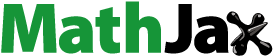
ABSTRACT
Piriformospora indica (P. indica) is a mutualistic endophyte that colonizes plant roots. In this study, effects of P. indica on rice resistance against rice leaffolder (Cnaphalocrocis medinalis Guenée) were investigated. Growth inhibition and leaf injury caused by leaffolder larvae infestation in P. indica-colonized rice was significantly alleviated. Moreover, growth retardation was observed in larvae which fed on P. indica-colonized plants. JA-Ile levels and trypsin inhibitor activities in leaf tissues of P. indica-colonized plants were higher than those in noncolonized plants under leaffolder-infested conditions. P. indica effects on increasing trypsin inhibitor expression and reducing larval growth were repressed by aspirin. JA signaling drives P. indica-enhanced insect resistance in rice, and trypsin inhibitors might be candidates involved in this mechanism. Changes in antioxidant enzyme activities and malondialdehyde levels in P. indica-inoculated plants were observed, and it was demonstrated that oxidative stress induced by insect infestation could be reduced in P. indica-colonized plants.
Introduction
Piriformospora indica (P. indica) is a root endophytic fungus mutually beneficial to associated plants, and it has a broad range of hosts including monocots and dicots (Verma et al. Citation1998). The effects of P. indica colonization on increasing biomass have been proven in several plant species such as rice, Chinese cabbage and barley (Lee et al. Citation2011; Hilbert et al. Citation2012; Tsai et al. Citation2020). The capability of P. indica to accelerate plant growth and to enhance crop yield could result from promoting root development and nutrient uptake (Achatz et al. Citation2010; Ansari et al. Citation2013). P. indica colonization not only benefits plant growth but also improves environmental stress tolerance (Sherameti et al. Citation2008; Jiang et al. Citation2020). Several studies have indicated that the contributions of beneficial endophytes to improving tolerance against abiotic stresses are mediated enhancing the activity of reactive oxygen species (ROS) scavenging (Gill and Tuteja Citation2010; Lata et al. Citation2018; White et al. Citation2019). Under drought stresses, a reduction in malondialdehyde (MDA) and increased activities of antioxidative enzymes were found in P. indica-colonized Chinese cabbage and rice (Sun et al. Citation2010; Tsai et al. Citation2020). Moreover, P. indica colonization can also contribute to maintaining the osmotic potential of host plants by modulating proline accumulation under water stress conditions (Saddique et al. Citation2018).
Infestation of insect herbivores is a severe problem that threatens crop production. Plants develop several defense mechanisms against insect pests or to heal injured tissues. Insect chewing or mechanical wounding usually trigger jasmonic acid (JA) biosynthesis, and sucking insect attack often induces salicylic acid (SA) biosynthesis (Vos et al. Citation2013; Huot et al. Citation2014; Singh et al. Citation2018). The expression of several JA biosynthesis genes such as lipoxygenases and allene oxide synthase (AOS) could be induced by wounding stimuli (Ziegler et al. Citation2001; Upadhyay and Mattoo Citation2018). Moreover, the jasmonate resistant gene (JAR) encodes a protein that catalyzes the conversion of JA to JA-Ile, and it is activated in wound-stimulated plants (Lyons et al. Citation2013). JA and ethylene contents increased significantly in corn attacked by beet armyworm (Spodoptera exigua) larvae (Schmelz et al. Citation2003). In white butterfly (Pieris rapae) larvae infested Arabidopsis, both JA and JA-Ile contents increased (Vos et al. Citation2013). Moreover, when plants are subjected to mechanical damage or insect attack, protease inhibitors are often induced to inhibit the activity of insect intestinal proteases and repress digestive systems (Koiwa et al. Citation1997; Shamsi et al. Citation2016). Wang et al. (Citation2011b) found that the activity of trypsin inhibitor, a kind of protease inhibitor, could be induced in leaffolder (Cnaphalocrocic medinalis) larvae-infested rice plants mediated through ethylene and SA signaling pathways. Increases in JA and trypsin inhibitors were also observed in rice stem borer (Chilo suppressalis)-infested rice plants (Liu et al. Citation2018). In several plant species such as corn, cotton and potatoes, H2O2 induced by mechanical stimuli functions as a secondary messenger to trigger the JA-dependent defense pathway (Orozco-Cardenas and Ryan Citation1999; Orozco-Cárdenas et al. Citation2001).
The impact of symbiotic fungi on herbivore resistance has been investigated in arbuscular mycorrhizal fungi (AMF)-colonized plants. Tomato plants preinoculated with Glomus mosseae performed stronger expressions of defense genes, such as protease inhibitors, in insect-feeding conditions (Song et al. Citation2013). In potato, AMF inoculation enhanced JA biosynthesis-related gene expressions and also induced priming effects of resistance against cabbage loopers (Schoenherr et al. Citation2019). In contrary, some cases have shown that symbiotic mycorrhizal fungi such as Sebacina vermifera might have a negative effect on insect resistance by reducing defective protease inhibitor activity (Barazani et al. Citation2005). Fall Armyworm feeding caused more severe foliar damage in AMF-colonized maize plants than in noncolonized plants (Real-Santillán et al. Citation2019). The effect of AMF symbiosis on insect pest growth has also been observed, and the results showed that the weight and number of aphids collected from AMF-colonized potato plants were not obviously changed (Rizzo et al. Citation2020). In rice plants, AMF symbiosis increased of fall armyworm larval growth and rice water weevil larvae density; however, the effect was dependent on soil status (Bernaola and Stout Citation2019). Daneshkhah et al. (Citation2013) indicated that P. indica colonization can help Arabidopsis protect against nematodes. In addition, the effect of P. indica on insect tolerance has been observed in rice infested with water weevils (Lissorhoptrus oryzophilus). Both rice water weevil adults and larvae induce JA biosynthesis in rice roots to inhibit root growth; however, gibberellic acid (GA) biosynthesis is promoted by the antagonism of JA under P. indica symbiotic conditions and reduces the negative effect of the water weevil on root growth (Cosme et al. Citation2016). The effects of P. indica symbiosis on increasing herbivore resistance in sweet potato were reported recently (Li et al. Citation2021). However, understanding on the effect of P. indica symbiosis on herbivore resistance of colonized plants is still limited.
Cnaphalocrocic medinalis (Guenée), commonly called rice leaffolder, is an important insect pest in Asia that is widely distributed in rice production areas (Hill Citation1983). Rice leaffolder larvae fold leaf blades and chew leaf tissues to reduce leaf area and decrease photosynthetic efficiency. Padmavathi et al. (Citation2013) indicated that more than 50% of grain would fail to fill when 25% of the flag leaf area was damaged at the flowering stage. In this study, the effects of P. indica colonization on resistance against leaffolder larval infestation were investigated in rice plants, and the mechanisms of herbivore resistance induced by P. indica colonization were further clarified.
Materials and methods
Plant material and growth conditions
The rice cultivar (Oryza sativa L.) used in this study was Taichung native 1 (TCN1). Rice seeds were sterilized using 1% (v/v) NaOCl for 30 min before imbibition at 30°C in the dark for 2 d. Next, seedlings were cultured with Kimura solution (Chu and Lee Citation1989). The photoperiod for seedling growth was 12 h light/12 h dark, and the temperature cycle was 30°C/25°C (day/night). Leaf counting started from the first leaf that appeared after coleoptile (Chang et al. Citation2019). A rice osjar1 mutant (no. NG8384) obtained from the National Institute of Agrobiological Sciences in Japan was a Tos17 insertion line and was also applied in this study. The osjar1 mutant presents incomplete husk closure and abnormally developed seeds (Riemann et al. Citation2008). In our study, hemizygous osjar1 mutants were used as materials.
P. indica cultivation
P. indica was maintained and subcultured with Kaefer agar-medium (Hill and Kafer Citation2001). For liquid culture, P. indica (1 cm in diameter) was picked up from 14 d-agar medium culture and continuously cultured in 50 mL Kaefer liquid medium at 30°C.
P. indica inoculation
After P. indica liquid was cultured for 12 d, the liquid medium was filtered out and P. indica was collected. The collected P. indica was rinsed with distilled water. Following, three grams of P. indica was homogenized with Kimura hydroponic solution before cocultured with germinated rice seedlings (Tsai et al. Citation2020). The inoculation solution was suspended daily within first 6 d, and then it was replaced to culture solution without P. indica for rice growing continuously. Control plants were grown in Kimura hydroponic solution without P. indica.
P. indica colonization evaluation
P. indica colonization was investigated with Pitef1 transcripts in rice plant roots. The Pitef1 gene encoded translation elongation factor EF-1α of P. indica has been widely used as a molecular marker to monitor the active mycelium amount in the roots of P. indica-colonized plants (Bütehorn et al. Citation2000; Jogawat et al. Citation2016; Guo et al. Citation2017; Liu et al. Citation2019).
Rice leaffolder (Cnaphalocrocic medinalis (Guenée)) rearing
Rice leaffolder (Cnaphalocrocic medinalis (Guenée)) is propagated at 30°C/25°C (day/night). Larvae were fed on corn (Tainan white cultivar) seedlings and moths were fed with 10% sucrose solution (Chang et al. Citation2019).
Rice leaffolder larvae feeding treatment
The 3rd instar larvae were starved for 3 h before transfer to rice plants. A larva was released onto the 4th leaf of each rice plant. After the period required for each experiment, the larvae were removed and plant leaves were collected for subsequent analyses.
Aspirin treatment
Aspirin is an inhibitor of allene oxide synthase (AOS), a key enzyme of the JA biosynthetic pathway (Pan et al. Citation1998). Aspirin was predissolved in NaOH solution. Rice plants were grown in Kimura solution untill 4-leaf stage and then treated with 150 μM aspirin for 12 h before rice leaffolder larval feeding treatment. For the experiments analyzing aspirin effects on OsAOS1 gene expressions and larval growth rate, five plants were used for each treatment in each independent experiment. RNA for further gene expression analysis was extracted from leaves collected from the five plants for each treatment. Three repeat experiments were performed, and the data are shown as the mean of three experiments. The weight of each larva collected from plants was measured individually, and the average weight of five larvae was calculated for each treatment. The experiments were repeated three times, and the data were presented as the mean of three experiments.
Leaf injury score evaluation
Leaf injury evaluation was performed according to the method of Edilberto (Citation2013). The percentage of the damaged area to the leaf area was counted, and the levels of leaf damage caused by rice leaffolders were classified according the damage area percentage.
Chlorophyll fluorescence assay
Chlorophyll fluorescence of rice leaf tissues was monitored by MAXI-Imaging-PAM (WALZ, Germany). After dark acclimation for 30 min, the minimum fluorescence (Fo) and the maximum fluorescence (Fm) of the 4th leaf were measured after the saturated light source was irradiated. The difference between Fo and Fm is variable fluorescence (Fv), and the quantum yield (Fv / Fm) of the large photochemistry was calculated. Plants were subjected to light acclimation under photosynthetic active radiation (PAR) with a light intensity of 530 μmol m−2 s−1 for 3 min, and the steady state fluorescence (Fs) and the maximum fluorescence (Fm’) of the 4th leaf were measured. The actual photochemical quantum yield was calculated according to the formula ΦPSII = (Fm’ − Fs)/Fm’.
Larvae weight and metamorphosis analysis
To evaluate the relative growth rate and metamorphosis of rice leaffolder larvae fed on P. indica-colonized and noncolonized rice plants, the 3rd instar larvae of the rice leaffolder were weighed before fed with experimental plant materials, and then larvae were weighed again after feeding for 3 d (grown to 4th instar) and 6 d (grown to 5th instar). The relative growth rate of larvae was estimated according to the changes in weight after feeding. The days of feeding are expressed as D. The larval weights before and after feeding are indicated as B and A, respectively. Relative growth rate = (A − B)/[D*(A + B)/2] (Han et al. Citation2015). Moreover, the number of pupae and adults were counted after larval metamorphosis, and the percentage of successful metamorphosis of larvae into pupae and adults was calculated. The number of larval samples was 15 for each treatment of an independent experiment, and the average larval weight was calculated. The experiments were repeated three times, and the results are shown as the mean of three experiments.
Real-time RT–PCR
The One Step SYBR® PrimeScript™ RT–PCR Kit II (TAKARA, Shiga, Japan) was used for real-time RT–PCR analysis, and the process followed the description of Chang et al. (Citation2019). Primers used for detecting ubiquitin and OsAOS1 mRNA were described by Wang et al. (Citation2011a). Primers for detecting the expression of P. indica Pitef1 gene were 5′-GAAGAGCGCGGAAAAGGCT-3′ and 5′-AAAACTACTCTACATCGACG-3′. Specific primers designed for analysis of OsJAR1 gene expression were 5′-AAGGTTTGTGAACCCATCAAACAGC-3′ and 5′-AATAATACTTTGCAGCACTTGTTACG-3′ (Lu et al. Citation2015).
Trypsin inhibitor analysis
Proteins were extracted from rice leaf tissues according to the method of Chen et al. (Citation2006). Protein samples for each treatment were extracted from leaves collected from four plants. Extracted protein was added to the trypsin inhibitor assay reaction solution which included trypsin and benzoyl-arginine p-nitroanilide (BApNA, a substrate of trypsin). Trypsin inhibitor amounts in protein samples were determined according to the inhibition level of BApNA digested by trypsin. Trypsin inhibitor amounts were finally calculated according to the standard curve based on the soybean trypsin inhibitor protein. Experiments were repeated three times, and the data are shown as the mean of three experiments.
JA content analysis
Extraction and analysis of plant hormones were performed according to the description by Chen et al. (Citation2014), and LC-MS/MS analysis was performed at the Agricultural Biotechnology Research Center of Academia Sinica, Taiwan. LC-MS/MS analysis was performed using a linear ion trap–orbitrap mass spectrometer (Orbitrap Elite; Thermo Fisher Scientific) and UHPLC system (ACQUITY UPLC; Waters). The detected hormones were separated by a HSS T3 (Waters) column and mass spectrometry was performed by electrospray ionization (ESI). To establish a standard for absolute quantification, stable isotope-labeled hormones such as dihydrojasmonic acid (H2JA, OlChemim, cat. no.0145324) were added to samples. The extract buffer included 100 mL 2-propanol, 50 mL dH2O and 100 μL 12 N HCl; moreover, H2JA (0.375 μg) were added as standards. Leaf tissues (approximately 60 mg) were ground with liquid nitrogen and then homogenized in 1 mL extract buffer and shaken for 30 min at 4°C. Following, the samples were centrifuged at 13,000×g for 10 min, and then 6 mL dichloromethane was added to the supernatant (700 μL). After shaking for 30 min at 4°C, the samples were centrifuged. Samples were dried by a vacuum concentrator for approximately 1 h before storage at −20°C until LC-MS analysis. The sample was dissolved in 200 μL of methanol and then centrifuged at 4°C before the supernatant was subjected to LC-MS analysis. The data analysis formula was as follows: (JA area /H2JA area) × [1/FW (g)] × [isotope content in 1 mL extraction buffer (ng)]. For JA content analysis, leaf samples were collected from four plants for each treatment in each independent experiment. Three repeat experiments were performed, and the results are shown as the mean of three experiments.
MDA analysis
MDA of leaf tissues was extracted by trichloroacetic acid buffer and analyzed according to the method described by Heath and Packer (Citation1968).
Antioxidant enzyme activity analysis
The superoxide dismutase (SOD) activity assay was performed according to Paoletti et al. (Citation1986). Rice leaf tissues (50 mg) were homogenized with 2 mL of sodium phosphate buffer (50 mM, pH 7.4) before centrifugation at 12,000xg for 20 min at 4°C. The supernatant (0.2 mL) was mixed sequentially with 1.6 mL Tea-Dea buffer (100 mM triethanolamine-diethanolamine, pH 7.4), 0.08 mL reduced form nicotinamide adenine dinucleotide (NADH, 7.5 mM), 0.05 mL EDTA (100 mM) and MnCl2 (50 mM) buffer (pH 7.0). Then, 1 mL β-mercaptoethanol (10 mM) was added to the reaction solution. The reaction was carried out for 10 min before the absorbance was measured at 340 nm. One unit of SOD activity was defined as the amount of protein to inhibit 50% NADH oxidation per min. For ascorbate peroxidase (APX) and glutathione reductase (GR) activity assays, leaf samples were homogenized with sodium phosphate buffer (50 mM, pH 6.8). APX activity was determined following the method of Nakano and Asada (Citation1981), and one unit of activity was defined as the amount of enzyme that cause 1 μmol ascorbate degradation. GR activity was measured following the method of Foster and Hess (Citation1980). One unit of GR activity was defined as the amount of enzyme that contributed to the degradation of 1 μmol NADPH.
Statistical analyses
Statistical analyses were performed using R software for Fisher's least significant difference (LSD) and Excel for Student’s t-test.
Results
Effects of P. indica colonization on insect resistance of rice plants
Changes in the injury levels of leaffolder larvae-induced leaf damage were observed in P. indica-colonized rice plants. The 4-leaf-stage noncolonized and P. indica-colonized rice plants were given with leaffolder larvae. After 3 d of larval treatment, wilting and growth slowdown occurred significantly in the leaf tissues of noncolonized plants. However, the leaffolder infestation induced damage was alleviated in P. indica-colonized plants (A). P. indica colonization was evaluated according to the expression of Pitef1 in plant roots (B). Furthermore, the fresh weights of noncolonized plant shoots and roots decreased 40% and 17%, respectively, after larval feeding (C,D). In P. indica-colonized plants, the decrease in fresh weight caused by leaffolder infestation was 28% and 14% in shoot and root tissues, respectively (C,D). The influence of P. indica on photosynthetic systems was also evaluated under larval chewing stimulation conditions. The maximum photochemical quantum yield (Fv/Fm) value of the plants without the leaffolder treatment was 0.80. The Fv/Fm values of larvae-infested leaves decreased to 0.62. However, the Fv/Fm was still maintained at 0.74 in larvae-attacked leaves of P. indica-colonized plants (E). In addition, the actual photochemical quantum yield (ФPSII) was also investigated. The data in F show that the ФPSII of non-P. indica inoculated plants significantly decreased from 0.39 to 0.26 after leaffolder chewing. P. indica-colonized plants maintained a ФPSII value of 0.35 even under larvae-infested conditions (F).
Figure 1. Effects of P. indica colonization on rice growth and photosynthesis under rice leaffolder infestation conditions. The 4-leaf-stage P. indica-inoculated and noninoculated plants were treated with rice leaffolder larvae. The phenotype (A), biomass of shoots (C) and roots (D), Fv/Fm (E) and ΦPSII (F) of plants were detected after 3 d of larval feeding treatments. In (C) to (F), the data are shown the mean ± SE of six independent experiments. There were five plant samples for each treatment in each independent experiment. (B) Expression of Pitef1 genes in plant roots (n = 3). Control, non-P. indica inoculated and nonlarvae-feeding treatment. LF, rice leaffolder larvae. The different letters indicate statistically significant differences among group samples (P < 0.05).
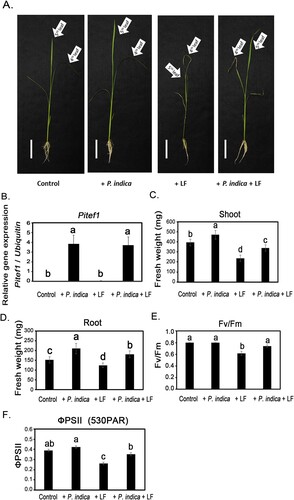
Growth retardation of leaffolder larvae fed on P. indica-colonized rice plants
In addition to plant growth, the development of leaffolder larvae fed on P. indica-colonized and noncolonized plants was also monitored. In the case of non-P. indica inoculated plants, the 3rd-instar larvae grew into 4th instars with a relative growth rate of 0.32, and that of larvae grown on P. indica-inoculated plants was 0.28 (A). Moreover, the relative growth rate of the 5th-instar larvae grown on P. indica-colonized plants was also significantly lower than that of the larvae grown on noncolonized plants (A). The number of larvae that successfully pupated or emerged was counted. The data in B shown that 82.2% of the leaffolders grown on noncolonized rice seedlings successfully pupated, but only 66.7% of the leaffolders grown on P. indica-colonized rice seedlings successfully pupated. A total of 68.9% of the larvae grown on noncolonized plants successfully emerged into moths; however, only 46.7% of larvae grown on P. indica-colonized plants transformed to moths (B). Therefore, P. indica colonization conducted had an obvious impact on the metamorphosis of leaffolder larvae.
Figure 2. Growth of rice leaffolder fed on P. indica-colonized rice plants. (A) Relative growth rate of larvae fed on P. indica-colonized and noncolonized rice plants. Larvae (3rd instar) were fed plants for 3 d (the larvae grew to 4th instar) and 6 d (larvae grew to 5th instar), and the relative growth rate of larvae was calculated according to the weight of 3rd, 4th and 5th-instar larvae. (B) Percentage of pupation and emergence of larvae. Control, larvae fed on noncolonized plants. The results are shown as the mean ± SE of three independent experiments. Significant differences were analyzed by Student’s t-test. *p < 0.05, ** p < 0.01.
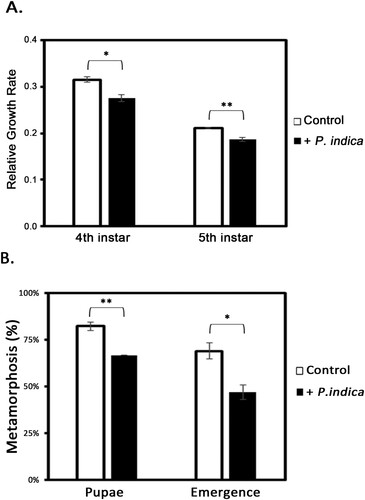
P. indica modified JA biosynthesis in larvae-infested rice plants
P. indica colonization stimulated both free-form JA and jasmonyl-isoleucine (JA-Ile) increases in leaf tissues (). To reveal the effects of P. indica colonization on defensive hormone biosynthesis under herbivory stresses, the amount of JA in leaf tissues of rice plants with or without P. indica inoculation was detected after larval infestation for 12 h. The results showed that the contents of both JA and JA-Ile in leaves significantly increased due to larval chewing (). On the other hand, in larvae fed leaves, JA level of the P. indica-colonized plants was lower than noncolonized plants after infestation, but the JA-Ile content of P. indica-colonized plants was significantly higher than that of noncolonized plants ().
Figure 3. JA and JA-Ile content in leaf tissues of P. indica-inoculated plants under rice leaffolder infestation conditions. The 4-leaf-stage P. indica-inoculated and noninoculated plants were treated with leaffolder larvae, and leaves were collected after 12 h of larval feeding for detection of JA and JA-Ile content. Data are shown as the mean ± SE of three independent experiments. Control, non-P. indica inoculated and nonlarvae-feeding treatment. LF, rice leaffolder larvae. The different letters indicate statistically significant differences among group samples (P < 0.05).
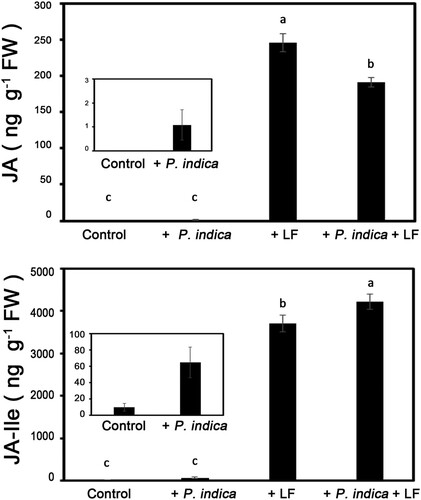
To further clarify whether JA was involved in P. indica-promoted insect herbivore resistance, aspirin, a JA biosynthesis inhibitor, was applied to P. indica-colonized rice plants. Rice plants were pretreated with aspirin for 12 h and then given rice leaffolder larvae for 3 d before leaf samples were collected to determine the expression of JA biosynthesis allene oxide synthase 1 gene (OsAOS1). The transcript level of OsAOS1 in P. indica-colonized plants was higher than that in noncolonized plants under leaffolder infestation conditions; however, P. indica-induced OsAOS1 expression was significantly repressed in aspirin-treated plants (A). Furthermore, the growth rate of larvae fed on P. indica- and aspirin-treated rice plants was observed. Under non-aspirin treatment conditions, the growth rate of larvae with non-P. indica inoculated plants was 0.31, and it decreased to 0.27 if the larvae were fed P. indica-colonized plants. However, P. indica colonization induced larval growth retardation was not present in larvae fed on aspirin-treated plants (B).
Figure 4. JA biosynthesis gene expressions and larval growth rate in aspirin-treated plants. (A) Effects of aspirin on OsAOS1 gene expression in leaves of P. indica-inoculated and larvae treated plants. (B) Relative growth rate (RGR) of larvae fed on aspirin-treated and P. indica-inoculated plants. The 4-leaf-stage plants were pretreated with aspirin for 12 h before larval feeding. Leaf samples were collected to analyze OsAOS1 expressions after 3 d of larval feeding treatment. Larvae were weighed before being moved to the target plants and after 3 d of feeding, and RGR was calculated based on the changes in weight. Data are the mean ± SE of three independent experiments. The different letters indicate statistically significant differences among group samples (P < 0.05). Control, non-inoculated and nonlarvae-feeding treatment. LF, rice leaffolder larvae.
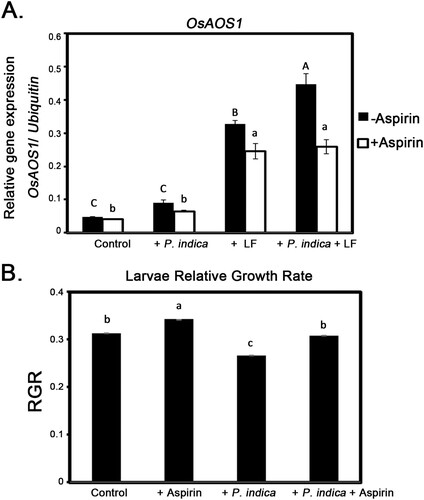
Moreover, P. indica effects on leaf damage caused by leaffolder larvae were observed on JASMONATE RESISTANT 1 (JAR1) hemizygous mutant plants (osjar1) of the rice cultivar Nipponbare. The JAR1 gene encodes jasmonate-amido synthetase which catalyzes the biosynthesis of JA-Ile conjugates (Suza and Staswick Citation2008; Shimizu et al. Citation2013). OsJAR1 gene expression and JA-Ile content in the osjar1 hemizygous mutant were lower than those in the wild type in both control and leaffolder larvae-infested plants (Supplemental Figure S1A and S1B). The larvae-induced injury states of wild-type and mutant plants under various P. indica- and larvae-treated conditions were measured according to leaf damage area. In wild-type plants, 43% of the plant population exhibited the most severe damage level (injury score 9) after leaffolder larvae treatment for 48 h. The population with injury score 9 of the osjar1 hemizygous mutant was higher than that of wild-type plants. The population with injury score 9 of P. indica-colonized wild-type plants decreased to 20%. However, the severely damaged population of osjar1plants increased to 30%, even though the plants were colonized with P. indica (Supplemental Figure S2).
Effects of P. indica colonization on enhancing rice leaffolder-induced trypsin inhibitor expression
To further investigate the mechanism of P. indica-enhanced leaffolder resistance, trypsin inhibitor activities of rice leaf tissues were measured. The results showed that trypsin inhibitor levels were obviously induced by leaffolder chewing and were further enhanced in P. indica-colonized plants (). On the other hand, the P. indica-induced trypsin inhibitor expression in larvae-infested plants was repressed by exogenous aspirin (). The results demonstrated that trypsin inhibitor expression enhanced in P. indica-colonized plants under insect herbivore stress conditions was mediated through JA signaling.
Figure 5. Changes in P. indica- and larvae-induced trypsin inhibitor expression in aspirin-treated plants. The 4-leaf-stage plants were pretreated with aspirin (150 μM) for 12 h before larval feeding. Leaf samples were collected for analysis of trypsin inhibitor activity after 48 h of larvae-feeding treatment. Data are shown as the mean ± SE of three independent experiments. The different letters indicate statistically significant differences among group samples (P < 0.05). Control, non-P. indica inoculated and nonlarvae-feeding treatment. LF, rice leaffolder larvae.
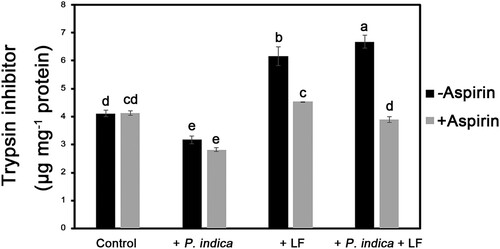
P. indica increased antioxidant capacity of rice plants under herbivore stresses
MDA content is an indicator of oxidative stresses. To monitor the status of oxidative stresses caused by leaffolder larvae chewing, the MDA content of leaf tissues was measured at 2, 24 and 48 h after larvae feeding. MDA content was significantly increased in larval infested leaves, and the herbivore-induced MDA was significantly repressed in P. indica-colonized plants after larvae-infested treatment for 48 h compared with noncolonized plants (). To clarify the regulation of antioxidation in P. indica-colonized plants, the activities of the antioxidant enzymes SOD, APX and GR in leaf tissues were measured. In nonlarvae-infested plants, SOD activity was not obviously promoted by P. indica colonization; however, SOD activity was enhanced 1.8-fold in P. indica-colonized plants compared with noncolonized plants under herbivore-attacking conditions (). Compared with the noncolonized control samples, APX and GR activities respectively increased 2.1- and 1.8-fold in P. indica-colonized plants under nonherbivore-treated conditions (). In leaffolder-infested plants, APX activities in P. indica-inoculated plants were still significantly higher than those in non-P. indica inoculated plants ().
Figure 6. Changes in MDA levels in P. indica-colonized and leaffoler-infested plants. MDA levels in leaves of 4-leaf-stage plants with or without P. indica colonization were determined after rice leaffolder larvae-feeding treatment for 2, 24 and 48 h. Data are the mean ± SE of three independent experiments. The different letters indicate statistically significant differences among group samples (P < 0.05). Control, non-P. indica colonized and nonlarvae-feeding treatment, MDA, malondialdehyde.
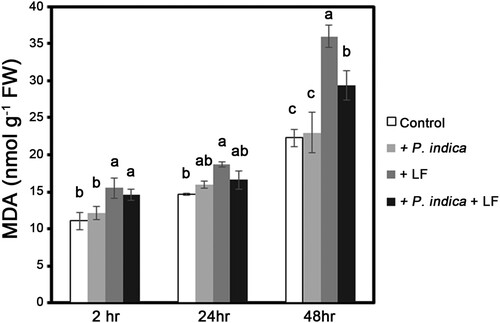
Figure 7. Activities of antioxidant enzymes in P. indica-colonized and leaffolder-infested plants. Superoxide dismutase (SOD), ascorbate peroxidase (APX) and glutathione reductase (GR) activity in leaf samples of the 4-leaf-stage plants with or without P. indica colonization were determined after rice leaffolder larvae-feeding treatment for 48 h. Data are the mean ± SE of three independent experiments. The different letters indicate statistically significant differences among group samples (P < 0.05). Control, non-P. indica colonized and nonlarvae-feeding treatments.
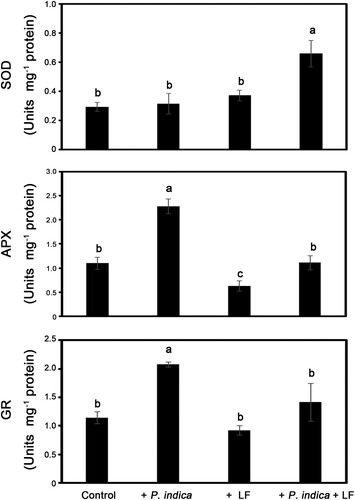
Discussion
P. indica symbiosis reduced pest insect caused plant damage and retarded larvae growth
P. indica effects on promoting plant growth and inducing abiotic stress tolerance have been well exemplified in several studies (Lee et al. Citation2011; Hilbert et al. Citation2012; Jiang et al. Citation2020; Tsai et al. Citation2020). The results presented here showed that both shoot biomass reduction and leaf wilting caused by insect infestation were significantly alleviated in P. indica-colonized plants (A,C). Moreover, insect herbivore-induced photosynthetic system damage was also relatively decreased in P. indica-inoculated plants (E,F). These results indicated that rice plants colonized with P. indica increased resistance against leaffolder larval infestation. P. indica effects on promoting root growth and modifying root architecture have been observed in several plant species (Varma et al. Citation1999; Bakshi et al. Citation2015). Furthermore, P. indica effects on root growth can also occur under some environmental stresses such as water stresses (Saddique et al. Citation2018; Tsai et al. Citation2020). In our study, the root biomass of larvae-infested plants also declined even when the larval attacking site was on leaves (D). However, P. indica-colonized roots exhibited higher biomass than noncolonized plant roots (D). This might have resulted from better growth potential promoted by P. indica colonization, and it could have been due to obtaining more carbon sources from shoots if P. indica-colonized plants had a higher photosynthetic capacity.
Symbiosis with AMF increased the population of Tetranychus urticae on host bean plants (Hoffmann et al. Citation2009). Sebacina vermifera symbiosis repressed defense signals and reduced herbivore resistance (Barazani et al. Citation2005). In contrast, P. indica colonization contributed to increasing insect resistance of rice plants and also impeding growth of leaffolder larvae on P. indica-colonized rice plants ( and ). It was suggested that the effects of endophytic fungi on herbivore resistance are species specific. The impact of symbiosis between P. indica and rice plants affected not only larval growth but also the metamorphosis of rice leaffolders. Both pupal and moth metamorphosis percentage of leaffolders grown on P. indica-colonized rice plants were lower (), which may have caused a decrease in the population of rice leaffolders.
JA signaling involved in P. indica-induced herbivore resistance
JA is a defense hormone induced by several abiotic and biotic stresses (Yang et al. Citation2019). The expression of several genes involved in JA biosynthesis and signaling pathways could be induced by insect stimulation (Ye et al. Citation2012; Wang et al. Citation2013; Chang et al. Citation2019). In Spodoptera mauritia saliva-treated rice leaves, OsJAR1 gene expression and JA-Ile accumulation were increased (Wakuta et al. Citation2011; Fukumoto et al. Citation2013). In our study, the results showed that both JA and JA-Ile levels were significantly increased in leaves after leaffolder infestation (). Larvae-induced JA-Ile levels were promoted in P. indica-colonized rice plants; in contrast, JA levels in P. indica-colonized plants were lower than that in noncolonized plants (). The osjar1 hemizygous mutant contain lower JA-Ile levels compared with wild-type plants (Supplemental Figure S1B). The data in Figure S2 show that the proportion of plants with injured leaf area exceeding 50% of the complete leaf area (injury score 9) caused by larval infestation in the osjar1 hemizygous mutant was higher than that in the wild-type group. The effect of P. indica colonization in reducing larvae-induced leaf damage in osjar1 mutants was lower than that in the wild type. It was suggested that P. indica symbiosis could enhance the insect defense ability of rice plants by modulating leaffolder-stimulated OsJAR1 expression to promote JA conversion to JA-Ile. Cosme et al. (Citation2016) indicated that P. indica colonization could reduce the plant growth inhibition caused by water weevil larvae infestation in rice roots mediated by the interaction of GA and JA signaling. Our study showed that P. indica colonization in rice roots can contribute to increasing the tolerance against leaffolder and reducing leaf tissue damage through JA signaling. Thus, it was suggested that modulation of the JA signaling pathway in the leaf tissues of P. indica-colonized plants could be a systemic response. Endophyte-induced systemic disease resistance was observed in barley (Deshmukh et al. Citation2006). Moreover, in nonlarvae-infested rice plants, it was also demonstrated that P. indica-induced JA accumulation in leaves (). Therefore, P. indica colonization may stimulate priming effects to induce stress-related hormone biosynthesis. The priming effect of P. indica colonization on inducing JA accumulation also occurred in leaf tissues of P. indica-inoculated sweet potato plants (Li et al. Citation2021). In P. indica-inoculated cucumber, increased of pest tolerance against nematodes and salicylic acid accumulation in roots were observed (Atia et al. Citation2020). However, the effects of P. indica on salicylic acid accumulation were not observed in leaf tissues of P. indica-colonized sweet potato (Li et al. Citation2021).
The effects of protease inhibitors on herbivore larval growth have been demonstrated in several plant species such as sweet potato, taro and peanut (Yeh et al. Citation1997; Senthilkumar et al. Citation2010; Lokya et al. Citation2020). In P. indica-colonized rice plants, trypsin inhibitor accumulation stimulated by leaffolder infestation was further promoted (), and leaffolder larvae fed on P. indica-colonized rice performed a lower growth rate (Figures 2A and 4B). It was considered that JA-induced trypsin inhibitor may play a role in the growth-retarding effects of P. indica colonization on larvae. Our study demonstrated that the trypsin inhibitor induced by larval infestation could be repressed by exogenous aspirin (). Moreover, in P. indica-colonized conditions, the reduction in larvae induced trypsin inhibitor expression was also found in osjar1 hemizygous mutants that contained lower JA-Ile (Supplemental Figure S1C). The injury caused by larval infestation in P. indica-colonized osjar1 mutant was more severe than that in P. indica-colonized wild-type rice plants (Supplemental Figure S2). JA-dependent trypsin inhibitor regulations have also been observed in several plant species (Wang et al. Citation2002; Botelho-Júnior et al. Citation2008; Li et al. Citation2017a).
P. indica reduce insect herbivore caused oxidative stresses
Under several biotic or abiotic stresses such as drought, flooding, salt, heavy metals, high light intensity, wounding and pest attack, electron transport chains in the chloroplasts and mitochondria in plant tissues are usually blocked and accompanied by a large amount of ROS production (Hodges et al. Citation2001). MDA is a product of lipid peroxidation and is often used as an indicator of oxidative stresses (Shulaev and Oliver Citation2006; Han et al. Citation2016). Accumulation of MDA in leaffolder larvae-infested leaves was significantly lower in P. indica-colonized rice plants (). It was indicated that symbiotic interaction with P. indica can reduce oxidative stresses induced by larval chewing. The damaging effects of ROS could be prevented by enzymatic and nonenzymatic antioxidants that function as free radical scavengers (Ahmad et al. Citation2010). In barley root systems, P. indica colonization conducted antioxidative and glutathione-ascorbate cycle activities to increase resistance against salt and disease stresses (Waller et al. Citation2005). Li et al. (Citation2021) indicated that P. indica colonization could increase the activities of catalase but not APX, peroxidase and SOD in sweet potato. SOD is considered as the first line of defense to against ROS by removing superoxide ion (O2−) radicals (Alscher et al. Citation2002). The effects of SOD overexpression on increasing antioxidative capacity have been investigated in plants (Li et al. Citation2017b). APX is a cytosolic H2O2-scavenging enzyme that functions as a regulator of ROS levels (Davletova et al. Citation2005). The Arabidopsis apx1 mutant exhibited more severe wound-induced oxidative damage in both chloroplasts and the nucleus than the wild type, and it was indicated that APX plays a role in regulating ROS levels and protecting organelles in wounding-induced oxidative stresses (Maruta et al. Citation2012). Our study showed that APX and SOD activities in P. indica-inoculated plants were significantly higher under herbivore-stressed conditions (). It was indicated that P. indica colonization could promote conversion to O2 and H2O2. Furthermore, the efficiency of H2O2 conversion to H2O was also increased. Increasing SOD and APX activities in P. indica-colonized rice plants might contribute to eliminating ROS and further reducing oxidative damage caused by leaffolders. On the other hand, even though the increase in GR activity was not significant in P. indica-colonized plants under herbivore stress conditions, it was obviously enhanced as well as APX by P. indica colonization in nonlarvae-infested plants. It was suggested that P. indica colonization might induce priming effects against environmental stresses.
In conclusion, P. indica colonization functions to maintain photosynthetic efficiency and plant growth under pest threat conditions. P. indica colonization contributes to increased herbivore resistance of rice plants by mediating JA signaling and reducing oxidative stresses. JA-induced trypsin inhibitors might also play an important role in repressing larval growth and metamorphosis in P. indica-colonized plants.
Supplemental Material
Download Zip (428.2 KB)Disclosure statement
No potential conflict of interest was reported by the author(s).
Additional information
Funding
Notes on contributors
Chih-Yun Chen
Chih-Yun Chen obtained Master’s degree from Department of Agronomy, National Taiwan University.
Po-Hsun Huang
Po-Hsun Huang obtained Master’s degree from Department of Agronomy, National Taiwan University.
Kai-Wun Yeh
Kai-Wun Yeh is professor and senior researcher at National Taiwan University. His research interests focus on insect-resistant mechanism of plants and also flowering physiology.
Shu-Jen Wang
Shu-Jen Wang is professor of crop physiology in Department of Agronomy at National Taiwan University. Her research interests focus on regulatory mechanisms of plant response to biotic and abiotic stresses, and the interaction between beneficial endophytes and plants.
References
- Achatz B, Kogel K, Franken P, Waller F. 2010. Piriformospora indica mycorrhization increases grain yield by accelerating early development of barley plants. Plant Signal Behav. 5:1685–1687.
- Ahmad P, Jaleel CA, Salem MA, Nabi G, Sharma S. 2010. Roles of enzymatic and nonenzymatic antioxidants in plants during abiotic stress. Crit Rev Biotechnol. 30:161–175.
- Alscher RG, Erturk N, Heath LS. 2002. Role of superoxide dismutases (SODs) in controlling oxidative stress in plants. J Exp Bot. 53:1331–1341.
- Ansari MW, Trivedi DK, Sahoo RK, Gill SS, Tuteja N. 2013. A critical review on fungi mediated plant responses with special emphasis to Piriformospora indica on improved production and protection of crops. Plant Physiol Biochem. 70:403–410.
- Atia MAM, Abdeldaym EA, Abdelsattar M, Ibrahim DSS, Saleh I, Elwahab MA, Osman GH, Arif IA, Abdelaziz ME. 2020. Piriformospora indica promotes cucumber tolerance against Root-knot nematode by modulating photosynthesis and innate responsive genes. Saudi J Biol Sci. 27:279–287.
- Bakshi M, Vahabi K, Bhattacharya S, Sherameti I, Varma A, Yeh KW, Baldwin I, Johri AK, Oelmüller R. 2015. WRKY6 restricts Piriformospora indica-stimulated and phosphate-induced root development in Arabidopsis. BMC Plant Biol. 15:305.
- Barazani O, Benderoth M, Groten K, Kuhlemeier C, Baldwin IT. 2005. Piriformospora indica and Sebacina vermifera increase growth performance at the expense of herbivore resistance in Nicotiana attenuata. Oecologia. 146:234–243.
- Bernaola L, Stout MJ. 2019. Effects of arbuscular mycorrhizal fungi on rice-herbivore interactions are soil-dependent. Sci Rep. 9:14037.
- Botelho-Júnior S, Siqueira-Júnior CL, Jardim BC, Machado OLT, Neves-Ferreira AGC, Perales J, Jacinto T. 2008. Trypsin inhibitors in passion fruit (Passiflora f. edulis flavicarpa) leaves: accumulation in response to methyl jasmonate, mechanical wounding, and herbivory. J Agric Food Chem. 56:9404–9409.
- Bütehorn B, Rhody D, Franken P. 2000. Isolation and characterisation of Pitef1 encoding the translation elongation factor EF-1α of the root endophyte Piriformospora indica. Plant Biol. 2:687–692.
- Chang YA, Dai NC, Chen HJ, Tseng CH, Huang ST, Wang SJ. 2019. Regulation of rice sucrose transporter 4 gene expression in response to insect herbivore chewing. J Plant Interact. 14:525–532.
- Chen HJ, Wang SJ, Chen CC, Yeh KW. 2006. New gene construction strategy in T-DNA vector to enhance expression level of sweet potato sporamin and insect resistance in transgenic Brassica oleracea. Plant Sci. 171:367–374.
- Chen YL, Lee CY, Cheng KT, Chang WH, Huang RN, Nam HG, Chen YR. 2014. Quantitative peptidomics study reveals that a wound-induced peptide from PR-1 regulates immune signaling in tomato. Plant Cell. 26:4135–4148.
- Chu C, Lee TM. 1989. The relationship between ethylene biosynthesis and chilling tolerance in seedlings of rice (Oryza sativa). Bot Bull Acad Sinica (Taiwan. 30:263–273.
- Cosme M, Lu J, Erb M, Stout MJ, Franken P, Wurst S. 2016. A fungal endophyte helps plants to tolerate root herbivory through changes in gibberellin and jasmonate signaling. New Phytol. 211:1065–1076.
- Daneshkhah R, Cabello S, Rozanska E, Sobczak M, Grundler FM, Wieczorek K, Hofmann J. 2013. Piriformospora indica antagonizes cyst nematode infection and development in Arabidopsis roots. J Exp Bot. 64:3763–3774.
- Davletova S, Rizhsky L, Liang H, Shengqiang Z, Oliver DJ, Coutu J, Shulaev V, Schlauch K, Mittler R. 2005. Cytosolic ascorbate peroxidase 1 is a central component of the reactive oxygen gene network of Arabidopsis. Plant Cell. 17:268–281.
- Deshmukh S, Hückelhoven R, Schäfer P, Imani J, Sharma M, Weiss M, Waller F, Kogel KH. 2006. The root endophytic fungus Piriformospora indica requires host cell death for proliferation during mutualistic symbiosis with barley. Proc Natl Acad Sci USA. 103:18450–18457.
- Edilberto D. 2013. Standard evaluation system for rice, 5th ed. Los Banos: International Rice Research Institute. p. 31.
- Foster JG, Hess JL. 1980. Responses of superoxide dismutase and glutathione reductase activities in cotton leaf tissue exposed to an atmosphere enriched in oxygen. Plant Physiol. 66:482–487.
- Fukumoto K, Alamgir KM, Yamashita Y, Mori IC, Matsuura H, Galis I. 2013. Response of rice to insect elicitors and the role of OsJAR1 in wound and herbivory-induced JA-Ile accumulation. J Integr Plant Biol. 55:775–784.
- Gill SS, Tuteja N. 2010. Reactive oxygen species and antioxidant machinery in abiotic stress tolerance in crop plants. Plant Physiol Biochem. 48:909–930.
- Guo H, Glaeser SP, Alabid I, Imani J, Haghighi H, Kämpfer P, Kogel KH. 2017. The abundance of endofungal bacterium Rhizobium radiobacter (syn. Agrobacterium tumefaciens) increases in its fungal host Piriformospora indica during the tripartite Sebacinalean symbiosis with higher plants. Front Microbiol. 8:629.
- Han Y, Lei W, Wen L, Hou M. 2015. Silicon-mediated resistance in a susceptible rice variety to the rice leaf folder, Cnaphalocrocis medinalis Guenée (Lepidoptera: Pyralidae). PLoS ONE. 10:e0120557.
- Han Y, Li P, Gong S, Yang L, Wen L, Hou M. 2016. Defense responses in rice induced by silicon amendment against infestation by the leaf folder Cnaphalocrocis medinalis. PLoS ONE. 11:e0153918.
- Heath RL, Packer L. 1968. Photoperoxidation in isolated chloroplasts: I. Kinetics and stoichiometry of fatty acid peroxidation. Arch Biochem Biophys. 125:189–198.
- Hilbert M, Voll LM, Ding Y, Hofmann J, Sharma M, Zuccaro A. 2012. Indole derivative production by the root endophyte Piriformospora indica is not required for growth promotion but for biotrophic colonization of barley roots. New Phytol. 196:520–534.
- Hill DS. 1983. Agricultural insect pests of the tropics and their control. Cambridge, MA, USA: Cambridge University Press.
- Hill T, Kafer E. 2001. Improved protocols for Aspergillus minimal medium: trace element and minimal medium salt stock solutions. Fungal Genet Newsl. 48:20–21.
- Hodges DM, Forney CF, Wismer WV. 2001. Antioxidant responses in harvested leaves of two cultivars of spinach differing in senescence rates. J Am Soc Hortic Sci. 126:611–617.
- Hoffmann D, Vierheilig H, Riegler P, Schausberger P. 2009. Arbuscular mycorrhizal symbiosis increases host plant acceptance and population growth rates of the two-spotted spider mite Tetranychus urticae. Oecologia. 158:663–671.
- Huot B, Yao J, Montgomery BL, He SY. 2014. Growth–defense tradeoffs in plants: a balancing act to optimize fitness. Mol Plant. 7:1267–1287.
- Jiang W, Pan R, Wu C, Xu L, Abdelaziz ME, Oelmüller R, Zhang W. 2020. Piriformospora indica enhances freezing tolerance and post-thaw recovery in Arabidopsis by stimulating the expression of CBF genes. Plant Signal Behav. 15:1745472.
- Jogawat A, Vadassery J, Verma N, Oelmüller R, Dua M, Nevo E, Johri AK. 2016. PiHOG1, a stress regulator MAP kinase from the root endophyte fungus Piriformospora indica, confers salinity stress tolerance in rice plants. Sci Rep. 16(6):36765.
- Koiwa H, Bressan RA, Hasegawa PM. 1997. Regulation of protease inhibitors and plant defense. Trends Plant Sci. 2:379–384.
- Lata R, Chowdhury S, Gond SK, Jr WJ. 2018. Induction of abiotic stress tolerance in plants by endophytic microbes. Lett Appl Microbiol. 66:268–276.
- Lee YC, Johnson JM, Chien CT, Sun C, Cai D, Lou B, Oelmüller R, Yeh KW. 2011. Growth promotion of Chinese cabbage and Arabidopsis by Piriformospora indica is not stimulated by mycelium-synthesized auxin. Mol Plant Microbe Interact. 24:421–431.
- Li Q, Kuo YW, Lin KH, Huang W, Deng C, Yeh KW, Chen SP. 2021. Piriformospora indica colonization increases the growth, development, and herbivory resistance of sweet potato (Ipomoea batatas L.). Plant Cell Rep. 40:339–350.
- Li R, Wang M, Wang Y, Schuman MC, Weinhold A, Schäfer M, Jiménez-Alemán GH, Barthel A, Baldwin IT. 2017a. Flower-specific jasmonate signaling regulates constitutive floral defenses in wild tobacco. Proc Natl Acad Sci USA. 114:E7205–E7214.
- Li Z, Han X, Song X, Zhang Y, Jiang J, Han Q, Liu M, Qiao G, Zhuo R. 2017b. Overexpressing the Sedum alfredii Cu/Zn superoxide dismutase increased resistance to oxidative stress in transgenic Arabidopsis. Front Plant Sci. 8:1010.
- Liu H, Senthilkumar R, Ma G, Zou Q, Zhu K, Shen X, Tian D, Hua MS, Oelmüller R, Yeh KW. 2019. Piriformospora indica-induced phytohormone changes and root colonization strategies are highly host-specific. Plant Signal Behav. 14:1632688.
- Liu X, Li J, Xu L, Wang Q, Lou Y. 2018. Expressing OsMPK4 impairs plant growth but enhances the resistance of rice to the striped stem borer Chilo suppressalis. Int J Mol Sci. 19:1182.
- Lokya V, Swathi M, Mallikarjuna N, Padmasree K. 2020. Response of midgut trypsin- and chymotrypsin-like proteases of Helicoverpa armigera larvae upon feeding with peanut BBI: biochemical and biophysical characterization of PnBBI. Front Plant Sci. 11:266.
- Lu J, Robert CAM, Riemann M, Cosme M, Mène-Saffrané L, Massana J, Stout MJ, Lou Y, Gershenzon J, Erb M. 2015. Induced jasmonate signaling leads to contrasting effects on root damage and herbivore performance. Plant Physiol. 167:1100–1116.
- Lyons R, Manners JM, Kazan K. 2013. Jasmonate biosynthesis and signaling in monocots: a comparative overview. Plant Cell Rep. 32:815–827.
- Maruta T, Inoue T, Noshi M, Tamoi M, Yabuta Y, Yoshimura K, Ishikawa T, Shigeoka S. 2012. Cytosolic ascorbate peroxidase 1 protects organelles against oxidative stress by wounding- and jasmonate-induced H2O2 in Arabidopsis plants. Biochim Biophys Acta. 20:1901–1907.
- Nakano Y, Asada K. 1981. Hydrogen peroxide is scavenged by ascorbate-specific peroxidase in spinach chloroplasts. Plant Cell Physiol. 22:867–880.
- Orozco-Cardenas M, Ryan CA. 1999. Hydrogen peroxide is generated systemically in plant leaves by wounding and systemin via the octadecanoid pathway. Proc Natl Acad Sci USA. 96:6553–6557.
- Orozco-Cárdenas ML, Narváez-Vásquez J, Ryan CA. 2001. Hydrogen peroxide acts as a second messenger for the induction of defense genes in tomato plants in response to wounding, systemin, and methyl jasmonate. Plant Cell. 13:179–191.
- Padmavathi Ch, Katti G, Padmakumari AP, Voleti SR, Subba Rao LV. 2013. The effect of leaffolder Cnaphalocrocis medinalis (Guenee) [Lepidoptera: Pyralidae] injury on the plant physiology and yield loss in rice. J Appl Entomol. 137:249-256.
- Pan Z, Camara B, Gardner HW, Backhaus RA. 1998. Aspirin inhibition and acetylation of the plant cytochrome P450, allene oxide synthase, resembles that of animal prostaglandin endoperoxide H synthase. J Biol Chem. 273:18139–18145.
- Paoletti F, Aldinucci D, Mocali A, Caparrini A. 1986. A sensitive spectrophotometric method for the determination of superoxide dismutase activity in tissue extracts. Anal Biochem. 154:536–541.
- Real-Santillán RO, Del-Val E, Cruz-Ortega R, Contreras-Cornejo HÁ, González-Esquivel CE, Larsen J. 2019. Increased maize growth and P uptake promoted by arbuscular mycorrhizal fungi coincide with higher foliar herbivory and larval biomass of the Fall Armyworm Spodoptera frugiperda. Mycorrhiza. 29:615–622.
- Riemann M, Riemann M, Takano M. 2008. Rice JASMONATE RESISTANT 1 is involved in phytochrome and jasmonate signaling. Plant Cell Environ. 31:783–792.
- Rizzo E, Sherman T, Manosalva P, Gomez SK. 2020. Assessment of local and systemic changes in plant gene expression and aphid responses during potato interactions with arbuscular mycorrhizal fungi and potato aphids. Plants (Basel). 9:82.
- Saddique MAB, Ali Z, Khan AS, Rana IA, Shamsi IH. 2018. Inoculation with the endophyte Piriformospora indica significantly affects mechanisms involved in osmotic stress in rice. Rice. 11:34.
- Schmelz EA, Alborn HT, Tumlinson JH. 2003. Synergistic interactions between volicitin, jasmonic acid and ethylene mediate insect-induced volatile emission in Zea mays. Plant Physiol. 117:403–412.
- Schoenherr AP, Rizzo E, Jackson N, Manosalva P, Gomez SK. 2019. Mycorrhiza-induced resistance in potato involves priming of defense responses against cabbage looper (Noctuidae: Lepidoptera). Environ Entomol. 48:370–381.
- Senthilkumar R, Cheng CP, Yeh KW. 2010. Genetically pyramiding protease-inhibitor genes for dual broad-spectrum resistance against insect and phytopathogens in transgenic tobacco. Plant Biotechnol J. 8:65–75.
- Shamsi TN, Parveen R, Fatima S. 2016. Characterization, biomedical and agricultural applications of protease inhibitors: A review. Int J Biol Macromol. 91:1120–1133.
- Sherameti I, Tripathi S, Varma A, Oelmüller R. 2008. The root-colonizing endophyte Pirifomospora indica confers drought tolerance in Arabidopsis by stimulating the expression of drought stress–related genes in leaves. Mol Plant Microbe Interact. 21:799–807.
- Shimizu T, Miyamoto K, Miyamoto K, Minami E, Nishizawa Y, Iino M, Nojiri H, Yamane H, Okada K. 2013. OsJAR1 contributes mainly to biosynthesis of the stress-induced jasmonoyl-isoleucine involved in defense responses in rice. Biosci Biotechnol Biochem. 77:1556–1564.
- Shulaev V, Oliver DJ. 2006. Metabolic and proteomic markers for oxidative stress. New tools for reactive oxygen species research. Plant Physiol. 141:367–372.
- Singh PK, Nag A, Arya P, Kapoor R, Singh A, Jaswal R, Sharma TR. 2018. Prospects of understanding the molecular biology of disease resistance in rice. Int J of Mol Sci. 19:1141.
- Song YY, Ye M, Li CY, Wang RL, Wei XC, Luo SM, Zeng RS. 2013. Priming of anti-herbivore defense in tomato by arbuscular mycorrhizal fungus and involvement of the jasmonate pathway. J Chem Ecol. 9:1036–1044.
- Sun C, Johnson JM, Cai D, Sherameti I, Oelmüller R, Lou B. 2010. Piriformospora indica confers drought tolerance in Chinese cabbage leaves by stimulating antioxidant enzymes, the expression of drought-related genes and the plastid-localized CAS protein. J Plant Physiol. 167:1009–1017.
- Suza WP, Staswick PE. 2008. The role of JAR1 in Jasmonoyl-L: -isoleucine production during Arabidopsis wound response. Planta. 227:1221–1232.
- Tsai HJ, Shao KH, Chan MT, Cheng CP, Yeh KW, Oelmüller R, Wang SJ. 2020. Piriformospora indica symbiosis improves water stress tolerance of rice through regulating stomata behavior and ROS scavenging systems. Plant Signal. Behav. 15:1722447.
- Upadhyay RK, Mattoo AK. 2018. Genome-wide identification of tomato (Solanum lycopersicum L.) lipoxygenases coupled with expression profiles during plant development and in response to methyl-jasmonate and wounding. J Plant Physiol. 231:318–328.
- Varma A, Verma S, Sudha SN, Bütehorn B, Franken P. 1999. Piriformospora indica, a cultivable plant-growth-promoting root endophyte. Appl Environ Microbiol. 65:2741–2744.
- Verma S, Varma A, Rexer KH, Hassel A, Kost G, Sarbhoy A, Bisen P, Bütehorn Mycologia B, Franken P. 1998. Piriformospora indica, gen. et sp. nov., a new root-colonizing fungus. Mycologia. 90:896–903.
- Vos IA, Verhage A, Schuurink RC, Watt LG, Pieterse CMJ, Van Wees SCM. 2013. Onset of herbivore-induced resistance in systemic tissue primed for jasmonate-dependent defenses is activated by abscisic acid. Front Plant Sci. 4:539.
- Wakuta S, Suzuki E, Saburi W, Matsuura H, Nabeta K, Imai R, Matsui H. 2011. OsJAR1 and OsJAR2 are jasmonyl-l-isoleucine synthases involved in wound- and pathogen-induced jasmonic acid signalling. Biochem Biophy Res Commun. 409:634–639.
- Waller F, Achatz B, Baltruschat H, Fodor J, Becker K, Fischer M, Heier T, Hückelhoven R, Neumann C, Wettstein D, et al. 2005. The endophytic fungus Piriformospora indica reprograms barley to salt-stress tolerance, disease resistance, and higher yield. Proc Natl Acad Sci USA. 102:13386–13391.
- Wang Q, Li J, Hu L, Zhang T, Zhang G, Lou Y. 2013. OsMPK3 positively regulates the JA signaling pathway and plant resistance to a chewing herbivore in rice. Plant Cell Rep. 32:1075–1084.
- Wang SJ, Ho CH, Chen HW. 2011a. Rice develop wavy seminal roots in response to light stimulus. Plant Cell Rep. 30:1747–1758.
- Wang SJ, Lan YC, Chen SF, Chen YM, Yeh KW. 2002. Wound-response regulation of the sweet potato sporamin gene promoter region. Plant Mol Biol. 48:223–231.
- Wang X, Hu L, Zhou G, Cheng J, Lou Y. 2011b. Salicylic acid and ethylene signaling pathways are involved in production of rice trypsin proteinase inhibitors induced by the leaf folder Cnaphalocrocis medinalis (Guenée). Chi Sci Bull. 56:2351–2358.
- White JF, Kingsley KL, Zhang Q, Verma R, Obi N, Dvinskikh S, Elmore MT, Verma SK, Gond SK, Kowalski KP. 2019. Review: endophytic microbes and their potential applications in crop management. Pest Manag Sci. 75:2558–2565.
- Yang J, Duan G, Li C, Liu L, Han G, Zhang Y, Wang C. 2019. The crosstalks between jasmonic acid and other plant hormone signaling highlight the involvement of jasmonic acid as a core component in plant response to biotic and abiotic stresses. Front Plant Sci. 10:1349.
- Ye M, Luo SM, Xie JF, Li YF, Xu T, Liu Y, Song YY, Zhu-Salzman K, Zeng RS. 2012. Silencing COI1 in rice increases susceptibility to chewing insects and impairs inducible defense. PLoS ONE. 7:e36214.
- Yeh KW, Lin MI, Tuan SJ, Chen YM, Lin CJ, Kao SS. 1997. Sweet potato (Ipomoea batatas) trypsin inhibitors expressed in transgenic tobacco plants confer resistance against Spodoptera litura. Plant Cell Rep. 16:696–699.
- Ziegler J, Keinänen M, Baldwin IT. 2001. Herbivore-induced allene oxide synthase transcripts and jasmonic acid in Nicotiana attenuata. Phytochemistry. 58:729–738.