [Internet]. U.S. Departent of Health and Human Services, Food and Drug Adminstration. Center for Biologics Evaluation and Research; 2019 [cited 2023 May 18]. Available from: https://www.fda.gov/regulatory-information/search-fda-guidance-documents/design-and-analysis-shedding-studies-virus-or-bacteria-based-gene-therapy-and-oncolytic-products
- U.S. Pharmacopeia. General chapter, <601> inhalation and nasal Drug products: aerosols, sprays, and powders - performance quality tests. USP-NF. Rockville (MD): Untied States Pharmacopeia; 2023.
- U.S. Pharmacopeia. General chapter, <1601> products for nebulization - characterization tests. USP-NF. Rockville (MD): Untied States Pharmacopeia; 2023.
- Cipolla D, Wu H, Gonda I, et al. Aerosol performance and stability of liposomes containing ciprofloxacin nanocrystals. J Aerosol Med Pulm Drug Deliv. 2015;28(6):411–422. doi: 10.1089/jamp.2015.1241
- European Pharmacopeia. Ph Eur 9.8 general chapter 2.9.44 preparations for nebulization - characterization. Strasbourg (France): Directorate for the Quality of Medicines and HealthCare of the Council of Europe (EDQM); 2019.
- Li B, Manan RS, Liang S-Q, et al. Combinatorial design of nanoparticles for pulmonary mRNA delivery and genome editing. Nat Biotechnol. 2023. doi: 10.1038/s41587-023-01679-x