ABSTRACT
Possible involvement of calcium (Ca) and zinc (Zn) in mitigation of salt (NaCl) stress-induced oxidative damage in Brassica juncea was investigated. Salt stress (200 mM NaCl) reduced leaf pigment synthesis and some key photosynthetic attributes including stomatal conductance and internal CO2 concentration. Exogenous application of Ca and Zn resulted in enhanced growth possibly by induction of the antioxidant defense system, resulting in improved redox state thereby favoring growth improvement. Proline accumulation (3.39-fold) was stimulated by exogenous application of Zn and Ca causing improvement in growth through enhancement in relative water content (78.46%) and increased flavonoid accumulation (86.19%). NaCl stress enhanced the hydrogen peroxide (H2O2), malondialdehyde and methylglyoxal content by 3-fold, 1.51-fold, and 2.98-fold, respectively, however, supplementation of Ca and Zn individually as well as in combination reduced the accumulation to an appreciable level. Ca and Zn treatment helped Brassica juncea plants to strengthen the antioxidant system and glyoxalase system and also enzymes of ascorbate-glutathione (AsA-Glu) cycle for better protection to membranes from reactive oxygen species. Moreover, Ca and Zn supplementation reduced the salt-induced damage by maintaining Na/K ratio through improved K uptake.
1. Introduction
Of several abiotic stresses prevalent on the globe, salinity stress is important to study because it is spreading at a very high speed, leading to the conversion of fertile land to derelict lands. Increased salinity has affected approximately 7% of total land surface globally and 20% of the agricultural land (Munns and Tester Citation2008, Cabot et al. Citation2014). Salinity is known to decrease plant growth and productivity due to ionic and osmotic stresses. Salinity stress decreases the yield and yield components in rice seedlings (Mohammadi et al. Citation2013). Kumar and Khare (Citation2016) also reported reduced grain yield and quality of yield in rice seedlings under NaCl stress. Accumulation of Na+ has been reported to hamper many important biological functions such as photosynthesis, respiration, and uptake of nutrients (Ahmad et al. Citation2015, Ahmad et al. Citation2016b, Ahanger and Agarwal Citation2017). Like other stresses, high salinity leads to overproduction of reactive oxygen species (ROS) leading to oxidative effects on key and sensitive biomolecules, including membrane lipids, proteins, and nucleic acids, etc. (Ahmad et al. Citation2010). Plants counteract the stress-induced changes by regulating their defense systems such as compatible solutes and antioxidants. The antioxidants are enzymatic and non-enzymatic, while compatible osmolytes include proline, sugars, and amino acids, which provide protection to the biomolecules (Ahanger et al. Citation2015). Such protective mechanisms protect plants from salinity-induced oxidative and ionic effects, minimizing the excess generation of ROS, and hence protecting cellular functioning (Ahmad et al. Citation2010, Ahmad et al. Citation2015). Ascorbate-glutathione cycle (AsA-Glu cycle) is playing a major role in cell detoxification under abiotic stress (Noctor and Foyer Citation1998). AsA-Glu cycle uses ascorbate as electron donor and reduces H2O2 to H2O (Smirnoff Citation2000). Methylglyoxal (MG) is highly reactive and is elevated under abiotic stress (Yadav et al. Citation2005, Hoque et al. Citation2012, Hoque et al. Citation2016). Plants are equipped with glutathione (GSH)-dependent glyoxalase (Gly) system to tackle this MG stress. Antioxidant system in collaboration with glyoxalase system enhanced the abiotic stress tolerance in plants (Nahar et al. Citation2015).
Application of mineral nutrients is a very potential strategy for the maintenance of plant growth and development. Various nutrient elements result in alleviation of abiotic stresses in plants and help the plant to flourish well under stressful conditions (Ahanger et al. Citation2015, Ahmad et al. Citation2015, Siddiqui et al. Citation2015). Zinc (Zn) and calcium (Ca) are important elements, which play a leading role in plant stress avoidance (Rezaei and Abbasi Citation2014, Ahmad et al. Citation2015). Zinc is an important component of DNA binding proteins, the Zn-fingers, and is also involved in enzyme activity, stability of membranes, and proteins structure (Aravind and Prasad Citation2004, Tavallali et al. Citation2009). Apart from being an important component of antioxidant enzyme, Cu/Zn-SOD (superoxide dismutase) (Ahmad et al. Citation2010), Zn acts as cofactor of many enzymes and is also associated with protein synthesis, cell division and other metabolic activities of the cell. Due to the low solubility of Zn in the soil solution, it becomes unavailable to roots leading to its deficiency in plants.
Calcium, being a macronutrient, is essential for the normal growth and development of plants, because it plays an important role in stabilization of membrane structures, enhances uptake of nutrients, and activates metabolic processes (Tuna et al. Citation2007, Sarwat et al. Citation2013). Calcium also alleviates the deleterious stress effects by modulating the antioxidant metabolism (Zorrig et al. Citation2012, Ahmad et al. Citation2015). Wu and Wang (Citation2012) have ascribed the alleviatory role of exogenously applied Ca to its ability to induce ionic homeostasis. However, the role of Ca and Zn in the regulation of salinity stress has not been elucidated properly, and thus it needs to be studied in detail.
Mustard (Brassica juncea L.) is an important and major oil-yielding crop worldwide. The oil content of mustard seedlings is decreasing due to salinity stress. Apart from having medicinal properties, mustard (residual part) is also used as a fertilizer and fodder for cattle. The production of mustard is adversely affected by salinity stress (Ashraf and McNeilly Citation2004). Considering the increasing salinity problem and limited use of micronutrients in mitigating the stress, the present study aimed to investigate the effect of Ca and Zn, either individually or in combination, for improving tolerance of mustard plants against NaCl stress.
2. Material and methods
2.1. Plant materials and growth conditions
Brassica juncea (Czern. & Cross.) seeds were kept in Petri-plates lined with Whatman1 filter paper in seed germinator (temperature = 25 ± 0.5°C, photoperiod = 16 h, light intensity = 175 μmol m−2 s−1) for 4 days. The germinated seedlings were transferred to pots containing peat, perlite, and sand (1:1:1) and were given full strength Hoagland’s nutrient solution (200 mL per pot) for 2 weeks on every alternate day. After 15 days of seedling growth, the pots were irrigated with modified Hoagland’s nutrient solution containing 0 (control) or 200 mM NaCl. Exogenous application of zinc (ZnSO4·7H2O, 1 mM) and calcium (CaCl2, 50 mM) was started 25 days after sowing (DAS) (i.e. 10 days after stress imposition) individually or in combination. The control plants were applied with equal amount of distilled water. Plants were maintained under controlled conditions at day/night temperature of 26/16°C, with five replicates laid in a randomized block design. Plants were harvested for analysis after 60 days after treatment (85 days old plants) and some seedlings in the pots were allowed to grow till maturity to obtain the yield parameters.
2.2. Growth and dry weight (DW)
Shoot and root lengths were measured using a manual scale. The samples were oven-dried for 72 h at 65°C for estimation of shoot and root DWs.
2.3. Yield attributes
At maturity (110-day-old plant) plants were harvested and the data related to the yield were recorded.
2.4. Oil content estimation
Solvent extraction methods were used for the estimation of oil content. Mustard seeds (3 g) were grinded in the presence of sodium sulfate (Na2SO4) and the powder was kept in a test tube. Hexane (20 mL) was added to the test tube as mobile phase. Elute containing oil was taken in a vial and hot water bath was used to evaporate hexane. Then the oil collected was weight and its percentage was calculated by the formula:
2.5. Estimation of pigment content
For estimation of leaf chlorophyll pigments, fresh leaf samples (100 mg) were immersed in tubes containing dimethyl sulfoxide (DMSO, 10 mL) and kept at 65°C for 40 min. Thereafter, 1 mL aliquot was mixed with 2 mL of DMSO, and the absorbance of the solution was recorded at 480, 510, 645, 663 nm spectrophotometrically (Beckman 640 D, USA) using DMSO as blank (Hiscox and Israelstam Citation1979).
2.6. Estimation of gas exchange parameters
Measurement of gas exchange parameters, including net CO2 assimilation rate (A), stomatal conductance (gs), and transpiration rate (E), were measured in intact leaves using a portable infrared gas analyzer (LCA-4 model, Analytical Development Company, Hoddesdon, England).
2.7. Determination of leaf relative water content (LRWC) and electrolyte leakage
LRWC was determined in fresh leaves by employing the method of Smart and Bingham (Citation1974). Briefly fresh leaf discs were taken and their fresh weight (FW) recorded, and subsequently, they were floated on water for 4 h. The turgid weight (TW) was then noted. Thereafter, leaf discs were oven-dried at 75°C, and the DW recorded. Percent LRWC was calculated using the following formula:
For electrolyte leakage determination, fresh leaf discs were transferred into deionized water (10 mL) and the electrical conductivities (ECs) were recorded after incubating the contents at 0 (EC0), 60°C (EC1), and 100°C (EC2) in a water bath (Dionisio-Sese and Tobita Citation1998). Calculations were done as per following formula:
2.8. Estimation of proline content
Plant tissue weighing 300 mg was extracted in 10 mL of sulfosalicylic acid (3%). The homogenate was centrifuged at 10,000 × g for 15 min. The supernatant (2 mL) was incubated at 100°C for 1 h after mixing with equal quantity of glacial acetic acid and acid ninhydrin. Thereafter, the tubes were removed, and the reaction was terminated on ice bath. The proline was separated with 4 mL toluene (Bates et al. Citation1973). The absorbance was measured at 520 nm using toluene as a blank.
2.9. Estimation of hydrogen peroxide (H2O2), malondialdehyde (MDA) and MG
For hydrogen peroxide (H2O2) measurement, fresh leaf samples (500 mg) were macerated in 0.1% trichloroacetic acid (TCA), and the homogenate was centrifuged at 12,000 × g for 15 min. The supernatant (0.5 mL) was mixed with equal volume of 10 mM potassium phosphate buffer (pH 7.0) followed by addition of 1 mL potassium iodide (1 M) solution. Optical density was recorded at 390 nm (Velikova et al. Citation2000).
Lipid peroxidation was estimated by measuring the MDA content formation following the procedure of Rao and Sresty (Citation2000). Fresh leaves (500 mg) were homogenized in 0.1% (w/v) TCA and centrifuged at 10,000 × g for 10 min. The supernatant (1 mL) was reacted with 20% TCA (4 mL) containing 0.5% (w/v) thiobarbituric acid and subsequently heated for 30 min at 95°C. The samples were removed and cooled on ice, followed by centrifugation at 10,000 × g for 15 min. Optical densities of the supernatants were recorded at 532 and 600 nm.
The method of Wild et al. (Citation2012) was employed for the determination of MG content. The absorbance was recorded at 288 nm. MG content was calculated using a standard curve of known MG concentrations.
2.10. Antioxidant enzymes
2.10.1. Extraction of enzymes
Extraction was carried out by grinding 500 mg fresh leaves in 50-mM sodium phosphate buffer (pH 7.0) containing 1% polyvinyl pyrrolidone. The homogenate was centrifuged at 10,000 × g for 15 min at 4°C, and the supernatant was used as an enzyme source. For extraction of ascorbate peroxidase (APX), the buffer was supplemented with 2.0-mM ascorbate. The method of Bradford (Citation1976) was employed for protein estimation by using bovine serum albumin as a standard.
2.10.2. Enzyme assay
Superoxide dismutase: activity of SOD (EC1.15.1.1) was measured following the photoreduction of nitroblue tetrazolium at 560 nm. The amount of protein able to cause 50% decline in SOD-inhabitable Nitro Blue Tetrazolium Chloride reduction was considered as one unit of enzyme activity. The activity was expressed as unit mg−1 protein (van Rossum et al. Citation1997). The method of Luck (Citation1971) was adopted to assay catalase (CAT, EC 1.11.1.6) activity and change in the absorbance was monitored at 240 nm. CAT activity was expressed as EU mg−1 protein. The assay of APX (EC 1.11.1.11) activity was used for measuring the change in the absorbance at 290 mm for 3 min and the activity was expressed as EU mg−1 protein (Nakano and Asada Citation1981).
For estimation of glutathione reductase (GR, EC 1.6.4.2) activity, the method of Foyer and Halliwell (Citation1976) was followed. The change in optical density was measured at 340 nm, and the activity was expressed in μmol nicotinamide adenine dinucleotide phosphate (NADPH) oxidized/unit mg−1 protein. The activity of monodehydroascorbate reductase (MDHAR, EC 1.6.5.4) was assayed following the method described by Miyake and Asada (Citation1992), the change in the absorbance recorded at 340 nm for 3 min, and expressed as μmol NADPH oxidized/unit mg−1 protein. Dehydroascorbate reductase (DHAR, EC: 1.8.5.1) activity was assayed using the method suggested by Nakano and Asada (Citation1981). The change in optical density was measured at 265 nm and the activity was expressed as Units mg−1 protein.
For estimation of guaiacol peroxidase (GPX, EC: 1.11.1.9) activity, the method of Elia et al. (Citation2003) was employed. The change in the absorbance was monitored at 340 nm for 2 min, and the activity was expressed as EU mg−1 protein. The activity of glutathione S-transferase (GST, EC: 2.5.1.18) was determined following the procedure described by Hasanuzzaman and Fujita (Citation2013). The change in the absorbance was recorded at 340 nm for 2 min, and the activity was expressed as EU mg−1 protein.
2.11. Estimation of ascorbic acid (AsA), glutathione, and α-tocopherol
For estimation of AsA and reduced GSH, 500 mg fresh leaf tissues were extracted in 5% meta-phosphoric acid and 1 mM EDTA followed by centrifugation at 11,500 × g for 15 min at 4°C. The supernatant was collected and estimation of AsA and GSH was carried out following the methods described by Huang et al. (Citation2005) and Yu et al. (Citation2003), respectively. Standard curves with known concentrations of AsA and GSH were used. The content of GSH was calculated by subtracting GSSG (oxidized glutathione) from total GSH. The method of Baker et al. (Citation1980) was followed for determination of α-tocopherol, and the absorbance was recorded at 520 nm. Calculations were done using a standard curve of α-tocopherol.
2.12. Estimation of glyoxalase I (Gly I) and glyoxalase II (Gly II)
The method of Hossain et al. (Citation2009) was used for the determination of activity of Gly I (EC 4.4.1.5) using an extinction co-efficient of 3.37 mM−1 cm−1.
The activity of Gly II (EC 3.1.2.6) was estimated according to the method of Mostofa and Fujita (Citation2013) by employing an extinction co-efficient of 13.6 mM−1 cm−1.
2.13. Estimation of flavonoids
Flavonoid contents were estimated following the method of Zhishen et al. (Citation1999) using catechin as standard. Optical density was recorded at 510 nm and expressed as mg catechin equivalents g−1 of extract.
2.14. Elemental analysis
Dried leaf samples (100 mg) were digested in an appropriate acid mixture and the content of mineral elements, Na, K, and Ca were estimated following the method of Wolf (Citation1982) by a flame photometer. The plant material (0.5 g) were dried in oven and then digested in nitric acid and sulfuric acid mixture (5:1, v/v) at 60°C for 24 h. The digested material was treated with HNO3/HClO4 (5/1, v/v). Atomic absorption spectrophotometer (Perkin-Elmer, Analyst Model 300) was used for the estimation of Zn.
2.15. Statistical analysis
The data presented is means of five replicates. Shapiro-Wilk’s test (P > .05) for normality and parametric Levene’s test (P > .05) for data homogeneity assumption were met. Standard analysis of variance for parametric data was employed for all statistical analyses, and least significant differences were calculated at P < .05 following the Duncan test in SPSS program.
3. Results
3.1. Growth and biomass yield
The results related to effects of NaCl, Zn, and Ca on growth and biomass yield are presented in . Shoot and root lengths decreased by 39.68% and 39.85%, respectively, with 200 mM NaCl treatment. Application of Zn enhanced the shoot length by 16.79% and root length by 21.96%. Salt (NaCl)-treated plants supplemented with Ca also enhanced shoot length by 26.26% and root length by 25.13%. Zinc + Ca in combination showed 44.87% and 36.20% increase in shoot and root length, respectively, compared to that of NaCl-treated plants. The combined application of Ca and Zn was found to be more beneficial than when Ca and Zn applied individually. The shoot DW also decreased by 52.47% with 200 mM NaCl treatment. However, shoot DW increased with NaCl + Zn + Ca by 28.15%. When Zn and Ca were applied individually an increase in shoot DW by 14.62% with NaCl + Zn and 22.30% with NaCl + Ca treatments compared with NaCl-treated plants were observed. The control plants supplemented with Zn and Ca also showed an increase in DW and the maximum increase of 19.20% was recorded with NaCl + Zn + Ca treatment compared to when Zn and Ca were applied individually ().
Table 1. Effect of Zn and Ca on growth and biomass yield in mustard seedlings under NaCl stress. Data presented are the means ± SE (n = 5). Means following different letters differ significantly at P ≤ .05.
3.2. Yield parameters
The results related to the effect of NaCl, Zn, and Ca in mustard seedlings are depicted in . NaCl stress decreased silique per plant, seeds per silique, 1000 seeds weight and seed yield by 43.45%, 45.29%, 33.44%, and 32.73%, respectively as compared to control. However, supplementation of Zn and Ca individually enhanced the upper yield parameters to appreciable level. But combination of Zn + Ca proved to be more beneficial as it enhanced the silique per plant by 49.37%, seeds per silique by 51.82%, 1000 seeds weight by 22.70% and seed yield by 29.68% over the plants treated with NaCl alone.
Table 2. Effect of Zn and Ca on yield attributes in mustard seedlings under NaCl stress. Data presented are the means ± SE (n = 5). Means following different letters differ significantly at P ≤ .05.
3.3. Oil content
NaCl stress decreased the oil content of seeds by 23.32% over the control plants (). However, minimum decline in oil content was recorded by the application of Zn (18.75%), Ca (17.15%), and Zn + Ca (14.66%) to NaCl-stressed seedlings in respect to control. Control seedlings treated with Zn and Ca showed insignificant increase in oil content.
3.4. Leaf pigments
The leaf total chlorophyll (Chl) content declined by 44.65% with 200 mM NaCl treatment (). Increases in total Chl content recorded were 12.41% with Zn application, 16.55% with Ca, and 24.82% with Zn + Ca relative to that of the plants irrigated with NaCl. The concentration of carotenoids was reduced by 42.55% with NaCl stress relative to the control treatment (). However, with the application of Zn and Ca, carotenoid content increased and the maximum increase of 44.44% was recorded with NaCl + Zn + Ca over the plants treated with NaCl only.
Table 3. Ameliorating role of Zn and Ca on pigment content, gas exchange attributes, leaf relative water content (LRWC), electrolyte leakage, and proline content in mustard seedlings under NaCl stress. Data presented are the means ± SE (n = 5). Means following different letters differ significantly at P ≤ .05.
3.5. CO2 assimilation, transpiration rate, and stomatal conductance
CO2 assimilation rate (A) and transpiration rate (E) decreased by 45.60% and 35.58%, respectively, with NaCl treatment (). Salt-treated plants supplemented with Zn and Ca showed an increase in the above-mentioned parameters. Maximum increases of 23.17% in A and 22.09% in E were observed with NaCl + Zn + Ca treatment compared to those of the plants treated with only NaCl. Stomatal conductance (gs) increased by 42.10% with NaCl stress. However, combined application of Zn + Ca decreased gs to 20.37% relative to that of the NaCl-treated plants.
3.6. LRWC, electrolyte leakage and proline content
Salt (200 mM) brought down the LRWC from 90.12% to 57.62% () in the present study. Salt-treated plants supplemented with Zn and Ca enhanced the LRWC by 72.31%, 73.15%, and 78.46% with NaCl + Zn, NaCl + Ca, and NaCl + Zn + Ca, respectively, compared to that of the NaCl-treated plants. Electrolyte leakage also increased to 74.71% with 200 mM NaCl stress. Supplementation of Zn and Ca individually or in combination (Zn + Ca) decreased the electrolyte leakage by 66.91%, 58.12%, and 46.52%, respectively, relative to that of the plants treated with NaCl (). Proline accumulation in leaf tissues enhanced by 2.47-fold with 200 mM NaCl treatment. Further accumulations of proline, 2.95-fold, 3.08-fold, and 3.39-fold were recorded for NaCl + Zn, NaCl + Ca, and NaCl + Zn + Ca, respectively, compared to that of the NaCl-treated plants ().
3.7. Hydrogen peroxide, MDA, and MG content
Salt increased the production of H2O2 causing considerable increase in MDA content, which however declined with Zn and Ca application ((A)). Salt increased the accumulation of H2O2 and MDA by 3.00-fold and 1.51-fold, respectively, under 200 mM NaCl. Application of Zn and Ca decreased the H2O2 and MDA contents, and maximum decreases of 1.38-fold in H2O2 and 1.16-fold in MDA were recorded with NaCl + Zn + Ca relative to that of the control plants. Salt stress elevated the MG content by 179.13% as compared to control, however, decline in MG was observed by the supplementation of Zn (11.72%), Ca (32.47%), and Zn + Ca (46.02%) in NaCl-stressed seedlings compared to the NaCl-alone treated seedlings ((B)).
3.8. Enzymatic antioxidants and AsA-Glu cycle
Salt-treated plants increased the SOD content by 25.83%. However, further increases of 10.58%, 25.83%, and 23.17% were recorded with the application of NaCl + Zn, NaCl + Ca, and NaCl + Zn + Ca, respectively, compared to that under NaCl treatment (). Catalase (CAT) activity decreased by 18.06% with NaCl stress. However, application of Zn and Ca enhanced the CAT activity by 4.72% with NaCl + Zn, 5.51% with NaCl + Ca, and 17.32% with NaCl + Zn + Ca compared to that in the NaCl-treated plants (). The APX activity increased by 45.11% with NaCl treatment. Individual addition of Zn or Ca increased the APX activity by 7.35% and 10.41%, respectively. A maximal APX enhancement of 30.32% was recorded with the combined application of NaCl + Zn + Ca relative to NaCl-treated plants (). The GR activity increased by 24.95% with 200 mM NaCl stress relative to that in plants exposed to the control treatment. Salt-treated plants supplied with Zn and Ca showed further increases of 18.89%, 17.97%, and 27.34% with NaCl + Zn, NaCl + Ca, and NaCl + Zn + Ca, respectively, over that in the NaCl-treated plants ().
Table 4. Effect of Zn and Ca on SOD, CAT, APX, GR, MDHAR, DHAR, GPX, GST, AsA, GSH, and tocopherol under NaCl stress. Data presented are the means ± SE (n = 5). Means following different letters differ significantly at P ≤ .05.
MDHAR and DHAR decreased by 41.50% and 49.07%, respectively, with NaCl application compared to those in the control plants (). Supplementation of Zn and Ca enhanced the activities of MDHAR and DHAR in the present study and maximum increases of 69.38% and 110.27% were recorded with NaCl + Zn + Ca treatment over that in the NaCl-treated plants alone. Control plants treated with Zn + Ca showed increases of 11.34% in MDHAR and 6.66% in DHAR relative to that in the control plants.
The GPX activity increased by 53.33% with NaCl stress, and further increases of 8.69%, 8.69%, and 34.78% were observed with treatments NaCl + Zn, NaCl + Ca, and NaCl + Zn + Ca, respectively, relative to that in the NaCl-treated plants. The control plants treated with Zn + Ca also showed a 13.33% increase in the GPX activity ().
Salt (200 mM)-treated plants showed an increase of 88.24% in the GST activity. Relative to that in the NaCl-treated plants, application of Zn and Ca further enhanced the GST activity by 14.07%, 16.72%, and 55.34% with NaCl + Zn, NaCl + Ca, and NaCl + Zn + Ca, respectively. An increase of 20.50% in the GST activity was also recorded when control plants were treated with Zn + Ca alone ().
AsA content decreased by 45.83% with NaCl treatment. However, increases of 10.34%, 13.33%, and 18.75% in AsA were observed with NaCl + Zn, NaCl + Ca, and NaCl + Zn + Ca, respectively, in comparison with that in the NaCl-treated plants (). Glutathione (GSH) increased by 73.04% with 200 mM NaCl treatment (). Salt-treated plants supplied with Zn and Ca showed a further enhancement of 12.56%, 14.57%, and 37.68% with NaCl + Zn, NaCl + Ca, and NaCl + Zn + Ca, respectively, over that of the NaCl-treated plants. The control plants treated with Zn + Ca showed 10.43% increase in GSH relative to that of the control plants. Accumulation of tocopherol of 6.97-fold was recorded with 200 mM NaCl treatment (). Relative to that in the NaCl-stressed plants, further increases of 8.56-fold, 8.96-fold, and 9.92-fold were observed with NaCl + Zn, NaCl + Ca, and NaCl + Zn + Ca treatments, respectively. An increase of 1.24-fold was registered at application of Zn + Ca.
3.9. Gly I and Gly II
Gly I activity enhanced by 67.74% in NaCl-stressed seedlings as compared with control. Further increase by 17.30%, 26.92%, and 38.40% over NaCl-alone treated seedlings was recorded by the supplementation of Zn, Ca, and Zn + Ca, respectively, to NaCl-treated seedlings ((A)).
Figure 3. Effect of Zn and Ca on (A) Gly I and Gly II activity and (B) flavonoid content in mustard seedlings under NaCl stress. Data presented are the means ± SE (n = 5). Means following different letters differ significantly at P ≤ .05.
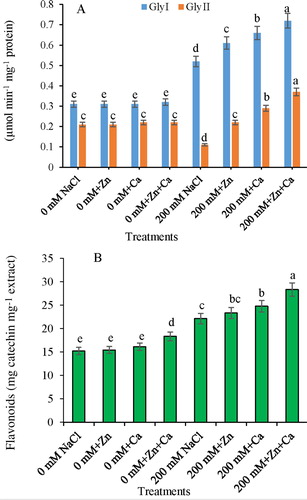
Gly II decreased by 47.61% with NaCl stress over control. Application of Zn, Ca, and Zn + Ca enhanced the Gly II by 100%, 163.63%, and 236.36%, respectively, over the NaCl-treated seedlings alone ((A)).
3.10. Flavonoids
The control plants supplemented with Zn + Ca exhibited an increase of 20.44% in flavonoid content over the control plants supplied with no Zn or Ca. Salt (200 mM) application increased the flavonoid content by 45.36%, and further increases of 53.32%, 62.85%, and 86.19% with NaCl + Zn, NaCl + Ca, and NaCl + Zn + Ca treatments, respectively, were recorded over that of the control plants ((B)).
3.11. Nutrient uptake
Salt (200 mM NaCl)-treated plants showed decreased accumulation of Zn by 48.20% (). Application of Zn and Ca enhanced the accumulation of these nutrients in plant tissues by 475%, 14.93%, and 520% with NaCl + Zn, NaCl + Ca, and NaCl + Zn + Ca treatments, respectively, over that of the control plants. Accumulation of Ca and K also decreased by 75.38% and 53.69%, respectively, with NaCl stress. However, NaCl-treated plants supplied with Zn and Ca showed enhanced accumulation of these nutrients, and maximum accumulation recorded was 232% of Ca and 62.74% of K with NaCl + Zn + Ca treatment over NaCl-treated plants. The control plants supplied with Zn + Ca showed 21.06% and 3.89% increases in Ca and K accumulation, respectively, relative to that in the control plants. Sodium (Na+) accumulation increased by 468% with 200 mM NaCl treatment. However, application of Zn and Ca decreased Na+ accumulation by 37.99%, 48.38%, and 61.62% with NaCl + Zn, NaCl + Ca, and NaCl + Zn + Ca treatments, respectively, compared to that in the NaCl-treated plants (). Na/K ratio also increased by 12.80-fold under 200 mM NaCl stress. Salt-treated plants supplied with Zn and Ca showed 6.93-fold, 5.20-fold, and 3.00-fold with NaCl + Zn, NaCl + Ca, and NaCl + Zn + Ca treatments, respectively, relative to that of the control plants ().
Table 5. Effect of Zn and Ca on Na, Zn, Ca, K, and Na/K ratio under NaCl stress. Data presented are the means ± SE (n = 5). Means following different letters differ significantly at P ≤ .05.
4. Discussion
In the present study, salt stress (200 mM) caused a considerable retardation in plant growth, reducing plant height, root length, and shoot DW in Brassica juncea plants. However, foliar spray of Zn or Ca or Zn and Ca decreased the impact of NaCl on these parameters. Weisany et al. (Citation2014) and Ahmad et al. (Citation2015) have also reported the growth promoting effect of Ca, likely due to its significant influence on the uptake and partitioning of important mineral elements such as magnesium and potassium. Zinc is believed to regulate growth as it is essential for the formation of natural auxin indole-3-acetic acid, thereby controlling cell division and cellular expansion (Ali and Mahmoud Citation2013). Besides this, Zn is involved in the maintenance of structural integrity of biomembranes (Weisany et al. Citation2012), accumulation of phospholipids, up-regulation of protein synthesis, and the free radical scavenging (Jiang et al. Citation2014). Rockenfeller and Madeo (Citation2008) have suggested that Zn mediates nutrient translocation towards developing cells with subsequent reduction in the uptake of toxic ions such as Na+ and Cl− (Ibrahim and Faryal Citation2014, Jiang et al. Citation2014). On the other hand, Ca is an active regulator of several cellular functions such as cell division and elongation, the cytoplasmic streaming, photomorphogenesis, and, more importantly, has been reported to protect plants against environmental stresses (Kader and Lindberg Citation2010, Sarwat et al. Citation2013, Ahmad et al. Citation2015, Ahmad et al. Citation2016b). Calcium in interaction with calmodulin regulates key functions such as gene regulation, ion transport, cell motility, growth, proliferation, apoptosis, and stress tolerance (Yang and Poovaiah Citation2003, DalCorso et al. Citation2010). It is evident that salt stress leads to improved accumulation of Na and significant reduction in the uptake of other mineral ions like K, leading to increased Na/K ratio. In the present study, Ca and Zn supplemented plants not only responded by exhibiting their increased accumulation but also enhanced the uptake of essential ion K, thereby reducing the Na/K ratio. Such effects were much obvious when Ca and Zn were supplemented in combination compared to their individual treatments. Improved K uptake coupled with reduced uptake of Na led to ion homeostasis in Zn- and Ca-treated mustard plants. Rubio et al. (Citation2003) have reported that Ca reduces the negative impact of salinity stress by restricting the uptake and transport of Na and its binding to cell wall. Improved Ca and Zn uptake was associated with enhanced K uptake in the mustard plants. Such finding supports their involvement in maintaining the hydraulic conductance, tissue water balance, and the membrane functioning leading to improved growth under NaCl treatments (Ahanger and Agarwal Citation2016). It has been reported that enhanced NaCl boosts the mobility of Zn (Acosta et al. Citation2011; Novo et al. Citation2014) making it available to roots in abundance under stress. Calcium and Zn supplemented plants in the present study maintained lower Na/K ratio depicting the much selective absorption of Na at the root plasma membrane level. Earlier, Arshi et al. (Citation2010) also observed a significant reduction in Na/K in salt-stressed Glycine max as a result of Ca supplementation and it has been suggested that calcium and potassium show much similarity in behavior during the selective transport of ions across the membranes (Boursier and Läuchli Citation1990).
Decreases in yield attributes by NaCl stress in the present study corroborates with the findings of Kumar and Khare (Citation2016) in rice. Size of grain and its shape is very important as it determines its quality. Rao et al. (Citation2013) observed negative impact of salt stress on grain length and thickness and Lutts et al. (Citation1995) reported the loss of grain weight due to salinity. Salinity stress decreases the photosynthetic rate thus decreases the crop yield (Rubio et al. Citation2003). Another main reason of decrease of the yield is due to the salinity-induced nutrient deficiency in plants (Ahmad et al. Citation2016b). Application of Zn enhanced the yield attributes and the results are in coherence with Ma et al. (Citation2017) in wheat. Grain yield was enhanced by the supplementation of Zn was also reported by Karim et al. (Citation2012) in wheat seedlings under drought stress. The enhancement in grain yield may be due to the reason that plants are able to absorb more Zn through soil or leaf when given externally (Ma et al. Citation2017). External supplementation of Ca enhances the yield in the present study may be because it helps in uptake of nutrients (Ahmad et al. Citation2015, Ahmad et al. Citation2016a).
The oil yield content is also decreasing by NaCl stress in mustard seedlings. Similar results were observed in our previous study with Cd toxicity (Ahmad et al. Citation2015). The decrease in oil content may be due to NaCl inhibits the fatty acid biosynthesis. Another reason may be retarded seed development due to salinity (Ashraf and Tufail Citation1995, Boem et al. Citation1997, Akhtar et al. Citation2002). The supplementation of Zn and Ca enhanced the oil yield content in the present study and the reason might be Zn and Ca enhanced the uptake of mineral elements, Zn is also a part of the antioxidant enzyme SOD (ZnSOD) thus enhances the activity of quenching the ROS.
Chlorophyll content and the related photosynthetic characteristics including stomatal conductance and internal CO2 concentration in Brassica juncea leaves subjected to saline condition considerably declined. Such results have also been reported in mustard plant (Fatma et al. Citation2014, Iqbal et al. Citation2015). It is widely known that higher NaCl concentration has the potential to hamper the synthesis of enzymes mediating the synthesis and protection of photosynthetic pigments (El-Tayeb Citation2005, Fatma et al. Citation2014) and, more importantly, it impedes the uptake of ions like Mg that forms the central core of the chlorophyll molecule. It is believed that higher salt concentrations cause destruction in the structure and stability of pigment-protein complex, with significant increases in the chlorophyllase activity leading to chlorophyll degradation (Fang et al. Citation1998). Calcium- and Zn-mediated improvement in the photosynthetic pigments and photosynthetic attributes as observed in the present study seems to have a significant role in improving growth of B. juncea plants under stress conditions. Xu et al. (Citation2013) reported mitigation of drought-induced reduction in stomatal conductance, chlorophyll fluorescence, and net photosynthetic rate in Zoysia japonica due to foliar application of Ca. Ahmad et al. (Citation2015) and Rahman et al. (Citation2016) have also demonstrated improved pigment synthesis due to Ca supplementation in mustard and rice respectively. Our results showing an increase in chlorophyll after Zn application support the findings of Samreen et al. (Citation2017) for Vigna radiata. Zinc is known to prevent salt stress-induced drastic decline in chlorophyll likely because of its protective role for the sulfhydryl group (Weisany et al. Citation2011). Enhanced protection to photosynthetic attributes after Ca and Zn application may have been partly due to improved accumulation of osmolytes such as proline, glycine, and betaine (Iqbal et al. Citation2015) or improvement in the uptake of ions, such as K, which significantly contribute towards the maintenance of tissue water potential.
Brassica juncea plants supplemented with Zn and Ca exhibited relatively higher accumulation of proline under normal and stressed conditions, causing improvement in the osmotic strength of plants leading to significant increases in water uptake by plants and hence to photosynthetic efficiency. In barley and Brassica juncea, Unal et al. (Citation2014) and Iqbal et al. (Citation2015), respectively, also demonstrated increased proline accumulation after NaCl treatment. It is possible that Zn and Ca have regulated proline accumulation through their cumulative contribution in gene expression and amino acid degradation and, hence, to metabolism of proline resulting in greater stress tolerance. Proline is known to provide protection to enzyme activity and can bring neutralization of free radicals (Hare and Cress Citation1997). Improved accumulation of proline due to Zn and Ca application may cause improvement in uptake of water from the soil by regulating the solute potential. Many studies have observed that crop species accumulating greater contents of osmolytes show improved growth under stress environments (Kawakami et al. Citation2008, Ahanger et al. Citation2015, Ahanger and Agarwal Citation2016, Ahanger and Agarwal Citation2017). Increased osmolyte accumulation imparts tolerance to salt stress thereby protecting major metabolic structures and pathways by reducing the ROS formation and the ROS triggered damage (Ahanger et al. Citation2015, Ahmad et al. Citation2015).
Increase in the production of ROS is the key response of plants to stressful environments. In the present study, salinity triggered over-accumulation of H2O2 in B. juncea, which, however, was reduced by the application of Ca and Zn under both growth conditions. When H2O2 productivity is kept within certain threshold levels, it can lead to protection of plants by mediating the signaling events for quick triggering of tolerance mechanisms. Nevertheless, NaCl-induced overproduction of ROS, which causes peroxidation of membrane fatty acids, protein denaturation, and can also harm DNA ultrastructure (Tuteja et al. Citation2009). Increased accumulation of H2O2 in NaCl-stressed B. juncea leaf tissues may be probably due to reduced RWC that limited the diffusion of H2O2 from its generation site (Weisany et al. Citation2012). Salt-induced upsurge in H2O2 production may have imparted direct effects on the peroxidation rates of membrane lipids leading to membrane damage and leakage of cellular constituents. Our observations are in line with those of Arshi et al. (Citation2010) and Ahmad et al. (Citation2015) who also demonstrated reduced membrane damage in Ca supplemented mustard plants. Stress-mediated production of H2O2, and subsequent membrane leakage and MDA formation determine the intensity of oxidative stress (Tavallali et al. Citation2009, Ahmad et al. Citation2015), and its subsequent reduction in Ca and Zn supplemented plants depicts their potential to prevent oxidative damage under NaCl stress. Transient increase in ROS production during stressful conditions causes severe alterations in membrane fatty acid composition and pose stern effects on their functioning and of the cell overall (Abd_Allah et al. Citation2015). During the exposure to stress, protein degradation is enhanced, which is often evident under increased protease activity but is reduced due to optimal supplementation of mineral elements (Ahanger and Agarwal Citation2016). Many researchers have advocated strong correlation between ROS production and the structural and functional integrity of membranes (Tavallali et al. Citation2009, Ahmad et al. Citation2010, Arshi et al. Citation2010, Fatma et al. Citation2014, Ahmad et al. Citation2015, Iqbal et al. Citation2015). Reduction in lipid peroxidation, reflecting increased membrane stability in Ca and Zn supplemented plants, justifies their positive role in amelioration of the ROS-induced oxidative damage, thereby protecting the important molecules under salinity stress.
Several research reports have advocated the increased production of ROS as the more often elicited response to the stresses. Evolution of defense mechanisms, existing in different cellular compartments, work in close coordination to cause neutralization of ROS beyond the critical threshold levels. In this study, supplementation of Zn and Ca resulted in up-regulation of antioxidant system by improving the activity of enzymes with concomitant improvement in the content of non-enzymatic components thereby mediating the quick elimination of ROS. Simultaneous and abrupt increase in the generation rate of ROS leads to activation of the defense system for counteracting their subsequent negative effects. Earlier studies conducted by Ahanger and Agarwal (Citation2016), Ahmad et al. (Citation2016b), Ahmad et al. (Citation2015), Iqbal et al. (Citation2015), also supported the significant role of up-regulated antioxidant metabolism in growth maintenance and stress tolerance of plants. The present investigation also confirms an increase in activities of antioxidant enzymes studied, except catalase that showed a decreasing trend with increasing salt concentration. In the present study, salt stress up-regulated the activities of antioxidant enzymes, which were further enhanced due to supplementation of Zn and Ca, thereby mediating better ROS neutralization. Supplementation of optimal mineral concentrations has been reported to keep ROS under non-toxic levels (Iqbal et al. Citation2015, Ahanger and Agarwal Citation2016). Among these defensive enzymes, SOD functions as the frontline protector against superoxide radicals which are further scavenged either by catalases (CATs) or APX through ascorbate-glutathione pathway, resulting in its removal which avoids the subsequent more toxic radical formation (Ahmad et al. Citation2010, Bose et al. Citation2013). Our results of improved antioxidant metabolism due to Ca supplementation corroborate the findings of Arshi et al. (Citation2010) and Dolatabadian et al. (Citation2013). Exogenous application of Ca to Festuca arundinacea and Poa pratensis resulted in mitigation of heat stress by improving SOD activity (Jiang and Huang Citation2001). Zinc is reported to form the cofactor of SOD and it prevents the metal-mediated formation of toxic OH− radical. APX, GR, MDHAR, DHAR, GSH, and AsA are the key members of ascorbate-glutathione pathway. Siddiqui et al. (Citation2015) also demonstrated increased activity of different antioxidant enzymes in Vicia faba resulting in improved cadmium tolerance.
Up-regulated activities of APX and GR and production of GSH in this study could be related to better stress tolerance in B. juncea seedlings through maintaining the redox homeostasis of cells, in addition to better ROS scavenging. APX, GR, GSH, and AsA in ascorbate-glutathione pathway mediate removal of H2O2 by transferring electrons from NADPH to H2O2, utilizing GSH and AsA as mobile redox buffers. GSH and AsA are involved in many cellular processes, including their key roles in plant tolerance and in activation of enzymes involved in plant growth and development at different ontogenic stages (Hajiboland Citation2014, Fatma et al. Citation2014). The findings of the present study suggest that Zn and Ca application caused an increase in GSH concentration, which might have contributed to efficient H2O2 scavenging, lowering lipid peroxidation through increased activity of GR and maintaining the optimal concentrations of GSH. Under stressed conditions, Iqbal et al. (Citation2015) observed that increased GR activity and GSH content in wheat helped to protect photosynthetic apparatus from ROS. Improved GR activity in Ca and Zn supplemented B. juncea plants led to optimization of MDHAR and DHAR activities by way of enhanced GSH production. GST helps in the detoxification of herbicides and neutralization of toxic xenobiotics through their conjugation with the non-enzymatic antioxidant, the tripeptide glutathione (Ahmad et al. Citation2010). In addition to the obvious role in detoxification of radicals like hydroperoxides, maintaining higher activity of GST also causes hormone homeostasis, vacuolar sequestration of anthocyanins, cell apoptosis, and stress responses (Dixon et al. Citation2010). Our results of increased activity of GST in salt-stressed B. juncea concur with the findings of Gapińska et al. (Citation2007) for Lycopersicon esculentum. Our results that Ca and Zn supplementation induced improvement in antioxidant metabolism, suggest that these mineral elements can be used as effective tools to protect photosynthesis from the injurious effects of ROS.
The production of MG is eliminated by the coordinated action of Gly I, Gly II, and GSH (Racker Citation1951). Gly I activity has been reported to upregulate in response to different abiotic stresses (Veena and Sopory. Citation1999). Espartero et al. (Citation1995) also reported enhanced Gly I activity in tomato under NaCl stress. In glyoxalase system, MG reacts with GSH and produces hemithioacetal which is then converted to S-D-lactoylglutathione (SLG) catalyzed by Gly I enzyme. SLG is then converted to D-lactate by hydrolysis catalyzed by Gly II and later GSH regenerates (Kaur et al. Citation2014). Thus overexpression of glyoxalase system will enhance the salt tolerance (Kaur et al. Citation2014). Reddy and Sopory (Citation1999) reported that overexpression of Gly I gene decreased the endogenous MG and enhanced the tolerance against MG and salt stress. Singla-Pareek et al. (Citation2007) reported that overexpression of Gly II gene in rice enhances tolerance to MG and salt stress. Enhanced activities of Gly I and Gly II reduces MG levels under different abiotic stresses (Rahman et al. Citation2015a, Nahar et al. Citation2016). Supplementation of Ca further enhanced the Gly I and Gly II activity and the results corroborates with the findings of Rahman et al. (Citation2016). External supplementation of Ca stimulates glyoxalase enzyme activity and reduces MG content (Rahman et al. Citation2015a, Rahman et al. Citation2015b).
AsA and GSH function as a major redox buffer. AsA also functions as a cofactor for many enzymes involved in regulation of phytohormone metabolism, photosynthesis, regeneration of antioxidants, cellular division, and hence growth (Athar et al. Citation2008, Gallie Citation2013). In addition, non-enzymatic antioxidants protect cells and the organelles from the ROS-induced oxidative damage (Conklin and Barth Citation2004). Flavonoids and other secondary metabolite compounds, pigments such as anthocyanins, AsA, and GSH get accumulated in plants following stress exposure and impart an array of protective functions (Brunetti et al. Citation2013, Brunetti et al. Citation2015). Calcium and Zn supplementation provoked the synthesis of flavonoids in B. juncea plants under normal as well as NaCl-treated conditions thereby preventing the damage to major cellular functions including membrane structure. Flavonoids are the bioactive compounds that provide protection to the photosynthesizing cells and have the potential to protect plants from the highly energetic UV radiations, thereby inhibiting the ROS generation and can also mediate their quenching (Brunetti et al. Citation2013). It has been observed that improved photosynthetic efficiency is due to flavonoid induced quick scavenging of superoxide radicals (Majer et al. Citation2014). Plants exposed to stress conditions accumulate higher contents of polyphenols for promoting defensive mechanisms, and supplementation of mineral elements has been reported to promote their synthesis for maintaining the optimal cellular functioning (AbdElgawad et al. Citation2016). Optimal supplementation of mineral elements optimizes the accumulation of polyphenols reflecting growth maintenance as observed in Avena sativa (Ahanger et al. Citation2015) and Triticum aestivum (Ahanger and Agarwal Citation2016). Nevertheless, flavonoids regulate plant metabolism and are believed to be involved in signaling mechanisms by way of the interactions with a wide range of protein kinases like mitogen-activated protein kinases reflecting the growth and cellular differentiation initiation (Brunetti et al. Citation2013). Our results support the optimal application of Ca and Zn for enhancing the NaCl-stress tolerance through their involvement in the synthesis of flavonoids. It has been proposed that flavonoids act as chelators under salt stress (Winkel-Shirley Citation2002). Thus, improved antioxidant metabolism and glyoxalase system through supplementation of Ca and Zn may have contributed to the redox balance thereby exerting a positive influence on the growth performance of B. juncea under salt stress.
5. Conclusion
Calcium (Ca) and Zn supplementation significantly reversed the NaCl-induced oxidative damage by up-regulating the antioxidant metabolism and glyoxalase system. Our results strongly support the positive interactive role of Ca and Zn in averting the NaCl stress that led to deleterious changes in B. juncea. Improved antioxidant metabolism and glyoxalase system, reduced Na/K ratio and redox homeostasis due to Ca and Zn supplementation confers protection of cellular functioning under salt-induced oxidative stress.
Disclosure statement
No potential conflict of interest was reported by the authors.
Additional information
Funding
References
- Abd_Allah EF, Hashem A, Alqarawi AA, Bahkali AH, Alwhibi MS. 2015. Enhancing growth performance and systemic acquired resistance of medicinal plant Sesbania sesban (L.) Merr using arbuscular mycorrhizal fungi under salt stress. Saudi J Biol Sci. 22:274–283. doi: 10.1016/j.sjbs.2015.03.004
- AbdElgawad H, Zinta G, Hegab MM, Pandey R, Asard H, Abuelsoud W. 2016. High salinity induces different oxidative stress and antioxidant responses in maize seedlings organs. Front Plant Sci. 7:276.
- Acosta JA, Jansen B, Kalbitz K, Faz A, Martínez-Martínez S. 2011. Salinity increases mobility of heavy metals in soils. Chemosphere. 85:1318–1324. doi: 10.1016/j.chemosphere.2011.07.046
- Ahanger M, Agarwal R. 2016. Potassium up-regulates antioxidant metabolism and alleviates growth inhibition under water and osmotic stress in wheat (Triticum aestivum L.). Protoplasma. 254:1471–1486. doi: 10.1007/s00709-016-1037-0
- Ahanger MA, Agarwal RM. 2017. Salinity stress induced alterations in antioxidant metabolism and nitrogen assimilation in wheat (Triticum aestivum L) as influenced by potassium supplementation. Plant Physiol Biochem. 115:449–460. doi: 10.1016/j.plaphy.2017.04.017
- Ahanger MA, Agarwal R, Tomar NS, Shrivastava M. 2015. Potassium induces positive changes in nitrogen metabolism and antioxidant system of oat (Avena sativa L cultivar Kent). J Plant Interact. 10:211–223. doi: 10.1080/17429145.2015.1056260
- Ahmad P, Abdel Latef AA, Abd_Allah EF, Hashem A, Sarwat M, Anjum NA, Gucel S. 2016a. Calcium and potassium supplementation enhanced growth, osmolyte secondary metabolite production, and enzymatic antioxidant machinery in cadmium-exposed chickpea (Cicer arietinum L.). Front Plant Sci. 7:513.
- Ahmad P, Abdel Latef AA, Hashem A, Abd Allah EF, Gucel S, Tran LS. 2016b. Nitric oxide mitigates salt stress by regulating levels of osmolytes and antioxidant enzymes in chickpea. Front Plant Sci. 7:347.
- Ahmad P, Jaleel CA, Salem MA, Nabi G, Sharma S. 2010. Roles of enzymatic and nonenzymatic antioxidants in plants during abiotic stress. Crit Rev Biotechnol. 30:161–175. doi: 10.3109/07388550903524243
- Ahmad P, Sarwat M, Bhat NA, Wani MR, Kazi AG, Tran L-SP. 2015. Alleviation of cadmium toxicity in Brassica juncea L.(Czern. & Coss.) by calcium application involves various physiological and biochemical strategies. PLoS One. 10:e0114571. doi: 10.1371/journal.pone.0114571
- Akhtar J, Saqib M, Mahmood K. 2002. Effect of salinity on yield, growth and oil contents of four Brassica species. Pak J Agri Sci. 39:76–79.
- Ali E, Mahmoud AM. 2013. Effect of foliar spray by different salicylic acid and zinc concentrations on seed yield and yield components of mungbean in sandy soil. Asian J Crop Sci. 5:33. doi: 10.3923/ajcs.2013.33.40
- Aravind P, Prasad M. 2004. Zinc protects chloroplasts and associated photochemical functions in cadmium exposed Ceratophyllum demersum L., a freshwater macrophyte. Plant Sci. 166:1321–1327. doi: 10.1016/j.plantsci.2004.01.011
- Arshi A, Ahmad A, Aref I, Iqbal M. 2010. Calcium interaction with salinity-induced effects on growth and metabolism of soybean (Glycine max L.) cultivars. J Environ Biol. 31:795–801.
- Ashraf M, McNeilly T. 2004. Salinity tolerance in Brassica oilseeds. Crit Rev Plant Sci. 23:157–174. doi: 10.1080/07352680490433286
- Ashraf M, Tufail M. 1995. Variation in salinity tolerance in sunflower (Helianthus annuus L.). J Agron Crop Sci. 174:351–362. doi: 10.1111/j.1439-037X.1995.tb01122.x
- Athar HR, Khan A, Ashraf M. 2008. Exogenously applied ascorbic acid alleviates salt-induced oxidative stress in wheat. Environ Exp Bot. 63:224–231. doi: 10.1016/j.envexpbot.2007.10.018
- Baker H, Frank O, DeAngelis B, Feingold S. 1980. Plasma tocopherol in man at various times after ingesting free or acetylated tocopherol. Nutr Rep Int. 21:531–536.
- Bates L, Waldren R, Teare I. 1973. Rapid determination of free proline for water-stress studies. Plant Soil. 39:205–207. doi: 10.1007/BF00018060
- Boem FHG, Lavado RS, Porcelli CA. 1997. Effects of waterlogging followed by a salinity peak on rapeseed (Brassica napus L.). J Agron Crop Sci. 178:135–140. doi: 10.1111/j.1439-037X.1997.tb00481.x
- Bose J, Rodrigo-Moreno A, Shabala S. 2013. ROS homeostasis in halophytes in the context of salinity stress tolerance. J Exp Bot. 430:1241–1257.
- Boursier P, Läuchli A. 1990. Growth responses and mineral nutrient relations of salt-stressed sorghum. Crop Sci. 30:1226–1233. doi: 10.2135/cropsci1990.0011183X003000060014x
- Bradford MM. 1976. A rapid and sensitive method for the quantitation of microgram quantities of protein utilizing the principle of protein-dye binding. Anal Biochem. 72:248–254. doi: 10.1016/0003-2697(76)90527-3
- Brunetti C, Di Ferdinando M, Fini A, Pollastri S, Tattini M. 2013. Flavonoids as antioxidants and developmental regulators: relative significance in plants and humans. Int J Mol Sci. 14:3540–3555. doi: 10.3390/ijms14023540
- Brunetti C, Guidi L, Sebastiani F, Tattini M. 2015. Isoprenoids and phenylpropanoids are key components of the antioxidant defense system of plants facing severe excess light stress. Environ Exp Bot. 119:54–62. doi: 10.1016/j.envexpbot.2015.04.007
- Cabot C, Sibole JV, Barceló J, Poschenrieder C. 2014. Lessons from crop plants struggling with salinity. Plant Sci. 226:2–13. doi: 10.1016/j.plantsci.2014.04.013
- Conklin P, Barth C. 2004. Ascorbic acid, a familiar small molecule intertwined in the response of plants to ozone, pathogens, and the onset of senescence. Plant Cell Environ. 27:959–970. doi: 10.1111/j.1365-3040.2004.01203.x
- DalCorso G, Farinati S, Furini A. 2010. Regulatory networks of cadmium stress in plants. Plant Signal Behav. 5:663–667. doi: 10.4161/psb.5.6.11425
- Dionisio-Sese ML, Tobita S. 1998. Antioxidant responses of rice seedlings to salinity stress. Plant Sci. 135:1–9. doi: 10.1016/S0168-9452(98)00025-9
- Dixon DP, Skipsey M, Edwards R. 2010. Roles for glutathione transferases in plant secondary metabolism. Phytochemistry. 71:338–350. doi: 10.1016/j.phytochem.2009.12.012
- Dolatabadian A, Sanavy SAMM, Gholamhoseini M, Joghan AK, Majdi M, Kashkooli AB. 2013. The role of calcium in improving photosynthesis and related physiological and biochemical attributes of spring wheat subjected to simulated acid rain. Physiol Mol Biol Plants. 19:189–198. doi: 10.1007/s12298-013-0165-7
- Elia AC, Galarini R, Taticchi MI, Dörr AJM, Mantilacci L. 2003. Antioxidant responses and bioaccumulation in Ictalurus melas under mercury exposure. Ecotoxicol Environ Saf. 55:162–167. doi: 10.1016/S0147-6513(02)00123-9
- El-Tayeb M. 2005. Response of barley grains to the interactive e.ect of salinity and salicylic acid. Plant Growth Regul. 45:215–224. doi: 10.1007/s10725-005-4928-1
- Espartero J, Sánchez-Aguayo I, Pardo JM. 1995. Molecular characterization of glyoxalase-I from a higher plant; upregulation by stress. Plant Mol Biol. 29:1223–1233. doi: 10.1007/BF00020464
- Fang Z, Bouwkamp JC, Solomos T. 1998. Chlorophyllase activities and chlorophyll degradation during leaf senescence in non-yellowing mutant and wild type of Phaseolus vulgaris L. J Exp Bot. 49:503–510.
- Fatma M, Asgher M, Masood A, Khan NA. 2014. Excess sulfur supplementation improves photosynthesis and growth in mustard under salt stress through increased production of glutathione. Environ Exp Bot. 107:55–63. doi: 10.1016/j.envexpbot.2014.05.008
- Foyer CH, Halliwell B. 1976. The presence of glutathione and glutathione reductase in chloroplasts: a proposed role in ascorbic acid metabolism. Planta. 133:21–25. doi: 10.1007/BF00386001
- Gallie DR. 2013. L-ascorbic acid: a multifunctional molecule supporting plant growth and development. Scientifica. 2013, Article ID 795964. doi: 10.1155/2013/795964
- Gapińska M, Skłodowska M, Gabara B. 2007. Effect of short- and long-term salinity on the activities of antioxidative enzymes and lipid peroxidation in tomato roots. Acta Physiol Plant. 30:11–18. doi: 10.1007/s11738-007-0072-z
- Hajiboland R. 2014. Reactive oxygen species and photosynthesis. In: Oxidative damage to plants: Antioxidant networks and signaling. San Diego, CA: Elsevier; p. 1–63.
- Hare P, Cress W. 1997. Metabolic implications of stress-induced proline accumulation in plants. Plant Growth Regul. 21:79–102. doi: 10.1023/A:1005703923347
- Hasanuzzaman M, Fujita M. 2013. Exogenous sodium nitroprusside alleviates arsenic-induced oxidative stress in wheat (Triticum aestivum L.) seedlings by enhancing antioxidant defense and glyoxalase system. Ecotoxicology. 22:584–596. doi: 10.1007/s10646-013-1050-4
- Hiscox JT, Israelstam G. 1979. A method for the extraction of chlorophyll from leaf tissue without maceration. Can J Bot. 57:1332–1334. doi: 10.1139/b79-163
- Hoque TS, Hossain MA, Mostofa MG, Burritt DJ, Fujita M, Tran L-SP. 2016. Methylglyoxal: an emerging signaling molecule in plant abiotic stress responses and tolerance. Front Plant Sci. 7:161. doi: 10.3389/fpls.2016.01341
- Hoque TS, Uraji M, Tuya A, Nakamura Y, Murata Y. 2012. Methylglyoxal inhibits seed germination and root elongation and up-regulates transcription of stress-responsive genes in ABA-dependent pathway in Arabidopsis. Plant Biology. 14:854–858. doi: 10.1111/j.1438-8677.2012.00607.x
- Hossain MA, Hossain MZ, Fujita M. 2009. Stress-induced changes of methylglyoxal level and glyoxalase I activity in pumpkin seedlings and cDNA cloning of glyoxalase I gene. Aust J Crop Sci. 3:53.
- Huang C, He W, Guo J, Chang X, Su P, Zhang L. 2005. Increased sensitivity to salt stress in an ascorbate-deficient Arabidopsis mutant. J Exp Bot. 56:3041–3049. doi: 10.1093/jxb/eri301
- Ibrahim S, Faryal S. 2014. Augmentation of Trigonella foenum-graecum L.(methi) growth under salinity stress and allelochemical stress through Mn+ B+ Zn mixture foliar spray. J Pharmacogn Phytochem. 3(2):39–44.
- Iqbal N, Umar S, Khan NA. 2015. Nitrogen availability regulates proline and ethylene production and alleviates salinity stress in mustard (Brassica juncea). J Plant Physiol. 178:84–91. doi: 10.1016/j.jplph.2015.02.006
- Jiang Y, Huang B. 2001. Effects of calcium on antioxidant activities and water relations associated with heat tolerance in two cool-season grasses. J Exp Bot. 52:341–349. doi: 10.1093/jexbot/52.355.341
- Jiang W, Sun X, Xu H, Mantri N, Lu H. 2014. Optimal concentration of zinc sulfate in foliar spray to alleviate salinity stress in Glycine soja. J Agri Sci Technol. 16:445–460.
- Kader MA, Lindberg S. 2010. Cytosolic calcium and pH signaling in plants under salinity stress. Plant Signal Behav. 5:233–238. doi: 10.4161/psb.5.3.10740
- Karim MR, Zhang Y-Q, Zhao R-R, Chen X-P, Zhang F-S, Zou C-Q. 2012. Alleviation of drought stress in winter wheat by late foliar application of zinc, boron, and manganese. J Plant Nutr Soil Sci. 175:142–151. doi: 10.1002/jpln.201100141
- Kaur C, Singla-Pareek SL, Sopory SK. 2014. Glyoxalase and methylglyoxal as biomarkers for plant stress tolerance. Crit Rev Plant Sci. 33:429–456. doi: 10.1080/07352689.2014.904147
- Kawakami A, Sato Y, Yoshida M. 2008. Genetic engineering of rice capable of synthesizing fructans and enhancing chilling tolerance. J Exp Bot. 59:793–802. doi: 10.1093/jxb/erm367
- Kumar V, Khare T. 2016. Differential growth and yield responses of salt-tolerant and susceptible rice cultivars to individual (Na+ and Cl−) and additive stress effects of NaCl. Acta Physiol Plant. 38:25. doi: 10.1007/s11738-015-2047-9
- Luck H. 1971. Catalases. In: Methods of enzymatic analysis. New York: Academic Press; p. 885–893.
- Lutts S, Kinet JM, Bouharmont J. 1995. Changes in plant response to NaCl during development of rice (Oryza sativa L.) varieties differing in salinity resistance. J Exp Bot. 46:1843–1852. doi: 10.1093/jxb/46.12.1843
- Ma D, Sun D, Wang C, Ding H, Qin H, Hou J, Huang X, Xie Y, Guo T. 2017. Physiological responses and yield of wheat plants in zinc-mediated alleviation of drought stress. Front Plant Sci. 8:786. doi: 10.3389/fpls.2017.00786
- Majer P, Neugart S, Krumbein A, Schreiner M, Hideg É. 2014. Singlet oxygen scavenging by leaf flavonoids contributes to sunlight acclimation in Tilia platyphyllos. Environ Exp Bot. 100:1–9. doi: 10.1016/j.envexpbot.2013.12.001
- Miyake C, Asada K. 1992. Thylakoid-bound ascorbate peroxidase in spinach chloroplasts and photoreduction of its primary oxidation product monodehydroascorbate radicals in thylakoids. Plant Cell Physiol. 33:541–553.
- Mohammadi R, Mendioro MS, Diaz GQ, Gregorio GB, Singh RK. 2013. Mapping quantitative trait loci associated with yield and yield components under reproductive stage salinity stress in rice (Oryza sativa L.). J Genet. 92:433–443. doi: 10.1007/s12041-013-0285-4
- Mostofa MG, Fujita M. 2013. Salicylic acid alleviates copper toxicity in rice (Oryza sativa L.) seedlings by up-regulating antioxidative and glyoxalase systems. Ecotoxicology. 22:959–973. doi: 10.1007/s10646-013-1073-x
- Munns R, Tester M. 2008. Mechanisms of salinity tolerance. Annu Rev Plant Biol. 59:651–681. doi: 10.1146/annurev.arplant.59.032607.092911
- Nahar K, Hasanuzzaman M, Alam MM, Fujita M. 2015. Exogenous glutathione confers high temperature stress tolerance in mung bean (Vigna radiata L.) by modulating antioxidant defense and methylglyoxal detoxification system. Environ Exp Bot. 112:44–54. doi: 10.1016/j.envexpbot.2014.12.001
- Nahar K, Hasanuzzaman M, Alam MM, Rahman A, Suzuki T, Fujita M. 2016. Polyamine and nitric oxide crosstalk: antagonistic effects on cadmium toxicity in mung bean plants through upregulating the metal detoxification, antioxidant defense and methylglyoxal detoxification systems. Ecotoxicol Environ Saf. 126:245–255. doi: 10.1016/j.ecoenv.2015.12.026
- Nakano Y, Asada K. 1981. Hydrogen peroxide is scavenged by ascorbate-specific peroxidase in spinach chloroplasts. Plant Cell Physiol. 22:867–880.
- Noctor G, Foyer CH. 1998. Ascorbate and glutathione: keeping active oxygen under control. Annu Rev Plant Physiol Plant Mol Biol. 49:249–279. doi: 10.1146/annurev.arplant.49.1.249
- Novo LAB, Covelo EF, González L. 2014. Effect of salinity on zinc uptake by brassica juncea. Int J Phytoremediation. 16:704–718. doi: 10.1080/15226514.2013.856844
- Racker E. 1951. The mechanism of action of glyoxalase. J Biol Chem. 190:685–696.
- Rahman A, Mostofa MG, Alam MM, Nahar K, Hasanuzzaman M, Fujita M. 2015a. Calcium mitigates arsenic toxicity in rice seedlings by reducing arsenic uptake and modulating the antioxidant defense and glyoxalase systems and stress markers. BioMed Res Int. 2015:1–12.
- Rahman A, Mostofa MG, Nahar K, Hasanuzzaman M, Fujita M. 2015b. Exogenous calcium alleviates cadmium-induced oxidative stress in rice (Oryza sativa L.) seedlings by regulating the antioxidant defense and glyoxalase systems. Braz J Bot. 39:393–407. doi: 10.1007/s40415-015-0240-0
- Rahman A, Nahar K, Hasanuzzaman M, Fujita M. 2016. Calcium supplementation improves Na(+)/K(+) ratio, antioxidant defense and glyoxalase systems in salt-stressed rice seedlings. Front Plant Sci. 7:609.
- Rao PS, Mishra B, Gupta SR. 2013. Effects of soil salinity and alkalinity on grain quality of tolerant, semi-tolerant and sensitive rice genotypes. Rice Sci. 20:284–291. doi: 10.1016/S1672-6308(13)60136-5
- Rao KM, Sresty T. 2000. Antioxidative parameters in the seedlings of pigeonpea (Cajanus cajan (L.) Millspaugh) in response to Zn and Ni stresses. Plant Sci. 157:113–128. doi: 10.1016/S0168-9452(00)00273-9
- Reddy VS, Sopory SK. 1999. Glyoxalase I fromBrassica juncea: molecular cloning, regulation and its over-expression confer tolerance in transgenic tobacco under stress. Plant J. 17:385–395. doi: 10.1046/j.1365-313X.1999.00390.x
- Rezaei M, Abbasi H. 2014. Foliar application of nanochelate and non-nanochelate of zinc on plant resistance physiological processes in cotton (Gossipium hirsutum L.). Iran J Plant Physiol. 4:1137–1144.
- Rockenfeller P, Madeo F. 2008. Apoptotic death of ageing yeast. Exp Gerontol. 43:876–881. doi: 10.1016/j.exger.2008.08.044
- Rubio F, Flores P, Navarro JM, Martı´ V. 2003. Effects of Ca2+, K+ and cGMP on Na+ uptake in pepper plants. Plant Sci. 165:1043–1049. doi: 10.1016/S0168-9452(03)00297-8
- Samreen T, Shah HU, Ullah S, Javid M. 2017. Zinc effect on growth rate, chlorophyll, protein and mineral contents of hydroponically grown mungbeans plant (Vigna radiata). Arab J Chem. 10:S1802–S1807. doi: 10.1016/j.arabjc.2013.07.005
- Sarwat M, Ahmad P, Nabi G, Hu X. 2013. Ca2+ signals: the versatile decoders of environmental cues. Crit Rev Biotechnol. 33:97–109. doi: 10.3109/07388551.2012.672398
- Siddiqui SN, Umar S, Iqbal M. 2015. Zinc-induced modulation of some biochemical parameters in a high-and a low-zinc-accumulating genotype of Cicer arietinum L. grown under Zn-deficient condition. Protoplasma. 252:1335–1345. doi: 10.1007/s00709-015-0767-8
- Singla-Pareek SL, Yadav SK, Pareek A, Reddy MK, Sopory SK. 2007. Enhancing salt tolerance in a crop plant by overexpression of glyoxalase II. Transgenic Res. 17:171–180. doi: 10.1007/s11248-007-9082-2
- Smart RE, Bingham GE. 1974. Rapid estimates of relative water content. Plant Physiol. 53:258–260. doi: 10.1104/pp.53.2.258
- Smirnoff N. 2000. Ascorbic acid: metabolism and functions of a multi-facetted molecule. Curr Opin Plant Biol. 3:229–235. doi: 10.1016/S1369-5266(00)00069-8
- Tavallali V, Rahemi M, Maftoun M, Panahi B, Karimi S, Ramezanian A, Vaezpour M. 2009. Zinc influence and salt stress on photosynthesis, water relations, and carbonic anhydrase activity in pistachio. Sci Hortic. 123:272–279. doi: 10.1016/j.scienta.2009.09.006
- Tuna AL, Kaya C, Ashraf M, Altunlu H, Yokas I, Yagmur B. 2007. The effects of calcium sulphate on growth, membrane stability and nutrient uptake of tomato plants grown under salt stress. Environ Exp Bot. 59:173–178. doi: 10.1016/j.envexpbot.2005.12.007
- Tuteja N, Ahmad P, Panda BB, Tuteja R. 2009. Genotoxic stress in plants: shedding light on DNA damage, repair and DNA repair helicases. Mutat Res Rev Mutat Res. 681:134–149. doi: 10.1016/j.mrrev.2008.06.004
- Unal BT, Aktas L, Guven A. 2014. Effects of salinity on antioxidant enzymes and proline in leaves of barley seedlings in different growth stages. Bulg J Agri Sci. 20:883–887.
- van Rossum MW, Alberda M, van der Plas LH. 1997. Role of oxidative damage in tulip bulb scale micropropagation. Plant Sci. 130:207–216. doi: 10.1016/S0168-9452(97)00215-X
- Veena RV, Sopory S. 1999. Glyoxalase I from Brassica juncea: molecular cloning, regulation and its over-expression confer tolerance in transgenic tobacco under stress. Plant J 17:385–395. doi: 10.1046/j.1365-313X.1999.00390.x
- Velikova V, Yordanov I, Edreva A. 2000. Oxidative stress and some antioxidant systems in acid rain-treated bean plants. Plant Sci. 151:59–66. doi: 10.1016/S0168-9452(99)00197-1
- Weisany W, Sohrabi Y, Heidari G, Siosemardeh A, Badakhshan H. 2014. Effects of zinc application on growth, absorption and distribution of mineral nutrients under salinity stress in soybean (Glycine max L.). J Plant Nutr. 37:2255–2269. doi: 10.1080/01904167.2014.920386
- Weisany W, Sohrabi Y, Heidari G, Siosemardeh A, Ghassemi-Golezani K. 2011. Physiological responses of soybean (Glycine max L.) To zinc application under salinity stress. Aust J Crop Sci. 5:1441.
- Weisany W, Sohrabi Y, Heidari G, Siosemardeh A, Ghassemi-Golezani K. 2012. Changes in antioxidant enzymes activity and plant performance by salinity stress and zinc application in soybean (Glycine max L.). Plant Omics. 5:60.
- Wild R, Ooi L, Srikanth V, Münch G. 2012. A quick, convenient and economical method for the reliable determination of methylglyoxal in millimolar concentrations: the N-acetyl-l-cysteine assay. Anal Bioanal Chem. 403:2577–2581. doi: 10.1007/s00216-012-6086-4
- Winkel-Shirley B. 2002. Biosynthesis of flavonoids and effects of stress. Curr Opin Plant Biol. 5:218–223. doi: 10.1016/S1369-5266(02)00256-X
- Wolf B. 1982. A comprehensive system of leaf analyses and its use for diagnosing crop nutrient status. Commun Soil Sci Plant Anal. 13:1035–1059. doi: 10.1080/00103628209367332
- Wu G, Wang S. 2012. Calcium regulates K+/Na+ homeostasis in rice (Oryza sativa L.) under saline conditions. Plant Soil Environ. 58:121–127. doi: 10.17221/374/2011-PSE
- Xu C, Li X, Zhang L. 2013. The effect of calcium chloride on growth, photosynthesis, and antioxidant responses of Zoysia japonica under drought conditions. PLoS One. 8:e68214. doi: 10.1371/journal.pone.0068214
- Yadav SK, Singla-Pareek SL, Ray M, Reddy MK, Sopory SK. 2005. Methylglyoxal levels in plants under salinity stress are dependent on glyoxalase I and glutathione. Biochem Biophys Res Commun. 337:61–67. doi: 10.1016/j.bbrc.2005.08.263
- Yang T, Poovaiah B. 2003. Calcium/calmodulin-mediated signal network in plants. Trends Plant Sci. 8:505–512. doi: 10.1016/j.tplants.2003.09.004
- Yu C-W, Murphy TM, Lin C-H. 2003. Hydrogen peroxide-induced chilling tolerance in mung beans mediated through ABA-independent glutathione accumulation. Funct Plant Biol. 30:955–963. doi: 10.1071/FP03091
- Zhishen J, Mengcheng T, Jianming W. 1999. The determination of flavonoid contents in mulberry and their scavenging effects on superoxide radicals. Food Chem. 64:555–559. doi: 10.1016/S0308-8146(98)00102-2
- Zorrig W, Shahzad Z, Abdelly C, Berthomieu P. 2012. Calcium enhances cadmium tolerance and decreases cadmium accumulation in lettuce (Lactuca sativa). Afr J Biotechnol. 11:8441–8448.