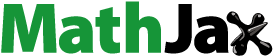
Abstract
The increasing nanomedicine usage has raised concerns about their possible impact on human health. Present evaluation strategies for nanomaterials rely on a case-by-case hazard assessment. They take into account material properties, biological interactions, and toxicological responses. Authorities have also emphasized that exposure route and intended use should be considered in the safety assessment of nanotherapeutics. In contrast to an individual assessment of nanomaterial hazards, we propose in the present work a novel and unique evaluation strategy designed to uncover potential adverse effects of such materials. We specifically focus on spherical engineered nanoparticles used as parenterally administered nanomedicines. Standardized assay protocols from the US Nanotechnology Characterization Laboratory as well as the EU Nanomedicine Characterisation Laboratory can be used for experimental data generation. We focus on both cellular uptake and intracellular persistence as main indicators for nanoparticle hazard potentials. Based on existing regulatory specifications defined by authorities such as the European Medicines Agency and the United States Food and Drug Administration, we provide a robust framework for application-oriented classification paired with intuitive decision making. The Hazard Evaluation Strategy (HES) for injectable nanoparticles is a three-tiered concept covering physicochemical characterization, nanoparticle (bio)interactions, and hazard assessment. It is cost-effective and can assist in the design and optimization of nanoparticles intended for therapeutic use. Furthermore, this concept is designed to be adaptable for alternative exposure and application scenarios. To the knowledge of the authors, the HES is unique in its methodology based on exclusion criteria. It is the first hazard evaluation strategy designed for nanotherapeutics.
1. Introduction
Engineered nanoparticles (ENP) were first introduced to the market as pharmaceutical indications in 1957 and 1959, when iron dextran and sucrose products of Sanofi Aventis and Vifor were approved for the intravenous treatment of iron deficiency in patients with chronic kidney disease. Since the approval of the anti-cancer drug Doxil®/Caelyx® by the United States Food and Drug Administration (FDA) in 1995, the number of yearly approved ENP formulations designed for medical purposes are steadily increasing (Etheridge et al. Citation2013; Pelaz et al. Citation2017; Weissig, Pettinger, and Murdock Citation2014; Witzigmann et al. Citation2015). Today, it is well accepted that ENP have an undisputable potential as drug carriers (Bozzuto and Molinari Citation2015; Coti et al. Citation2009; Puri et al. Citation2009), imaging agents (Arap et al. Citation2013; Toy et al. Citation2014; Unterweger et al. Citation2017), vaccines (Gregoriadis Citation1985; Gregory, Titball, and Williamson Citation2013; Zhao et al. Citation2014), and theranostic tools (Ambrogio et al. Citation2011; Elgqvist Citation2017; Xie, Lee, and Chen Citation2010). Nevertheless, ENP are not risk-free substances and safety concerns towards these novel formulations have to be addressed (Fadeel Citation2012; Wolfram et al. Citation2015). ENP may induce adverse side effects, which is not the case for the bulk chemicals they are composed of (Nel et al. Citation2006; Shvedova, Kagan, and Fadeel Citation2010; Singh Citation2015; Yildirimer et al. Citation2011). For example, physicochemical characteristics such as particle size, shape, and surface properties (e.g. charge) have proven to influence the toxicological profile of ENP heavily regardless of their chemical composition (Berger Citation2009; Buzea, Pacheco, and Robbie Citation2007; Park et al. Citation2011). It is therefore generally believed that ENP cannot be regulated in the same way as bulk chemicals (Feliu and Fadeel Citation2010). In particular, a more detailed understanding of how and why nanoparticles interact with biological systems has to be developed (Dusinska et al. Citation2009; Guadagnini et al. Citation2015; Seaton et al. Citation2010). However, due to the shortage of nano-specific safety guidelines, nanomedicines are not regulated differently from ‘ordinary’ pharmaceuticals up to date. The awareness of nanoparticle toxicity has sensitized researchers and authorities alike to address these concerns and in turn has led to an increasing interest in phenomena related to ‘nanotoxicology’ (Gallud and Fadeel Citation2015; Gebel et al. Citation2014; Krug Citation2014).
Over the past decades, a plethora of research has been conducted on various ENP to better understand how and why nanoparticles are potentially toxic. It was found that nanoparticles entering an organism start to interact with their surroundings immediately (Kettiger et al. Citation2013; Unfried et al. Citation2007; Wu and Lu Citation2016). The nature of these interactions is dependent on the physicochemical identity of the nanoparticles, their surface properties, as well as the environment they encounter (Figure 1)(Fubini, Ghiazza, and Fenoglio Citation2010; Joris et al. Citation2016; Kim et al. Citation2015; Yang et al. Citation2017).
Today, it is known that many ENP are readily taken up by numerous cell types and have the ability to cross intra-cellular barriers as well as inter-cellular spaces (Guarnieri et al. Citation2014; Saraiva et al. Citation2016; Shimizu, Nakamura, and Watano Citation2016). Depending on type, dose, and incubation time, ENP can cause, e.g. the generation of reactive oxygen species, activate the complement system, or disrupt the functionality of membranes and cellular barriers (Guo et al. Citation2015; Moghimi and Simberg Citation2017; Sayes, Banerjee, and Romoser Citation2009; Siegrist et al. Citation2017). These actions lead to acute or chronic damage in the organism culminating in serious outcomes such as inflammation, gene aberrations, and severe organ damage (Nemmar et al. Citation2016; Vermeij et al. Citation2015; Wen et al. Citation2017). Yet – albeit all the efforts of elucidating nanotoxicological patterns – no standardized procedures exist to uniformly determine the safety of ENP. Ongoing debates about general definitions, ideal test systems, or how to group and classify ENP prevent the harmonization of existing safety and testing guidelines (Bleeker et al. Citation2013; Mühlebach, Borchard, and Yildiz Citation2015; Scott-Fordsmand et al. Citation2014; Whitesides Citation2003). Complicating this matter further, a number of clinical applications (e.g. in oncology) rely explicitly on cytotoxic effects induced by nanomedicines. Here, toxic effects are thus part of their mode of action (MoA) (Bao et al. Citation2015; Hung et al. Citation2016). Today, finding a consensus on how to evaluate the safety of ENP is one of the most controversially discussed topics among researchers (Nel et al. Citation2006), regulatory bodies (Stone et al. Citation2014), and authorities (van Teunenbroek Citation2017) alike.
The term nanotoxicology is generally defined as ‘[…] the study of the adverse effects of engineered nanomaterials (ENM) on living organisms and the ecosystems, including the prevention and amelioration of such adverse effects. […]’ (Oberdörster Citation2010). This definition comprises environmental safety as well as adverse drug effects and consumer health thereby targeting every possible nanomaterial (including ENP). In contrast to this highly condensed and straightforward definition, the process of studying, preventing, or minimizing adverse effects caused by nanomaterials is very complex. So far it has not been possible to reach a consensus on how the safety on nanomaterials should be assessed using efficient methods and protocols, which are accepted by regulatory bodies. The reasons are manyfold: lack of defined reference material to benchmark the outcome of the tested material, lack of consensus on predictive endpoints for human toxicity, technical limitations of the tests for ENP (e.g. interference), and batch-to-batch differences due to insufficient control over the manufacturing process. For the past two decades, many government and non-government associations have tried to work out how to tackle this problem. Resulting from their work, the proposal to group or categorize nanomaterials for downstream risk and safety assessments has found large appreciation (Dekkers et al. Citation2016; Fadeel, Pietroiusti, and Shvedova Citation2017; Oomen et al. Citation2015). How to group nanomaterials will mostly be determined by their toxicological profile or the underlying mechanisms thereof. However, it is at present still common to evaluate the safety of nanomaterials on a case-by-case basis due to the lack of standardized experimental data needed for establishing a grouping strategy. This is a time-consuming and costly procedure, which is especially undesirable in medicinal research. The most common grouping strategies (please refer to the following reflection papers of the European Medicines Agency (EMA) as provided in the References section: Data requirements for intravenous iron-based nano-colloidal products developed with reference to an innovator medicinal product; Joint MHLW/EMA reflection paper on the development of block copolymer micelle medicinal products;Reflection paper on surface coatings: general issues for consideration regarding parenteral administration of coated nanomedicine products; Reflection paper on the data requirements for intravenous liposomal products developed with reference to an innovator liposomal product; Reflection paper on nanotechnology-based medicinal products for human use.) are based on categorizing nanomaterials according to physicochemical properties, nanoparticle-cell interactions, exposure threats, or endpoint toxicity (Arts et al. 2015; Sayes, Smith, and Ivanov Citation2013; Simkó, Tischler, and Mattsson Citation2015). These strategies all target different aspects of nanomaterial toxicity and can provide vital information on the nature of nanomaterial-based toxicity. These approaches are often tailored to fit specific exposure risk scenarios of involuntary inhalation or voluntary oral ingestion (Bolt Citation2014; Gebel et al. Citation2014; Hadrup et al. Citation2015). Intentional therapeutic exposure upon injection on the other hand is only rarely referred to or even specifically ruled out of present grouping concepts (Arts et al. 2015). Yet, there are several reasons why the definition of such a strategy would be highly beneficial. First, for medical applications, where ENP formulations offer increasing therapeutic options, injection is the preferred route of administration. This route of administration leads to a defined and measurable exposure in contrast to inhalation or ingestion scenarios. Second, with the correct choice of biological models, it is possible to distinguish between intentional pharmacological effects (i.e. cytotoxicity as a MoA in cancer) and off-target effects. Third, with more than 75 FDA approved ENP, over 100 in clinical trials and hundreds more in research (Bobo et al. Citation2016; Witzigmann et al. Citation2015), the need for nano-specific regulation and safety assessment strategies for medical candidates is obvious.
2. Aim
It is the aim of the present work to provide a proposal for a novel hazard evaluation strategy to determine the safety of ENP designed for the use as injectable nano-pharmaceutics (). It is important to note that inhalable and ingestible dosage forms were explicitly excluded from this study. Inhalables are based on particle size distributions that exceed the nano-range (Brown et al. Citation2013). Oral nanomedicines mostly consist of nanocrystals, which enhance solubility of poorly soluble drugs (Etheridge et al. Citation2013; Junghanns and Müller Citation2008). As they dissolve rapidly upon contact with gastric or intestinal fluids, these materials do not penetrate the body in the form of nanoparticles. Here, we propose a stepwise approach to assess the safety of nanomedicines (). First, ENP are required to meet specific physicochemical properties to allow for their use as injectable nanoparticles (iNP). Second, a novel classification system divides selected candidates into hazard classes based on cellular uptake and intracellular persistence. Third, ENP are evaluated based on their safety classification using a distinct set of standardized toxicological assays as conducted by the US Nanotechnology Characterization Laboratory and European Nanomedicine Characterization Laboratory (USNCL and EUNCL, respectively). The US- and EUNCL assist the respective regulatory agencies (EMA and FDA) during the approval process of medical applications containing nanomaterials. Finally, the retrieved information serves a multitude of downstream processes such as safe-by-design, safety predictions, risk assessment, data mining, and grouping.
Figure 1. Nanosafety. Together with the biological system, the surface properties and physicochemical identity define the toxic potential of any engineered nanoparticle.

Figure 2. Hazard Evaluation System for Injectable Nanoparticles (HES). The HES hazard evaluation strategy is based on a three-tiered approach, which combines physicochemical characterization of engineered nanoparticles (ENP), ENP interaction, and hazard assessment. This test strategy can be used to (i) determine if a given ENP qualifies as a parentally administered medical application and (ii) identify potential safety issues of injected nanomedicines at early stages of their development. Furthermore, linking the findings from tiers 2 and 3 to the physicochemical properties determined in tier 1 can serve as the basis for in vivo testing strategies, risk assessment strategy set up and future grouping criteria. This will help to design and optimize ENP through safe-by-design principles and support personalized medicine initiatives.

The three-tiered strategy allows us to identify suitable ENP for injection in an application-oriented and effective manner. Its structure could aid in minimizing the risk of adverse responses when moving from in vitro to in vivo testing as well as costly failures during later stages of their development. Our strategy is thus based on the International Organization of Standardization (ISO) definition of the term ‘nanoparticle’ and takes up a similar classification approach as used, for example, in the Biopharmaceutics Classification System (BCS) to estimate the oral bioavailability of drugs (Amidon et al. Citation1995; ISO Citation2015). The concept uses standardized testing protocols from the USNCL and EUNCL to specifically assess the hazardous potential of ENP. Ultimately, the HES approach should promote an application-oriented decision-making process. It will contribute to informed and effective ENP hazard assessment and can be combined with future grouping strategies for read-across purposes.
3. The hazard evaluation system for injectable nanoparticles (HES)
Approved nanomedicines designed for parenteral administration are very diverse with respect to their chemical identity, size, geometry, surface modifications, ζ-potential, and intended clinical uses. Very often, these formulations share certain physicochemical properties. They are mostly spherical or display an aspect ratio (AR) ≤ 3:1, have particle diameters below one micron (Buzea et al. Citation2007), and are stable as dispersions. However, this knowledge does not directly provide any information on the behavior or safety of an ENP after injection. ENP may provoke toxicity in a dose- and time-dependent manner upon interaction with biological systems. This includes, for example, recognition, phagocytosis or endocytosis, and processing of the immune system (macrophages) on a cellular level (Bartneck et al. Citation2010b; Sabella et al. Citation2014; Tiwari, Takashi, and Behari Citation2010; Weissleder, Nahrendorf, and Pittet Citation2014). On a whole-organism level, such interactions may lead to augmented circulation time, accumulation, retarded clearance, off-target interactions, organ damage, and chronic adverse effects. We strongly believe that the two key factors, directly providing preliminary information on these biological interactions and the ensuing in vivo consequences, are cellular uptake (Oh and Park Citation2014; Yameen et al. Citation2014) and intracellular persistence (Laux et al. Citation2017; Lee et al. Citation2013). High uptake and high persistency may trigger inflammatory events (Fadeel et al. Citation2017; Kennedy, Wilson, and Barakat Citation2009). Low quantities of ENP uptake by cells other than macrophages and low intracellular persistency over time is indicative of limited biological interactions. In the latter case, phagocytosis by macrophages (Bartneck et al. Citation2010a) or extracellular events such as platelet aggregation or complement activation should be closely monitored. Due to the possibility of linking physicochemical properties with nanoparticle-cell interactions in vitro, the risk of provoking adverse effects in vitro and in vivo (e.g. toxicity or elimination) can be minimized significantly.
The Hazard Evaluation System for Injectable Nanoparticles (HES) was designed as a concept to specifically test the suitability of ENP as injectable pharmaceutics. The three tiers – as shown graphically in – are physicochemical characterization (1), ENP interaction (2), and hazard assessment (3).
The ENP are physicochemically characterized before progressing to in vitro toxicity studies. Following the characterization, cellular uptake and intracellular persistence of the formulations are determined to categorize them into hazard groups for more detailed hazard assessment. Dedicated hazard assessment processes designed for every group ensure the recognition of hazardous potential associated to a pharmaceutical candidate. Every tier includes specific assays, measuring techniques, controls and definitions that are discussed in detail in the following sections. Formulations failing to meet the requirements of tier 1 to tier 3 are not further considered in the HES approach and are required to proceed in case-by-case evaluations. Such formulations are not necessarily considered unfit as injectable pharmaceutics; however, due to their innovation or unconventional characteristics represent classes of formulations where vital information is still lacking. Thus, the safety of these candidates must be evaluated case-by-case until enough data are acquired in order to expand the HES framework or separately establish a novel testing strategy. An important component of the proposed concept is the retrospective analysis of results (decision-making and in vivo analysis, ). Data mining and data analysis will offer the possibility to perform informed safety predictions and to implement grouping and modeling strategies. Such a rational approach will be the basis for future safe-by-design strategies.
3.1. Tier 1: Physicochemical characterization
In the first tier of the HES, the candidate materials enter a defined physicochemical characterization cascade (see flowchart ). The cascade serves as a systematic preselection sequence, in which ENP are tested according to six criteria: chemical identity, ζ-potential (zeta potential), aqueous stability, shape, size and size distribution, and contamination. Depending on the outcome of this initial screen, an ENP either qualifies to be further investigated in the HES or must yet undergo a ‘case-by-case’ safety assessment, as mentioned earlier. The chemical identity, ζ-potential, and aqueous stability of an ENP are not directly a safety issue in most cases. Nevertheless, knowing these three parameters is a prerequisite for their further experimental assessment. Having this knowledge beforehand can give insight into how an ENP sample behaves in dispersion, or in what manner it may interact with buffers or biological fluids. A formulation that fulfills all physicochemical requirements (as summarized in ) also fits the physicochemical definition of an injectable nanoparticle and can therefore enter tier 2 of the HES.
Figure 3. Physico-chemical characterization of nanoparticle properties. The physicochemical characterization of ENP represents tier 1 of the Hazard Evaluation System for Injectable Nanoparticles (HES). After the chemical identity, ζ-potential, and aqueous stability are determined, size, particle size distribution, and purity are examined. In this step-by-step approach, an ENP either moves on through the HES cascade or its safety has to be evaluated separately case-by-case depending on the results obtained during characterization. A candidate passing the entire cascade (Tier 1) qualifies as an injectable nanoparticle according to the HES guideline and moves on to ENP interaction (Tier 2, Figure 4). AR: Aspect Ratio.

Each one of the basic physicochemical properties of ENP should be measured using a standardized protocol. For the HES, we propose to use the written and recommended protocols of either the USNCL or the EUNCL. Both US- and EUNCL work together with the FDA or the European Medicines Agency (EMA), respectively. Furthermore, both NCL institutions do not only act as advisors to regulatory agencies but also provide assistance for small and medium-sized enterprises, spin-offs and newcomers towards nanomaterial characterization and product submission approvals. This assures that the assay protocols are well in-line with current testing standards and are re-evaluated on a regular basis.
In addition, proposals for alternative measurement techniques are provided as well. These techniques are not used by the US- or EUNCL but find much appreciation throughout their respective research fields. Furthermore, not all techniques suggested by the NCLs are available as a standard in every laboratory. References to selected protocols are listed in the appropriate sections of this publication.
3.1.1. Chemical identity
The chemical identity of an ENP should be assessed first as it may have an impact on the setup of further experiments regardless if the ENP is the active agent or its carrier system. Should any chemical contaminations (e.g. synthesis residues) be detected during the assay, it is strongly advised to purify the formulation before advancing further in tier 1 (Garbovskiy Citation2017). Batch-to-batch variability should be held as low as possible and must be recorded for at least three independently manufactured formulations.
Different analysis techniques can be used to gain information on the chemical identity of an ENP. The most commonly used technique is Inductively Coupled Plasma Mass Spectrometry (ICP-MS) (Fabricius et al. Citation2014). With ICP-MS entire samples or single nanoparticles (detection limit in nanogram per liter range) can be analyzed in very short time and high precision. Furthermore, ICP-MS can be coupled to several analytical separation methods whereby speciation and quantification measurements can also be performed. The USNCL provides four different protocols on how to characterize the chemical identity of nanomaterials all including ICP-MS techniques (USNCL-PCC-8, USNCL-PCC-9, USNCL-PCC-11, and USNCL-PCC-14). As an alternative, different techniques including High Performance Liquid Chromatography (HPLC) are also being used to analyze nanomaterials that are mainly carbon, or nonmetal based (Álvarez-Fuentes et al. Citation2012; Itoh et al. Citation2016).
Alternatively, Nanoscale Fourier-Transformation Infrared Spectroscopy (nano-FTIR) can also be used to determine the chemical identity of nanoparticles (Huth et al. Citation2012). Fourier-Transformation Infrared Spectroscopy (FTIR) is commonly used in chemistry to identify chemical structure of materials or to verify the chemical purity of a synthesis product. Nano-FTIR is a combination of FTIR and scattering-type Scanning Near-field Optical Microscopy (s-SNOM) based on Atomic Force Microscopy (AFM) in noncontact mode. The technique allows measurements on ultra-small probe quantities with a spatial resolution of 10 nm–20 nm. Nano-FTIR has already been successfully used to identify polymers and nanocomposites or in investigations regarding the elucidation of secondary protein structures as well as bacterial membranes (Amenabar et al. Citation2013).
Another important technique is the Microscale Thermogravimetric Analysis (µ-TGA). It is used to characterize the surface coating and purity of nanoparticles, especially when they are introduced to a biological environment (Mansfield et al. Citation2014). This method is very sensitive and can be used to determine the amount of coating on the nanoparticle surface. These experiments are done in an inert atmosphere at temperatures over 1000 °C (Dongargaonkar and Clogston Citation2018).
Alternatively, quantitative NMR can be used to characterize nanoparticles to reveal structural and dimensional information. Especially the coatings can be analyzed to explore characteristics like the ligand density on the surface (Guo and Yarger Citation2018).
3.1.2. Aqueous stability and ζ-potential
The lack of aqueous stability provokes agglomeration and sedimentation, which may trigger immune responses such as phagocytosis, generation of reactive oxygen species (ROS), or inflammatory reactions. Moreover, agglomeration-flocculates can sediment and in extreme cases could clot capillaries causing mechanical distress and serious pain. Since there is no generally accepted protocol on the measurement of ENP stability, the authors propose the following: ‘The aqueous stability of ENP should exceed the duration of the longest tests proposed in the tiers of the hazard evaluation strategy (HES). We therefore propose that ENP dispersions should not display any significant changes in particle size, shape, and particle size distribution over the course of at least one week, at a defined pH and at 37 °C using a physiological buffer system.’
However, depending on specific project requirements and application needs, this basic protocol can be adjusted to a specific time frame (e.g. short-term application) or for factors that can affect the stability profile, such as the mechanism of the payload delivery or surface modifications. Furthermore, additional test might be needed to demonstrate prolonged stability during storage at room temperature or at 4 °C in a specific container (e.g. plastic infusion bag or glass vials). It should also be noted that the stability of an ENP may change after dispersion in biological fluids (Aguilar-Castillo et al. Citation2015; Moore et al. Citation2015). Thus, performing stability measurements in biological fluids may yield valuable information as well and should be carried out. Aqueous stability measurements follow the same protocols used for particle size, shape, and particle size distribution. Method descriptions and protocols are covered in detail by the appropriate standardized protocols (see Section ‘3.1 Tier 1: Physicochemical characterization’).
The aqueous stability of any nanomaterial dispersion is often related to the surface charge (ζ-potential) of the nanoparticle. For this reason, ζ-potentials of ENP should be measured prior to analyzing their aqueous stability (Bhattacharjee Citation2016). Formulations that display positive or negative ζ-potentials are generally considered to be stable in water whereas nanoparticles with a ζ-potential near neutral tend to agglomerate (Clogston and Patri Citation2011). It should, however, be noted that highly positive charged particles have the potential to be acute cytotoxic (Dobrovolskaia et al. Citation2012). On the other hand, ζ-potential values directly depend on the pH of nanoparticle dispersions as pH influences protonation and deprotonation of the nanoparticles (Berg et al. Citation2009). Thus, ζ-potentials should always be determined at pH values that resemble the ones of the ENP storage solutions and ideally represent physiological conditions (i.e. ideally above 30 mV or below –30 mV). The protocols ensuring correct measurements of ζ-potentials and pH are referenced as USNCL-PCC-2 and USNCL-PCC-13, respectively. The preferred ζ-potential measuring technique is electrophoretic light scattering (ELS) whereas pH is predominantly measured with semi-micro combination pH electrodes.
3.1.3. Shape
The International Standards Organization (ISO) provides definitions for the terms nanomaterial, nano-object, nanofiber, nanoplatelet, and nanoparticle based on their external and internal dimensions. In this sense, external dimensions describe length, width, and height of a material whereas surface structures (e.g. pores) and internal structures are acknowledged as internal dimensions (ISO Citation2015). As an exclusion criterion, the HES approach considers external dimensions of ENP only, yet taking into account that internal dimensions may well influence stability or the MoA.
As mentioned before, most commercially available nano-pharmaceutics are spherical in shape or have an aspect ratio of ≤3:1. Therefore, only nanoparticles that display an aspect ratio (AR) ≤3:1 are considered for further investigations in the HES. So called high aspect ratio nanomaterials (HARN) (Oberdörster, Stone, and Donaldson Citation2007) are excluded from the process. This includes the fiber-like single or multi-walled carbon nanotubes (SWCNT or MWCNT, respectively). Although these nanomaterials are at present heavily studied, they show typical signs of complications associated to HARN. HARN may inflict mechanical cell or tissue damage often due to rigidity and size (e.g. leading to frustrated phagocytosis). Moreover, their geometry bears a higher risk of recognition by the immune system. Considering these facts, HARN are not ideal nanomaterials for parenteral applications and have not been used as such up to date. Consequently, HARN are also excluded from the HES and must be evaluated on a case-by-case basis.
Transmission Electron Microscopy (TEM) (Pyrz and Buttrey Citation2008), Scanning Electron Microscopy (SEM) (Goldstein et al. Citation2014), and cryoEM (Crawford et al. Citation2011) are commonly used to study the shape of nanoparticles. The chemical identity of a nanoparticle dictates which of these techniques should be applied. The procedures using TEM and SEM can be carried out according to the protocols USNCL-PCC-7 and USNCL-PCC-15, respectively. Additionally, the EUNCL provides a protocol for cryoEM analysis (EUNCL-PCC-20).
3.1.4. Particle size
Particle size is arguably the most important characteristic in the first HES tier since various definitions of the term ‘nanoparticle’ are based entirely on primary particle size. We propose the implementation of a novel size range specifically suiting ENP designed for injection. Such candidates normally cover a size range of 1–500 nm rather than the regulatory definition of 1–1000 nm. Candidates averaging sizes below 1 nm or above 500 nm are subjected to case-by-case evaluations and are excluded from the HES.
We feel that, in this particular case, the novel ENP size range of 1 nm to 500 nm is a valid alternative to existing definitions of the International Standards Organization (ISO), the Organization for Economic Co-operation and Development (OECD), the European Commission, the US Environmental Protection Agency, or regulatory authorities in charge of pharmaceuticals. In many cases, nano-objects are defined by a size of approximately 1– 100 nm (often referred to as the nanoscale) (ISO Citation2015; Krug and Wick Citation2011; Potočnik Citation2011; Serpone and Horikoshi Citation2013). The lower limit of 1 nm was set to specifically exclude single atoms and small atom groups. The upper limit of 100 nm was set to emphasize on the existence of so called ‘quantum size effects’ (Boilard et al. Citation2013; Krug and Wick Citation2011; Zhang et al. Citation2009) often originating from band-gap differences directly influenced by particle size (Chukwuocha, Onyeaju, and Harry Citation2012). Due to their submicron size, ENP may thus display unique characteristics that their bulk counterparts lack completely. The National Nanotechnology Initiative (NNI) states that quantum effects are size driven and in turn govern the behavior and properties of nanomaterials (NSCT/CoT/NSET Citation2014). A number of properties such as fluorescence, electrical conductivity, magnetic permeability, and chemical reactivity are affected when particles stay below the critical size of 100 nm (Navya and Daima Citation2016; Uskoković Citation2013). However, this 100 nm cutoff value remains controversial, for different reasons. Numerous regulatory organizations feel that the present justification of the upper size limit (100 nm) is not scientifically appropriate and should be extended with more qualifiers (such as particle size distribution and spatial dimensions) (Jong Citation2010; Lovestam Citation2010; Potočnik Citation2011). Additionally, it has been acknowledged that health and safety aspects with nanomaterials do not abruptly end at 100 nm (ISO Citation2015). In view of these debates and the growing interest in pharmaceutical and medical nano-applications, the FDA has considered an augmentation of the upper scale limit to 1000 nm (FDA Citation2005). Most other regulatory authorities have also adopted this scale enlargement over the past years. However, the FDA also states that setting the cutoff at one micron is not at all trouble-free as this size incorporates an even greater palette of diverse materials (e.g. pigments, quartz, sand). The FDA, therefore, states that it might be beneficial not to cover all nanomaterials within one definition but rather set definitions according to the applicatory field of the material in question (FDA Citation2005).
Taking these facts into account, the HES size definition for injectable nanoparticles was considered to be appropriate for medical regulation purposes. The lower limit of the HES size definition (i.e. 1 nm) is based on widely accepted nanoparticle definitions from authorities and regulatory bodies whereby atoms and small molecules are excluded. Its upper limit (i.e. 500 nm) newly considers the activity of immune cells and their ubiquitous presence in the vasculature system. It is generally accepted that uptake of intact nanoparticles through cell membranes is mediated by active transport (e.g. receptor mediated endocytosis) (Zhang et al. Citation2009). These uptake routes depend on particle size. Objects smaller than 500 nm are readily internalized via different types of endocytosis (e.g. clathrin versus caveolin-mediated transport) (Champion, Katare, and Mitragotri Citation2007; Kettiger et al. Citation2013). However, objects larger than 500 nm mostly lack this ability and are prone to be predominantly exposed to phagocytosis, which is an immune cell-specific process (Aderem Citation2003). Therefore, limiting ENP size to 500 nm rules out size-dependent involvement of immune cells in cellular uptake of ENP or immune mediated reactions. Finally, since injectables are not designed to trigger phagocytosis, the upper size limit of 500 nm in the HES definition of an iNP was chosen.
Alternatively, some nanoparticles are designed to be fusiogenic (e.g. cationic liposomes). For example, liposomes with integrated lipopeptides in the bilayer were able to fuse with plasma membranes and to empty their cargo into the cytoplasm of their target cells (reviewed by Li et al. (Citation2017)). Under such conditions, active transport will not be involved and the mentioned size definitions have to be adjusted.
Size measurements must be interpreted with caution since the term ‘particle size’ is often used interchangeably to describe the primary particle size as well as the hydrodynamic diameter of an ENP. Primary particle size denominates the true particle diameter, whereas the hydrodynamic diameter takes the first layer of solvent into account as well (Hackley and Clogston Citation2011). Hence, the hydrodynamic diameter should always be larger than the primary particle diameter and is therefore the decisive parameter when it comes to the discussed size limitations. Nevertheless, both parameters should be measured as either one can have an impact at later stages of the HES. The standard methods to measure hydrodynamic diameters of ENP are based on the technique of Dynamic Light Scattering (Kaszuba et al. Citation2008) (DLS, protocol USNCL-PCC-1). Here, Nanoparticle Tracking Analysis (NTA) (Hole et al. Citation2013; Maguire et al. Citation2017; Mehn et al. Citation2017) is frequently used as an alternative method. Atomic Force Microscopy (Rao et al. Citation2007) (AFM, protocol USNCL-PCC-6) and TEM (Pyrz and Buttrey Citation2008) (protocol USNCL-PCC-7) are typically used to determine primary particle sizes. Other techniques that are used to describe primary particle sizes include: Small-Angle X-Ray Scattering (SAXS) (Li, Senesi, and Lee Citation2016; Vippola et al. Citation2016) and electrospray differential mobility analysis (ES-DMA) (Guha et al. Citation2012).
3.1.5. Particle size distribution
The Scientific Committee on Emerging and Newly Identified Health Risks (SCENHIR) sub-categorizes nanoparticles into three categories depending on the range of particle size distributions. In contrast, we propose a more stringent approach. It demands particle size distributions to be as narrow as possible (Jong Citation2010). In physiological buffer, a particle size distribution limit of 20% or a polydispersity index value (PDI) of <0.2 based on an intensity-based Z-average diameter value is proposed. This limit is chosen because a PDI of <0.2 is considered to reflect monodispersity (Panchal et al. Citation2014; Roberson and Weltje Citation2014). However, the viscosity and refractive index of the solvent, ionic strength of the media, temperature, concentration of ENP, handling of ENP (e.g. filtration, sonication) should be considered for comparing different PDI values across studies. It should be noted that a PDI <0.2 for parenteral applications might be smaller than the corresponding value defined for other therapeutic applications. For example, for dermal delivery of liposomes, a PDI <0.3 has been deemed acceptable to reflect monodisperse ENP-drug preparations. In addition, the PDI will change in presence of proteins due to protein adsorption or the presence of protein agglomerates. If the condition of monodispersity is not fulfilled, size fractionation (Akthakul et al. Citation2005; Robertson et al. Citation2016) or filtering (Droppo Citation2006) may be used to narrow down the particle size distribution of particle populations. The method of choice to determine hydrodynamic particle size distributions and polydispersity is DLS according to protocol USNCL-PCC-1. In addition, true particle size distributions of ENP should be confirmed by SEM and TEM (FDA Citation2005; Jong Citation2010; Lovestam Citation2010).
3.1.6. Microbial contamination and endotoxins
A well-known issue with ENP submitted for pharmaceutical or medical approval is purity (Li and Boraschi Citation2016). The presence of impurities is the most common reason regulatory bodies neglect pharmaceutical formulations or applications during the approval sequence. Purity refers to the absence of chemical or biological contamination. As chemical impurities must be checked for and identified previously during the analysis of the chemical identity; this step of the HES focuses on biological contamination (i.e. bacteria, mycoplasma, or endotoxin). The USNCL defines a number of assays to assess contamination levels of nanomaterials. At least endotoxin (USNCL-STE-1.1 until 1.4), bacterial (USNCL-STE-2.1 and 2.2), and mycoplasma contaminations (USNCL-STE-3) should be monitored. Purity is an intrinsic property of any iNP. In case of the presence of contaminants, ENP should be sterilized and depyrogenized by using standard techniques such as autoclaving, filtration, or gamma-irradiation (Li et al. Citation2013; Vetten et al. Citation2014; Zheng et al. Citation2011). If, however, impurities are still detected thereafter, which cannot be removed by a modified production process, the candidate falls out of the HES and must be further assessed in a case-by-case evaluation. Because standard sterilization techniques can influence the stability and physicochemical properties of ENP, it must be ensured that the properties of postprocessed ENP are not significantly changed.
3.1.7. Physicochemical definition of an injectable nanoparticle (iNP)
The physicochemical definition of an iNP takes all the previously discussed issues into account and summarizes tier 1 of the Hazard Evaluation Strategy for Injectable Nanoparticles (HES) as follows: ‘An injectable nanoparticle (iNP) is defined as a nanoparticle measuring between 1 nm to 500 nm in all three external dimensions (height, length, width), whereas the length of the longest to the shortest axis of the nanoparticle do not differ by more than three-fold (aspect ratio 3:1). Additionally, iNP particle size distributions should be below 20% (polydispersity indices of <0.2) as determined in a physiological buffer system. Also, the aqueous stability should not be affected over the course of 7 days when measured at a distinct pH and 37 °C. Finally, an iNP must be endotoxin-free and should not show chemical, bacterial, or mycoplasma contamination.’
3.2. Tier 2: ENP interaction
In the second tier of the HES, a novel classification system divides iNP into four different risk categories depending on two biological readouts, which are linked to particle persistence in biological systems and cellular exposure. In this novel ENP interaction concept (), iNP are grouped according to the criteria of cellular uptake and intracellular persistence. We propose that these two parameters are key for iNP safety in circulation. In the HES plot, the intensity of cellular uptake and intercellular persistence increases from the top left to bottom right quadrant of the plot. This does not generally imply that high uptake and persistence of iNP bear the highest risk for a patient. Depending on the intended use of the iNP, high uptake and persistence are part of the MoA. Yet, the multitude of possible adverse effects is augmented when uptake and persistence are high. Consequently, iNP grouped into category IV necessitate a more detailed safety assessment as compared to iNP falling into lower risk categories (Category IV < III < II < I).
Figure 4. The HES ENP interaction. The HES ENP interaction divides nanoparticles into four different groups. The grouping strategy is based on cellular uptake and intracellular persistence of an iNP. By monitoring these two parameters, distinct hazard assessment strategies can be designed for individual ENP interaction groups. As a general rule, the higher the category the higher the number of possible hazards and thus the number of required safety assessments. It should be noted that the generation of ROS or an involvement and activation of the immune system has a major impact on the interpretation of the HES classification. For example, humoral responses mediated by the complement system may lead to hemolysis and platelet aggregation. Cellular immune responses may lead to particle depletion.

Neither USNCL nor EUNCL provides standardized protocols for measuring cellular uptake or intercellular persistence as this does not lie in the scope of their work. Therefore, experimental methods are proposed in the following sections to address these issues.
3.2.1. Cellular uptake
The techniques to measure cellular uptake can vary depending on the type of iNP and the availability of analytical technologies. Ideally, confocal-, darkfield-, and EM-microscopy (qualitative assessment) can be combined with flow cytometry, HPLC, single particle or single cell ICP-MS, or a combination of the latter techniques (quantitative assessment). The prerequisite for quantitative assessments is, however, knowing the initial administered dose. After a desired incubation time, the quantity of iNP and their residues is measurable in the supernatant as well as in the cell sample. This enables determining the quantity of iNP taken up. Measurements should be performed at time points 30 min, 4 h, 8 h, and 24 h. The indicator for low cellular uptake would then be defined as any quantity of iNP taken up in the first 8 h of incubation being below a certain cutoff value (percentage of total iNP dose). Alternatively, high cellular uptake levels would be represented by any quantity of iNP taken up in the first 8 h of incubation exceeding that same cutoff value. With this respect, erythrocytes may serve as negative control (minimal uptake) and macrophages as positive control (maximal uptake).
Cellular uptake of iNP should be monitored in the cell type, which is most likely to interact first with iNP after injection. After intravenous injection of iNP, for example, these are vascular endothelial cells as they line the blood vessels and the lymphoid system (Félétou Citation2011). Endothelial cells are known to readily take up nanoparticles (in contrast to, e.g. erythrocytes) (Siegrist et al. Citation2017). This can lead to severe cytotoxic effects that in turn damage the vascular system and potentially the tissue residing behind it, causing complications and pain in the patient. Furthermore, the endothelium acts as a semi-selective barrier and co-controls the migration of white blood cells into and out of the blood stream (Yuan and Rigor Citation2010). Endothelial dysfunction plays, therefore, a pivotal role in vascular diseases (Deanfield et al. Citation2005) and is often regarded as the early trigger of events such as arteriosclerosis (Davignon and Ganz Citation2004). We, therefore, believe that monitoring endothelial uptake of iNP in vitro provides essential information on endocytosis and cellular clearance of iNP. Intended target cells of the iNP should be excluded from these measurements, since the focus of these experiments is an ENP interaction in non-target cells. While the exact structure of the biocorona is controversially discussed (e.g. existence and relevance of a ‘soft’ protein corona), the detailed composition is very variable and highly fluctuating over time even in vitro (Casals et al. Citation2010; Docter et al. Citation2015). Furthermore, recent studies show that proteins adsorbed to nanoparticles in vitro are rapidly exchanged in vivo (Chen et al. Citation2017). Although, in the future, in vitro tests might permit to predict the role and influence of the biocorona in vivo, currently our understanding and ability of extrapolating the impact from in vitro to in vivo is insufficient. Therefore, future tests assessing the biocorona of nanoparticle might complement our test strategy.
For protein interactions of biomedical applied nanoparticles, see Shannahan (Citation2017). This topic is further reviewed by Treuel et al. (Citation2015), Ke et al. (Citation2017), and Neagu et al. (Citation2017).
3.2.2. Intracellular persistence
Intracellular persistence is monitored in the same cell type, preferably even in the same sample, as cellular uptake. The first measurements should be performed 8 h and 24 h after incubation, thereafter once every 24 h for a week. In the sense of a precautionary approach, low intracellular persistency is defined if the amount of iNP taken up after 24 h decreases by 80% until the fifth day of the measurement. High intracellular persistence is given, if less than 80% of the iNP taken up in the first 24 h has been degraded.
Measuring intracellular persistence can either be performed by confocal and EM microscopy or ICP-MS (Lee et al. Citation2013). While microscopy techniques will only yield qualitative results, ICP-MS allows for quantitative analyses. However, cellular samples are destroyed under ICP-MS in contrast to microscopy techniques. It should be emphasized, that intracellular persistence is not equivalent to chemical or colloidal stability as it is being tested for in tier 1 of the HES. Even though the two parameters can certainly be linked, the ability of an organism to degrade or eliminate an iNP underlies different and often more complex mechanisms than particle agglomeration or disintegration in aqueous solution.
Experimental results on cellular uptake are expected to be dose- and time-dependent. They are not only closely related to the number of nanoparticles taken up, but can vary depending on the capacity of cells to degrade or eliminate them after uptake. Thus, intracellular persistence correlates to intracellular accumulation and exposure. It is therefore expected that adverse effects gain in severity with increasing levels of uptake and persistence, in particular if iNP are administered repeatedly.
3.2.3. Dosimetry and dose
It is important to note that correct dosimetry is paramount for any type of nanosafety evaluation and ENP risk assessment (Teeguarden et al. Citation2007). Cellular uptake, intracellular persistence, and toxicity are in most cases dose-dependent. For in vitro studies on nanoparticles, appropriate doses range from ng/ml to µg/ml (Siegrist et al. Citation2017). To translate the dose-range from in vitro and in vivo experiments to humans (human equivalent dose, HED), no-observed-adverse-effect-level (NOAEL) values from corresponding animal experiments are used. The animal-derived NOAEL is multiplied with a conversion factor (km), which is different for each species. It is based on the body surface area and is set by the FDA. After calculating the HED, a maximum recommended starting dose (MRSD) is evaluated. Dividing HED values by a safety or uncertainty factor (often a factor of 10) is the common procedure to calculate an MRSD and reduce the risk of possible adverse effects in humans. The MRSD is given in milligrams per kilogram body weight (mg/kg), which can be reproducibly calculated for each individual (FDA Citation2005; Nair and Jacob Citation2016). highlights the maximum recommended dose and route of administration of selected nanomedicine formulations approved by the FDA. The given doses correspond to the highest recommended dose for a person weighing 60 kg with a body surface area of 1.69 m2. For reasons of completeness, nanocrystals – which are administered at very high doses – are also listed in . However, these oral dosage forms cannot be designated as iNP based on criteria of the HES as discussed in previous sections. Nevertheless, therapeutic doses of injectable nanomedicines typically cover three orders of magnitude depending on the indication, as the selection of approved nanomedicines in shows.
Table 1. Applied doses of FDA approved nanomedicines. Dose, dosing interval, administration route(s), and supplement size of selected FDA approved nanomedicines.
In accordance with regulatory guidelines on pharmaceutical applications, we therefore recommend that a no-observed-adverse-effect-level (NOAEL) as well as a maximum recommended starting dose (MRSD) specifically for iNP should be established routinely. Nano-specific HED and km values should be calculated on a case-by-case basis considering the unique toxicological profile of nanoparticles and their intended clinical application. This should be done for the final formulation, which will be administered to the patient. If the preclinical formulations substantially differ from the final product formulations, the previously measured values should be updated. We recommend that for injectable nanomedicines, the dose units should uniformly be given in mg/kg (rather than U/kg or mg/m2) as proposed in 2005 by the FDA for initial clinical trials (FDA Citation2005).
3.3. Tier 3: Hazard assessment
As described in , the ENP interaction system of iNP is subdivided into four categories that depend on uptake and persistence.
Category I – low cellular uptake and low intracellular persistence
Category II – high cellular uptake and low intracellular persistence
Category III – low cellular uptake and high intracellular persistence
Category IV – high cellular uptake and high intracellular persistence
The assignment of an iNP to one of the four HES risk categories () dictates which set of assays must be performed to assess the safety of the formulation in question. As the four categories are related to possible adverse outcomes, the assay cascades become more elaborate with increasing uptake and persistence of the iNP. However, this does not necessarily suggest increasing levels of nanoparticle toxicity: For example, an iNP in category II may show greater toxic potential than a counterpart entering category IV. We propose eight different parameters in total, which should be monitored throughout hazard assessment. These parameters cover complement activation, platelet aggregation, hemolysis, oxidative stress, cell viability, phagocytosis, inflammation, and DNA damage. An overview of assays recommended by the USNCL or the EUNCL is given in . This includes measurement technique, assay type, specific category affiliation, and a protocol reference. It should be noted that the incorporation of different toxicogenomics techniques such as genomics, proteomics, and metabolomics may complement or eventually replace a number of biochemical or cell based assays mentioned in since there is a growing interest in these approaches in the nanosafety community (Fröhlich Citation2017). In general, the HES approach is highly dependent on the selection of specific assays and endpoints that best represent the critical hazard criteria that are relevant to the in vivo situation. Therefore, the approach should provide some flexibility in revisiting and updating the specific subsets of assays as the science evolves.
Table 2. Hazard assessment portfolio. This table shows the nine possible parameters that can be determined in the HES Tier 3.
The most common routes of administration summarized under the term injection are intravenous, subcutaneous, and intramuscular. Complement activation, platelet aggregation, and hemolysis are risks associated with intravenously injected iNP. Such adverse effects are less likely if iNP are not in direct contact with the lymphoid system and bloodstream (Turner et al. Citation2011). Nevertheless, we believe it is important to test for these events routinely for any type of iNP-injection based on the observations that platelet aggregation and complement activation can be observed after s.c. (Ermidou-Pollet et al. Citation2005) and i.m (Atkinson, Taylor, and Chetty Citation1985) injections of nanomaterials (Meng et al. Citation2011). Moreover, hemolysis has been reported to occur after i.m. diclofenac-therapy as well (Ahrens et al. Citation2004). provides a summary of the required safety assessments for each category of iNP.
Figure 5. Recommended HES hazard assessments for iNP. Depending on the outcomes in tier 2, an iNP is proposed to pass a hazard assessment cascade individually tailored for one of the four risk categories. Increasing levels of uptake and persistence require more elaborate assay cascades. The eight assays include complement activation, platelet aggregation, hemolysis, oxidative stress, cell viability, phagocytosis, inflammation, and DNA damage.

3.3.1. Application of the hazard assessment concept
In the following sections, the case of super-paramagnetic iron oxide nanoparticles (SPIONs) is discussed to demonstrate the applicability and validity of the HES recommendations provided in . These iNPs are used as imaging agents and therapeutics to treat glioblastoma, iron deficiency anemia, and iron deficiency in chronic kidney disease (Kievit and Zhang Citation2011; McCormack Citation2012; Ros et al. Citation1995; Weinstein et al. Citation2010). We were able to match one specific type of SPION to every ENP interaction group and to discuss the proposed HES test strategy in the light of observed in vitro and in vivo toxic effects. SPIONs were categorized based on FDA compliant preclinical test procedures. Temporal changes in the surface coating of the ENP are not considered. This approach can be justified since storage stability under physiological conditions was assessed based on criteria discussed above.
Category I:
Pegylated SPIONs proposed as theranostics do not seem to be acutely toxic to rats after injection. In animal experiments, viability did not decrease over the course of three weeks (Jarockyte et al. Citation2016). Moreover, neither significant endocytic uptake nor accumulation of the particles was detectable leading to an extended systemic circulation and a prolonged contact with blood cells. These particles are considered to be well tolerated. However, PEGylation may potentially cause complement activation (leading to the hypersensitivity syndrome ‘C activation-related pseudo allergy – CARPA’) and represents a possible risk (Verhoef and Anchordoquy Citation2013). Based on these considerations, a test strategy with a focus on complement activation, platelet aggregation, and hemolysis is recommended. Moreover, even though no notable viability decrease was observed, there is a regulatory need of assuring that ENP do not affect cell viability. It is, therefore, required to test cell viability as well.
Category II:
Unlike their PEGylated counterparts, endocytic cellular uptake is commonly observed with uncoated SPIONs (Singh et al. Citation2010). These nanoparticles are rapidly degraded within the lysosomal compartment placing them in the risk category II. In vitro, their degradation has been shown to trigger cytotoxic events such as mitochondrial oxidative stress and a significant cell viability decrease. In vivo, phagocytosis and hypersensitivity have been reported to be the consequence of treatment of rats with this type of SPIONs (Mahmoudi et al. Citation2012; Park Citation2003; Szebeni et al. Citation2015).
Category III:
Approved drug formulations, such as Rienso (Friedrich et al. Citation2015) or Feraheme (Lu et al. Citation2010) are SPIONs coated with a layer of polyglucose sorbitol carboxymethylether (PSC). They are used to treat iron deficiency in patients suffering from chronic kidney disease. The PSC layer contributes to low cellular uptake and high persistence, simultaneously minimizing cytotoxic effects (low platelet aggregation and phagocytosis). In patients with a history of allergies, hypersensitivity was shown to be a likely side-effect (Lu et al. Citation2010). This is an example of a nanoparticle that would have successfully passed the proposed HES hazard assessment in category III.
Category IV:
In situ-coated lauric acid ferrofluid (SEONLA) (Friedrich et al. Citation2015; Zaloga et al. Citation2014) was used to validate the effect of different lauric acid coatings on SPIONs towards the application as a drug delivery system in vitro as well as in vivo. The formulation displayed high levels of cellular uptake (Zaloga et al. Citation2014) paired with a high biopersistence (Cicha Citation2016) in vitro. However, this formulation proved to be cytotoxic. Cell viability decreased and generation of reactive oxygen species combined with DNA damage were observed (Friedrich et al. Citation2015; Zaloga et al. Citation2014). Moreover, the tendency of SEONLA to agglomerate while in circulation is indicative of a liability for phagocytosis, complement activation, platelet aggregation and hemolysis. The proposed HES test strategy for category IV ENP would have been appropriate to uncover these risks.
4. HES compared to existing hazard evaluation strategies
The HES provides an application-oriented and effective hazard evaluation cascade based on a novel classification approach and well-accepted protocols. To our knowledge, the HES is the first hazard evaluation strategy that targets solely the pharmaceutical applicability of injectable ENP and does this in a unique manner.
To underline this statement, we compared our strategy to three alternative grouping and hazard evaluation strategies that were proposed either by regulatory authorities or by academia. The chosen strategies use different approaches and levels of complexity to determine nanomaterial safety.
4.1. QSAR-based grouping and modeling
The two-tiered strategy groups metal oxide nanoparticles based on their physicochemical characteristics and subsequently uses a quantitative structure-activity relationships (QSARs) analysis to identify statistical correlations with cytotoxic effects (Sayes et al. Citation2013). Such a QSAR approach was used previously as well to group non-nano substances based on structural similarities (ECHA Citation2013). Defined physicochemical properties of nanoparticles are measured using a screening approach, normalized, and statistically analyzed. This approach relies on in silico pattern recognition techniques and predictive modeling. The workflow is schematically depicted in .
Figure 6. Workflow of a QSAR based grouping and modeling approach. Quantitative structure-activity relationships (QSAR) are used to correlate physicochemical properties of nanoparticles and cytotoxic effects. QSAR model libraries support grouping of ENP.

This test strategy was validated based on the analysis of silver, copper, nickel, iron, and zinc nanoparticles. Nanoparticles were suspended in aqueous media according to a defined protocol. The measured properties included engineered particle size, agglomerate size in water, ζ-potential, pH, and age of suspension. The measured biological response was the production of ROS. In order to compare results, the raw data was normalized ‘[…] by dividing each raw data value by the standard deviation in its respective data type […]’ (Sayes et al. Citation2013). Statistical methods (i.e. principal component analysis – PCA and correlation analysis – CA) were used to group the metal oxide nanoparticles (Tämm et al. Citation2016). In contrast to the HES, this QSAR approach was specifically designed to support the analysis of metal nanoparticles. Considering that a solid validation of the HES will provide a large amount of processable data, data mining and QSAR analysis could aid to establish a future grouping strategy for iNP. For metal oxide nanoparticles this has already been shown by Zhang et al. (Citation2012) where the ENP band gap was used to develop predictive paradigm for oxidative stress and inflammation (Zhang et al. Citation2012).
4.2. Dosimetry-based risk assessment
In this approach, nanoparticles can be grouped as safe or unsafe in relation to the applied nanoparticle dose, which in this case is uniformly defined in square meters per kilogram (m2/kg) (Simkó, Nosske, and Kreyling Citation2014). Weighting factors are introduced to account for differences in physicochemical properties of ENP. The concept provides an elegant solution on how to calculate the dose rate (DR), committed tissue dose (TD), equivalent dose (ED), and effective dose (DEff) of a nanoparticle formulation based on the knowledge of the original deposited nanoparticle dose (D) ().
Table 3. Nanoparticle risk assessment based on dose exposure. Starting from a deposited dose, the dose rate or dose equivalents of nanoparticles deposited in specific target tissues or organs are estimated.
The reactivity and thus toxic potential of different nanoparticles at a given dose can be determined by calculating the ED considering several nanoparticle-specific properties (i.e. nanoparticle weighting factors, WN). The WN chosen by the authors were specific surface area, surface texture, ζ-potential, particle morphology, band gap energy levels for metal as well as metal oxide nanoparticles, and particle dissolution/dissociation rates. This concept supports our notion that a careful dose assessment is indispensable to compare and interpret experiments and studies. Although the HES does provide its own proposal on dosimetry as well, the two concepts differ significantly. In principle, the dosimetry concept is designed to enable risk assessments for any nanomaterial in any tissue or organ and groups nanomaterials thereafter. However, at present, the concept has limitations due to the lack of tissue- and organ-specific data and was applied to the analysis of metal particles only.The HES assumes that toxicity does not occur at a defined exposure but is based on dose-response relationships. The HES does not define exposure limits for toxicity but rather uncovers potential hazards.
4.3. The ‘DF4Nano’ concept
In 2015, the European Centre for Ecotoxicology and Toxicology of Chemicals ‘Nano Task Force’ (ECETOC) has published a new decision-making framework on nanomaterial grouping (Arts et al. 2015). The DF4NanoGrouping approach is a multi-tiered grouping strategy that ‘[…] uses the ‘functionality’ of nanomaterials for grouping rather than relying on intrinsic material properties alone […].’ With the strict exception of medical or therapeutic ENP, nanomaterials can be grouped with the DF4Nano approach according to the adverse outcome pathway (AOP) concept (Ankley et al. Citation2010). An AOP is designed to establish a link between a sequence of measurable key events and an adverse outcome. The DF4Nano is the first AOP concept specific to nanomaterials. The strategy is divided into four main parts as shown in . Starting with defining and measuring intrinsic material properties, the concept takes system-dependent nanomaterial properties into account before determining their impact on cells and entire systems (organs, organisms). The experimental section is divided into three tiers of in vitro assay cascades. In tier 1, basic physicochemical properties for ENM are determined. This includes the determination of particle size, surface area, shape, aspect ratio, chemical composition, aqueous solubility, and impurities. Thereafter, system-dependent properties such as exposure, uptake, biodistribution, biopersistence, and cellular effects are monitored in tier 2. In vivo studies are carried out in tier 3. Based on this concept, four main nanomaterial groups are defined (i.e. soluble, biopersistent, passive and active nanomaterials). Biopersistence refers to insoluble high-aspect-ratio (HARN) nanomaterials. Passive nanomaterials include non-fibrous, biopersistent materials that have the tendency to agglomerate in biological fluids. In contrast to active materials, they lack chemical reactivity. The thresholds and limitations defining the decision-making process of grouping in the DF4Nano concept are based on regulatory standards and authority proposals.
Figure 7. Overview of the DF4NanoGrouping approach of ECETOC. The DF4NanoGrouping strategy designed for nanomaterials adopts the Adverse Outcome Pathway (AOP) approach designed for chemicals. Generally, the grouping is divided into four steps where intrinsic material properties, system dependent properties, cellular effects, and apical toxic effects are determined. The experimental part of the concept is a three-tiered assay cascade. Nanomaterials are grouped into soluble, biopersistent, passive or active nanomaterials. The DF4NanoGrouping strategy is not applicable for nanomedicines or nano-therapeutics. It is explicitly designed for the risk assessment of environmental and accidental nanomaterial exposure. Adapted from Arts et al. (2015).

The DF4NanoGrouping concept is a rather complex grouping approach that is – to a certain extent – similar to the HES approach. However, the two concepts group nanomaterials based on different parameters. The DF4Nano concept is based on solubility and reactivity, whereas the HES categorizes nanoparticles according to cellular uptake and intracellular persistence. The DF4Nano concept cannot be used for the grouping and risk assessment of medical and therapeutic nanomaterials. The HES, on the other hand, does feature a possibility of establishing both for injectable nanomedicines. In contrast to the DF4Nano concept, however, the HES is not based on a systematic screening approach but combines a subset of individual experiments and specific exclusion criteria to generate standardized data needed for risk assessment.
5. Discussion
The proposed Hazard Evaluation System for Injectable Nanoparticles (HES) is a three-tiered hazard evaluation concept that was specifically designed for injectable nanoparticles (iNP) used as nanomedicines. To demonstrate that the approach poses a valid alternative or extension to existing grouping and risk assessment strategies, we compared the HES to three alternative screening and risk assessment strategies.
In , an overview of all 28 measured experimental parameters of the four discussed concepts is provided. ζ-potential, shape, size, and aspect ratio were the key properties for most concepts. Any experimental result should be critically evaluated considering the aspects of contamination, impurity, and dosimetry. This comparison shows that the HES uses the highest number of measured parameters. However, the HES (in contrast to the other strategies) is not a screening approach but uses an informed decision-making process to minimize the number of needed experiments. The HES follows thereby an intuitive and straightforward decision-making path.
Table 4. Overview of relevant hazard evaluation determinants.
In terms of future applications of the concept, several scenarios can be envisaged. First, preclinical safety evaluations will be facilitated since the HES is based on physicochemical exclusion criteria. Non-qualifying materials can be identified and the number of in vitro experiments can be minimized. Second, the number of in vitro experiments can be further reduced based on selective application of toxicological assays. This approach is cost-effective. Third, a careful in vitro evaluation of test materials at early stages of the development process helps to avoid costly in vivo experiments at later stages. This is in line with the 3R principles of animal welfare. Fourth, the HES concept will help to mitigate the risk of off-target effects in vivo. Fifth, it is tempting to speculate that a systematic evaluation of nanomaterials will form the basis for future grouping strategies. It will, thus, be possible to correlate physicochemical properties with hazard categories of injectable nanopharmaceuticals. This knowledge can guide the design of novel and/or optimized nanomaterials according to safe-by-design principles (as presented by the NanoReg and Prosafe European initiatives) to assist both regulatories and industry. It addresses issues regarding nanomaterial safety, both in products and processes (Gottardo Citation2017). The HES works towards this principle by focusing on injectable nanoparticles. By proposing a stepwise strategy based on key parameters, the HES encourages developers to consider safety during the different steps of product design.
6. Conclusions
The motivation of this project was to develop an application-oriented, cost-effective, robust, and user-friendly hazard assessment concept for nano-pharmaceuticals. To the knowledge of the authors, HES is the first hazard evaluation concept focusing entirely on the application of nanoparticles as injectable formulations. Validation of the HES approach using well characterized reference materials will be an important next step towards its acceptance and implementation by research scientists as well as regulatory authorities.
Acknowledgments
We express our gratitude to Prof. Dr. Martin Wilks (Swiss Centre for Applied Human Toxicology – SCAHT), Dr. Andrea Haase (‘‘Bundesinstitut für Risikobewertung – BfR’’ and the NanoReg2 consortium), Dr. Christoph Studer (‘‘Bundesamt für Gesundheit – BAG’’), and Dr. Blanca Suarez (TEMAS AG) for their valuable inputs on the novel hazard evaluation strategy.
Disclosure statement
Dr. P. Wick is executive board member of the EUNCL.
Additional information
Funding
References
- Aderem, A. 2003. “Phagocytosis and the Inflammatory Response.” The Journal of Infectious Diseases 187 (s2): S340–S345. doi:10.1086/374747
- Aguilar-Castillo, B. A., J. L. Santos, H. Luo, Y. E. Aguirre-Chagala, T. Palacios-Hernandez, and M. Herrera-Alonso. 2015. “Nanoparticle Stability in Biologically Relevant Media: influence of Polymer Architecture.” Soft Matter 11 (37): 7296–7307. doi:10.1039/C5SM01455G
- Ahrens, N., L. Schewior, E. Garbe, H. Kiesewetter, and A. Salama. 2004. “Massive Haemolysis after Intramuscular Diclofenac in a Patient Who Apparently Tolerated Oral Medication.” Vox Sanguinis 86 (1): 71–74. doi:10.1111/j.0042-9007.2004.00389.x
- Akthakul, A., A. I. Hochbaum, F. Stellacci, and A. M. Mayes. 2005. “Size Fractionation of Metal Nanoparticles by Membrane Filtration.” Advanced Materials 17 (5): 532–535. doi:10.1002/adma.200400636
- Álvarez-Fuentes, J., L. Martín-Banderas, I. Muñoz-Rubio, M. A. Holgado, and M. Fernández-Arévalo. 2012. “Development and Validation of an RP-HPLC Method for CB13 Evaluation in Several PLGA Nanoparticle Systems [WWW Document].” The Scientific World Journal 2012: 737526. doi:10.1100/2012/737526
- Ambrogio, M. W., C. R. Thomas, Y.-L. Zhao, J. I. Zink, and J. F. Stoddart. 2011. “Mechanized Silica Nanoparticles: A New Frontier in Theranostic Nanomedicine.” Accounts of Chemical Research 44 (10): 903–913. doi:10.1021/ar200018x
- Amenabar, I., S. Poly, W. Nuansing, E. H. Hubrich, A. A. Govyadinov, F. Huth, R. Krutokhvostov, L. Zhang, M. Knez, J. Heberle. J., et al. 2013. “Structural Analysis and Mapping of Individual Protein Complexes by Infrared Nanospectroscopy.” Nature Communications 4 (1): 3890. Ncomms doi:10.1038/ncomms3890
- Amidon, G. L., H. Lennernäs, V. P. Shah, and J. R. Crison. 1995. “A Theoretical Basis for a Biopharmaceutic Drug Classification: The Correlation of in Vitro Drug Product Dissolution and in Vivo Bioavailability.” Pharmaceutical Research 12: 413–420. doi:10.1023/A:1016212804288
- Ankley, G. T., R. S. Bennett, R. J. Erickson, D. J. Hoff, M. W. Hornung, R. D. Johnson, D. R. Mount, J. W. Nichols, C. L. Russom, P. K. Schmieder, et al. 2010. “Adverse Outcome Pathways: A Conceptual Framework to Support Ecotoxicology Research and Risk Assessment.” Environmental Toxicology and Chemistry 29 (3): 730–741. doi:10.1002/etc.34
- Arap, W., R. Pasqualini, M. Montalti, L. Petrizza, L. Prodi, E. Rampazzo, N. Zaccheroni, and S. Marchiò. 2013. “Luminescent Silica Nanoparticles for Cancer Diagnosis.” Current Medicinal Chemistry 20 (17): 2195–2211. doi:10.2174/0929867311320170005
- Arts, J. H. E., M. Hadi, M.-A. Irfan, A. M. Keene, R. Kreiling, D. Lyon, M. Maier, K. Michel, T. Petry, U. G. Sauer, et al. 2015a. “A Decision-Making Framework for the Grouping and Testing of Nanomaterials (DF4nanoGrouping).” Regulatory Toxicology and Pharmacology 71 (2): S1–S27. doi:10.1016/j.yrtph.2015.03.007
- Atkinson, P. M., D. I. Taylor, and N. Chetty. 1985. “Inhibition of Platelet Aggregation by Ketamine Hydrochloride.” Thrombosis Research 40 (2): 227–234. doi:10.1016/0049-3848(85)90333-0
- Bao, H., X. Yu, C. Xu, X. Li, Z. Li, D. Wei, and Y. Liu. 2015. “New Toxicity Mechanism of Silver Nanoparticles: Promoting Apoptosis and Inhibiting Proliferation.” PLoS One 10 (3): e0122535. doi:10.1371/journal.pone.0122535
- Bartneck, M., H. A. Keul, S. Singh, K. Czaja, J. Bornemann, M. Bockstaller, M. Moeller, G. Zwadlo-Klarwasser, and J. Groll. 2010a. “Rapid Uptake of Gold Nanorods by Primary Human Blood Phagocytes and Immunomodulatory Effects of Surface Chemistry.” ACS Nano 4 (6): 3073–3086. doi:10.1021/nn100262h
- Bartneck, M., H. A. Keul, G. Zwadlo-Klarwasser, and J. Groll. 2010b. “Phagocytosis Independent Extracellular Nanoparticle Clearance by Human Immune Cells.” Nano Letters 10 (1): 59–63. doi:10.1021/nl902830x
- Berg, J. M., A. Romoser, N. Banerjee, R. Zebda, and C. M. Sayes. 2009. “The Relationship between pH and Zeta Potential of ∼30 Nm Metal Oxide Nanoparticle Suspensions Relevant to in Vitro Toxicological Evaluations.” Nanotoxicology 3 (4): 276–283. doi:10.3109/17435390903276941
- Berger, M. 2009. “Size matters. Comparing the toxicity of micro- to nanoparticles” [WWW Document]. Accessed August 21 2017. http://www.nanowerk.com/spotlight/spotid=10128.php
- Bhattacharjee, S. 2016. “DLS and Zeta Potential – What They Are and What They Are Not?.” Journal of Controlled Release 235: 337–351. doi:10.1016/j.jconrel.2016.06.017
- Bleeker, E. A. J., W. H. de Jong, R. E. Geertsma, M. Groenewold, E. H. W. Heugens, M. Koers-Jacquemijns, D. van de Meent, J. R. Popma, A. G. Rietveld, S. W. P. Wijnhoven, et al. 2013. “Considerations on the EU Definition of a Nanomaterial: Science to Support Policy Making.” Regulatory Toxicology and Pharmacology 65 (1): 119–125. doi:10.1016/j.yrtph.2012.11.007
- Bobo, D., K. J. Robinson, J. Islam, K. J. Thurecht, and S. R. Corrie. 2016. “Nanoparticle-Based Medicines: A Review of FDA-Approved Materials and Clinical Trials to Date.” Pharmaceutical Research 33 (10): 2373–2387. doi:10.1007/s11095-016-1958-5
- Boilard, S. P., P. R. Amyotte, F. I. Khan, A. G. Dastidar, and R. K. Eckhoff. 2013. “Explosibility of Micron- and Nano-Size Titanium Powders.” Journal of Loss Prevention in the Process Industries 26 (6): 1646–1654. doi:10.1016/j.jlp.2013.06.003
- Bolt, H. M. 2014. “Grouping of Nanomaterials for Risk Assessment.” Archives of Toxicology 88 (12): 2077–2078. doi:10.1007/s00204-014-1416-2
- Bozzuto, G., and A. Molinari. 2015. “Liposomes as Nanomedical Devices.” International Journal of Nanomedicine 10: 975–999. doi10.2147/IJN.S68861
- Brown, J. S., T. Gordon, O. Price, and B. Asgharian. 2013. “Thoracic and Respirable Particle Definitions for Human Health Risk Assessment.” Particle and Fibre Toxicology 10 (1): 12. doi:10.1186/1743-8977-10-12
- Buzea, C., I. I. Pacheco, and K. Robbie. 2007. “Nanomaterials and Nanoparticles: sources and Toxicity.” Biointerphases 2 (4): MR17–MR71. doi:10.1116/1.2815690
- Casals, E., T. Pfaller, A. Duschl, G. J. Oostingh, and V. Puntes. 2010. “Time Evolution of the Nanoparticle Protein Corona.” ACS Nano 4 (7): 3623–3632. doi:10.1021/nn901372t
- Champion, J. A., Y. K. Katare, and S. Mitragotri. 2007. “Particle Shape: A New Design Parameter for Micro- and Nanoscale Drug Delivery Carriers.” Official journal of the Controlled Release Society 121 (1–2): 3–9. doi:10.1016/j.jconrel.2007.03.022
- Chen, F., G. Wang, J. I. Griffin, B. Brenneman, N. K. Banda, V. M. Holers, D. S. Backos, L. Wu, S. M. Moghimi, and D. Simberg. 2017. “Complement Proteins Bind to Nanoparticle Protein Corona and Undergo Dynamic Exchange In Vivo.” Nature Nanotechnology 12 (4): 387–393. doi:10.1038/nnano.2016.269
- Chukwuocha, E. O., M. C. Onyeaju, and T. S. T. Harry. 2012. “Theoretical Studies on the Effect of Confinement on Quantum Dots Using the Brus Equation World Journal of Condensed Matter Physics 2 (2): 96–100. doi:10.4236/wjcmp.2012.22017
- Cicha, I. 2016. “Strategies to Enhance Nanoparticle-Endothelial Interactions under Flow.” Journal of Cellular Biotechnology 1 (2): 191–208. doi:10.3233/JCB-15020
- Clogston, J. D., and A. K. Patri. 2011. “Zeta Potential Measurement.” Methods in Molecular Biology (Clifton, N.J.) 697: 63–70. doi:10.1007/978-1-60327-198-1
- Coti, K. K., M. E. Belowich, M. Liong, M. W. Ambrogio, Y. A. Lau, H. A. Khatib, J. I. Zink, N. M. Khashab, and J. F. Stoddart. 2009. “Mechanised Nanoparticles for Drug Delivery.” Nanoscale 1 (1): 16–39. doi:10.1039/b9nr00162j
- Crawford, R., B. Dogdas, E. Keough, R. M. Haas, W. Wepukhulu, S. Krotzer, P. A. Burke, L. Sepp-Lorenzino, A. Bagchi, and B. J. Howell. 2011. “Analysis of Lipid Nanoparticles by Cryo-EM for Characterizing siRNA Delivery Vehicles.” International Journal of Pharmaceutics 403 (1–2): 237–44. doi:10.1016/j.ijpharm.2010.10.025
- Davignon, J., and P. Ganz. 2004. “Role of Endothelial Dysfunction in Atherosclerosis.” Circulation 109 (23_suppl_1): III-27–III-32. doi:10.1161/01.CIR.0000131515.03336.f8
- Deanfield, J., A. Donald, C. Ferri, C. Giannattasio, J. Halcox, S. Halligan, A. Lerman, G. Mancia, J. J. Oliver, A. C. Pessina, et al., Working Group on Endothelin and Endothelial Factors of the European Society of Hypertension. 2005. “Endothelial Function and Dysfunction. Part I: Methodological Issues for Assessment in the Different Vascular Beds: A Statement by the Working Group on Endothelin and Endothelial Factors of the European Society of Hypertension.” Journal of Hypertension 23 (1): 7–17. doi:10.1097/00004872-200501000-00004
- Dekkers, S., A. G. Oomen, E. A. J. Bleeker, R. J. Vandebriel, C. Micheletti, J. Cabellos, G. Janer, N. Fuentes, S. Vázquez-Campos, T. Borges, et al. 2016. “Towards a Nanospecific Approach for Risk Assessment.” Regulatory Toxicology and Pharmacology 80: 46–59. doi:10.1016/j.yrtph.2016.05.037
- Dobrovolskaia, M. A., A. K. Patri, J. Simak, J. B. Hall, J. Semberova, S. H. De Paoli Lacerda, and S. E. McNeil. 2012. “Nanoparticle Size and Surface Charge Determine Effects of PAMAM Dendrimers on Human Platelets in Vitro.” Molecular Pharmaceutics 9 (3): 382–393. doi:10.1021/mp200463e
- Docter, D., D. Westmeier, M. Markiewicz, S. Stolte, S. K. Knauer, and R. H. Stauber. 2015. “The Nanoparticle Biomolecule Corona: lessons Learned – Challenge Accepted?” Chemical Society Reviews 44: 6094–6121. doi:10.1039/C5CS00217F
- Dongargaonkar, A. A., and J. D. Clogston. 2018. “Quantitation of Surface Coating on Nanoparticles Using Thermogravimetric Analysis.” In Characterization of Nanoparticles Intended for Drug Delivery, Methods in Molecular Biology, edited by McNeil S, 57–63. New York, NY: Humana Press. doi:10.1007/978-1-4939-7352-1_6
- Droppo, I. G. 2006. “Filtration in Particle Size Analysis.” In Encyclopedia of Analytical Chemistry, edited by R. A. Meyers and R. B. Flippen. Hoboken, New Jersey: John Wiley & Sons, Ltd. doi:10.1002/9780470027318.a1506
- Dusinska, M., M. Dusinska, L. Fjellsbø, Z. Magdolenova, A. Rinna, E. Runden Pran, A. Bartonova, E. Heimstad, M. Harju, L. Tran. et al. 2009. “Testing Strategies for the Safety of Nanoparticles Used in Medical Applications.” Nanomed 4 (6): 605–607. doi:10.2217/nnm.09.47
- ECHA. 2013. “Grouping of Substances and Read-Across – ECHA” [WWW Document]. Accessed August 28 2017. https://echa.europa.eu/support/registration/how-to-avoid-unnecessary-testing-on-animals/grouping-of-substances-and-read-across
- Elgqvist, J. 2017. “Nanoparticles as Theranostic Vehicles in Experimental and Clinical Applications—Focus on Prostate and Breast Cancer.” International Journal of Molecular Sciences 18 (5): 1102. doi:10.3390/ijms18051102
- Ermidou-Pollet, S., H. Nounopoulos, N. Sdougas, M. Szilágyi, and S. Pollet. 2005. “Influence of Subcutaneous Injection of Essential Fatty Acids on the Stress-Induced Modifications of Rat Platelet Aggregation and Membrane Lipid Composition.” Acta Biologica Hungarica 56 (3–4): 247–259. doi:10.1556/ABiol.56.2005.3-4.8
- Etheridge, M. L., S. A. Campbell, A. G. Erdman, C. L. Haynes, S. M. Wolf, and J. McCullough. 2013. “The Big Picture on Small Medicine: The State of Nanomedicine Products Approved for Use or in Clinical Trials.” Nanomedicine: Nanotechnology, Biology, and Medicine 9 (1): 1–14. doi:10.1016/j.nano.2012.05.013
- European Medicines Agency - Multidisciplinary - Data requirements for intravenous iron-based nano-colloidal products developed with reference to an innovator medicinal product [WWW Document], n.d. Accessed January 19 2018. http://www.ema.europa.eu/docs/en_GB/document_library/Scientific_guideline/2015/03/WC500184922.pdf
- European Medicines Agency - Multidisciplinary - Joint MHLW/EMA reflection paper on the development of block copolymer micelle medicinal products [WWW Document], n.d. Accessed January 19 2018. http://www.ema.europa.eu/docs/en_GB/document_library/Scientific_guideline/2013/02/WC500138390.pdf
- European Medicines Agency - Multidisciplinary - Reflection paper on surface coatings: general issues for consideration regarding parenteral administration of coated nanomedicine products [WWW Document], n.d. Accessed January 19 2018. http://www.ema.europa.eu/docs/en_GB/document_library/Scientific_guideline/2013/08/WC500147874.pdf
- European Medicines Agency - Multidisciplinary - Reflection paper on the data requirements for intravenous liposomal products developed with reference to an innovator liposomal product [WWW Document], n.d. Accessed January 19 2018. http://www.ema.europa.eu/docs/en_GB/document_library/Scientific_guideline/2013/03/WC500140351.pdf
- European Medicines Agency - Multidisciplinary - Reflection paper on nanotechnology-based medicinal products for human use [WWW Document], n.d. Accessed 19 Jan 2018. http://www.ema.europa.eu/docs/en_GB/document_library/Regulatory_and_procedural_guideline/2010/01/WC500069728.pdf
- Fabricius, A.-L., L. Duester, B. Meermann, and T. A. Ternes. 2014. “ICP-MS-Based Characterization of Inorganic Nanoparticles—Sample Preparation and off-Line Fractionation Strategies.” Analytical and Bioanalytical Chemistry 406 (2): 467–479. doi:10.1007/s00216-013-7480-2
- Fadeel, B. 2012. Clear and Present Danger? Engineered Nanoparticles and the Immune System [WWW document]. Swiss Med. Wkly. Accessed May 11 2016. http://blog.smw.ch/
- Fadeel, B., A. Pietroiusti, and A. A. Shvedova. 2017. Adverse Effects of Engineered Nanomaterials: Exposure, Toxicology, and Impact on Human Health. Cambridge, Massachusetts: Academic Press.
- FDA. 2005. “Guidance for Industry on Estimating the Maximum Safe Starting Dose in Initial Clinical Trials for Therapeutics in Adult Healthy Volunteers.” Availability [WWW Document]. Fed. Regist. Accessed August 24 2017. https://www.federalregister.gov/documents/2005/07/22/05-14456/guidance-for-industry-on-estimating-the-maximum-safe-starting-dose-in-initial-clinical-trials-for
- Félétou, M. 2011. “The Endothelium: Part 1: Multiple Functions of the Endothelial Cells—Focus on Endothelium-Derived Vasoactive Mediators.” In Integrated Systems Physiology: From Molecule to Function to Disease, edited by D. Neil Granger and Joey P. Granger, 1–306. San Rafael (CA): Morgan & Claypool Life Sciences.
- Feliu, N., and B. Fadeel. 2010. “Nanotoxicology: No Small Matter.” Nanoscale 2 (12): 2514–2520. doi:10.1039/c0nr00535e
- Friedrich, R. P., C. Janko, M. Poettler, P. Tripal, J. Zaloga, I. Cicha, S. Dürr, J. Nowak, S. Odenbach, I. Slabu. et al. 2015. “Flow Cytometry for Intracellular SPION Quantification: Specificity and Sensitivity in Comparison with Spectroscopic Methods.” International Journal of Nanomedicine 10: 4185–4201. doi:10.2147/IJN.S82714
- Fröhlich, E. 2017. “Role of Omics Techniques in the Toxicity Testing of Nanoparticles.” Journal of Nanobiotechnology 15 (1): 84. doi:10.1186/s12951-017-0320-3
- Fubini, B., M. Ghiazza, and I. Fenoglio. 2010. “Physico-Chemical Features of Engineered Nanoparticles Relevant to Their Toxicity.” Nanotoxicology 4 (4): 347–363. doi:10.3109/17435390.2010.509519
- Gallud, A., and B. Fadeel. 2015. “Keeping It Small: Towards a Molecular Definition of Nanotoxicology.” European Journal of Nanomedicine 7: 143–151. doi:10.1515/ejnm-2015-0020
- Garbovskiy, Y. 2017. “Biological Contamination of Nanoparticles and Its Manifestation in Optical Absorbance Measurements.” Analytical Chemistry 89 (14): 7282–7285. doi:10.1021/acs.analchem.7b01766
- Gebel, T., H. Foth, G. Damm, A. Freyberger, P.-J. Kramer, W. Lilienblum, C. Röhl, T. Schupp, C. Weiss, K.-M. Wollin, et al. 2014. “Manufactured Nanomaterials: Categorization and Approaches to Hazard Assessment.” Archives of Toxicology 88 (12): 2191–2211. doi:10.1007/s00204-014-1383-7
- Goldstein, A., Y. Soroka, M. Frušić-Zlotkin, I. Popov, and R. Kohen. 2014. “High Resolution SEM Imaging of Gold Nanoparticles in Cells and Tissues.” Journal of Microscopy 256 (3): 237–247. doi:10.1111/jmi.12179
- Gottardo, S. 2017. “NANoREG Framework for the Safety Assessment of Nanomaterials - EU Science Hub - European Commission [WWW Document].” EU Science Hub. Accessed March 7 2018. https://ec.europa.eu/jrc/en/publication/eur-scientific-and-technical-research-reports/nanoreg-framework-safety-assessment-nanomaterials
- Gregoriadis, G., 1985. Liposomes for drugs and vaccines. Trends Biotechnol. 3: 235–241. doi:10.1016/0167-7799(85)90014-9
- Gregory, A. E., R. Titball, and D. Williamson. 2013. “Vaccine Delivery Using Nanoparticles.” Frontiers in Cellular and Infection Microbiology 3: 13. doi:10.3389/fcimb.2013.00013
- Guadagnini, R., B. Halamoda Kenzaoui, L. Walker, G. Pojana, Z. Magdolenova, D. Bilanicova, M. Saunders, L. Juillerat-Jeanneret, A. Marcomini, A. Huk, et al. 2015. “Toxicity Screenings of Nanomaterials: Challenges Due to Interference with Assay Processes and Components of Classic in Vitro Tests.” Nanotoxicology 9 (Supp1): 13–24. doi:10.3109/17435390.2013.829590
- Guarnieri, D., S. Sabella, O. Muscetti, V. Belli, M. A. Malvindi, S. Fusco, E. De Luca, P. P. Pompa, and P. A. Netti. 2014. “Transport across the Cell-Membrane Dictates Nanoparticle Fate and Toxicity: A New Paradigm in Nanotoxicology.” Nanoscale 6 (17): 10264–10273. doi:10.1039/C4NR02008A
- Guha, S., M. Li, M. J. Tarlov, and M. R. Zachariah. 2012. “Electrospray–Differential Mobility Analysis of Bionanoparticles.” Trends Biotechnol 30 (5): 291–300. doi:10.1016/j.tibtech.2012.02.003
- Guo, C., Y. Xia, P. Niu, L. Jiang, J. Duan, Y. Yu, X. Zhou, Y. Li, and Z. Sun. 2015. “Silica Nanoparticles Induce Oxidative Stress, Inflammation, and Endothelial Dysfunction in Vitro via Activation of the MAPK/Nrf2 Pathway and Nuclear Factor-κB Signaling.” International Journal of Nanomedicine 10: 1463–1477. doi:10.2147/IJN.S76114
- Guo, C., and J. L. Yarger. 2018. “Characterizing Gold Nanoparticles by NMR Spectroscopy.” Magnetic Resonance in Chemistry. doi:10.1002/mrc.4753. [Epub ahead of print]
- Hackley, V. A., and J. D. Clogston. 2011. “Measuring the Hydrodynamic Size of Nanoparticles in Aqueous Media Using Batch-Mode Dynamic Light Scattering.” In Characterization of Nanoparticles Intended for Drug Delivery, edited by S. E. McNeil, 35–52. Totowa, NJ: Humana Press. doi:10.1007/978-1-60327-198-1_4
- Hadrup, N., A. K. Sharma, M. Poulsen, and E. Nielsen. 2015. “Toxicological Risk Assessment of Elemental Gold following Oral Exposure to Sheets and Nanoparticles - A Review.” Regulatory Toxicology and Pharmacology 72 (2): 216–221. doi:10.1016/j.yrtph.2015.04.017
- Hole, P., K. Sillence, C. Hannell, C. M. Maguire, M. Roesslein, G. Suarez, S. Capracotta, Z. Magdolenova, L. Horev-Azaria, A. Dybowska, et al. 2013. “Interlaboratory Comparison of Size Measurements on Nanoparticles Using Nanoparticle Tracking Analysis (NTA).” Journal of Nanoparticle Research 15 (12): 2101. doi:10.1007/s11051-013-2101-8
- Hung, H.-I., O. J. Klein, S. W. Peterson, S. R. Rokosh, S. Osseiran, N. H. Nowell, and C. L. Evans. 2016. “PLGA Nanoparticle Encapsulation Reduces Toxicity While Retaining the Therapeutic Efficacy of EtNBS-PDT In Vitro.” Scientific Reports 6 (1): 33234. doi:10.1038/srep33234
- Huth, F., A. Govyadinov, S. Amarie, W. Nuansing, F. Keilmann, and R. Hillenbrand. 2012. “Nano-FTIR Absorption Spectroscopy of Molecular Fingerprints at 20 Nm Spatial Resolution.” Nano Letters 12 (8): 3973–3978. doi:10.1021/nl301159v
- ISO. 2015. “ISO/TS 80004-2:2015(en), Nanotechnologies — Vocabulary — Part 2: Nano-objects.” [WWW Document]. Accessed August 28 2017. https://www.iso.org/obp/ui/#iso:std:iso:ts:80004:-2:ed-1:v1:en
- Itoh, N., E. Yamamoto, T. Santa, T. Funatsu, and M. Kato. 2016. “Effect of Nanoparticle Surface on the HPLC Elution Profile of Liposomal Nanoparticles.” Pharmaceutical Research 33 (6): 1440–1446. doi:10.1007/s11095-016-1886-4
- Jarockyte, G., E. Daugelaite, M. Stasys, U. Statkute, V. Poderys, T.-C. Tseng, S.-H. Hsu, V. Karabanovas, and R. Rotomskis. 2016. “Accumulation and Toxicity of Superparamagnetic Iron Oxide Nanoparticles in Cells and Experimental Animals.” International Journal of Molecular Sciences 17 (8): 1193. doi:10.3390/ijms17081193
- Jong, W. 2010. “Scientific Basis for the Definition of the Term ‘nanomaterial’.” [WWW Document] Accessed 28 August 2017. http://ec.europa.eu/health/scientific_committees/emerging/docs/scenihr_o_032.pdf
- Joris, F., D. Valdepérez, B. Pelaz, S. J. Soenen, B. B. Manshian, W. J. Parak, S. C. De Smedt, and K. Raemdonck. 2016. “The Impact of Species and Cell Type on the Nanosafety Profile of Iron Oxide Nanoparticles in Neural Cells.” Journal of Nanobiotechnology 14 (1): 69. doi:10.1186/s12951-016-0220-y
- Junghanns, J.-U. A. H., and R. H. Müller. 2008. “Nanocrystal Technology, Drug Delivery and Clinical Applications.” International Journal of Nanomedicine 3 (3): 295–310.
- Kaszuba, M., D. McKnight, M. T. Connah, F. K. McNeil-Watson, and U. Nobbmann. 2008. “Measuring Sub Nanometre Sizes Using Dynamic Light Scattering.” Journal of Nanoparticle Research 10 (5): 823–829. doi:10.1007/s11051-007-9317-4
- Ke, P. C., S. Lin, W. J. Parak, T. P. Davis, and F. Caruso. 2017. “A Decade of the Protein Corona.” ACS Nano 11 (12): 11773–11776. doi:10.1021/acsnano.7b08008
- Kennedy, I. M., D. Wilson, and A. I. Barakat; HEI Health Review Committee. 2009. “Uptake and Inflammatory Effects of Nanoparticles in a Human Vascular Endothelial Cell Line.” Research reports - Health Effects Institute 136: 3–32.
- Kettiger, H., A. Schipanski, P. Wick, and J. Huwyler. 2013. “Engineered Nanomaterial Uptake and Tissue Distribution: From Cell to Organism.” International Journal of Nanomedicine 8: 3255–3269. doi:10.2147/IJN.S49770
- Kievit, F. M., and M. Zhang. 2011. “Surface Engineering of Iron Oxide Nanoparticles for Targeted Cancer Therapy.” Accounts of Chemical Research 44 (10): 853–862. doi:10.1021/ar2000277
- Kim, I.-Y., E. Joachim, H. Choi, and K. Kim. 2015. “Toxicity of Silica Nanoparticles Depends on Size, Dose, and Cell Type.” Nanomedicine: Nanotechnology, Biology, and Medicine 11 (6): 1407–1416. doi:10.1016/j.nano.2015.03.004
- Krug, H. F. 2014. “Nanosafety Research – Are We On the Right Track?” Angewandte Chemie (International Ed. In English) 53 (46): 12304–12319. doi:10.1002/anie.201403367
- Krug, H. F., and P. Wick. 2011. “Nanotoxicology: An Interdisciplinary Challenge.” Angewandte Chemie International Edition 50 (6): 1260–1278. doi:10.1002/anie.201001037
- Laux, P., C. Riebeling, A. M. Booth, J. D. Brain, J. Brunner, C. Cerrillo, O. Creutzenberg, I. Estrela-Lopis, T. Gebel, G. Johanson, et al. 2017. “Biokinetics of Nanomaterials: The Role of Biopersistence.” NanoImpact 6: 69–80. doi:10.1016/j.impact.2017.03.003
- Lee, J. H., Y. S. Kim, K. S. Song, H. R. Ryu, J. H. Sung, J. D. Park, H. M. Park, N. W. Song, B. S. Shin, D. Marshak, et al. 2013. “Biopersistence of Silver Nanoparticles in Tissues from Sprague–Dawley Rats.” Particle and Fibre Toxicology 10 (1): 36. doi:10.1186/1743-8977-10-36
- Li, L. K. Y. Mak, J. Shi, C. H. Leung, C. M. Wong, C. W. Leung, C. S. K. Mak, K. Y. Chan, N. M. M. Chan, E. X. Wu, and P. W. T. Pong. 2013. “Sterilization on Dextran-Coated Iron Oxide Nanoparticles: Effects of Autoclaving, Filtration, UV Irradiation, and Ethanol Treatment.” Microelectronic Engineering 111: 310–313. doi:10.1016/j.mee.2013.02.021
- Li, T., A. J. Senesi, and B. Lee. 2016. “Small Angle X-Ray Scattering for Nanoparticle Research.” Chemical Reviews 116 (18): 11128–11180. doi:10.1021/acs.chemrev.5b00690
- Li, Y., and D. Boraschi. 2016. “Endotoxin Contamination: A Key Element in the Interpretation of Nanosafety Studies.” Nanomed 11 (3): 269–287. doi:10.2217/nnm.15.196
- Li, Z., Y. Zhang, D. Zhu, S. Li, X. Yu, Y. Zhao, X. Ouyang, Z. Xie, and L. Li. 2017. “Transporting Carriers for Intracellular Targeting Delivery via Non-Endocytic Uptake Pathways.” Drug Delivery 24 (2): 45–55. doi:10.1080/10717544.2017.1391889
- Lovestam, G. 2010. “Considerations on a Definition of Nanomaterial for Regulatory Purposes.” [WWW Document]. Accessed 28 August 2017. https://ec.europa.eu/jrc/sites/jrcsh/files/jrc_reference_report_201
- Lu, M., M. H. Cohen, D. Rieves, and R. Pazdur. 2010. “FDA Report: Ferumoxytol for Intravenous Iron Therapy in Adult Patients with Chronic Kidney Disease.” American Journal of Hematology 85 (5): 315–319. doi:10.1002/ajh.21656
- Maguire, C. M., Sillence, K. Roesslein, M. Hannell, C. Suarez, G. Sauvain, J.-J. Capracotta, S. Contal, S. Cambier, S. El Yamani. N. et al., 2017. “Benchmark of Nanoparticle Tracking Analysis on Measuring Nanoparticle Sizing and Concentration.” Journal of Micro and Nano-Manufacturing 5 (4): 041002–041010. doi:10.1115/1.4037124
- Mahmoudi, M., H. Hofmann, B. Rothen-Rutishauser, and A. Petri-Fink. 2012. “Assessing the in Vitro and in Vivo Toxicity of Superparamagnetic Iron Oxide Nanoparticles.” Chemical Reviews 112 (4): 2323–2338. doi:10.1021/cr2002596
- Mansfield, E., K. M. Tyner, C. M. Poling, and J. L. Blacklock. 2014. “Determination of Nanoparticle Surface Coatings and Nanoparticle Purity Using Microscale Thermogravimetric Analysis.” Analytical Chemistry 86: 1478–1484. doi:10.1021/ac402888v
- McCormack, P. L. 2012. “Ferumoxytol: In Iron Deficiency Anaemia in Adults with Chronic Kidney Disease.” Drugs 72: 2013–2022. doi:10.2165/11209880-000000000-00000
- Mehn, D., F. Caputo, M. Rösslein, L. Calzolai, F. Saint-Antonin, T. Courant, P. Wick, and D. Gilliland. 2017. “Larger or More? Nanoparticle Characterisation Methods for Recognition of Dimers.” RSC Advances 7 (44): 27747–27754. doi:10.1039/C7RA02432K
- Meng, J., M. Yang, F. Jia, Z. Xu, H. Kong, and H. Xu. 2011. “Immune Responses of BALB/c Mice to Subcutaneously Injected Multi-Walled Carbon Nanotubes.” Nanotoxicology 5 (4): 583–591. doi:10.3109/17435390.2010.523483
- Moghimi, S. M., and D. Simberg. 2017. “Complement Activation Turnover on Surfaces of Nanoparticles.” Nano Today 15: 8–10. doi:10.1016/j.nantod.2017.03.001
- Moore, T. L., L. Rodriguez-Lorenzo, V. Hirsch, S. Balog, D. Urban, C. Jud, B. Rothen-Rutishauser, M. Lattuada, and A. Petri-Fink. 2015. “Nanoparticle Colloidal Stability in Cell Culture Media and Impact on Cellular Interactions.” Chemical Society Reviews 44 (17): 6287–6305. doi:10.1039/C4CS00487F
- Mühlebach, S., G. Borchard, and S. Yildiz. 2015. “Regulatory Challenges and Approaches to Characterize Nanomedicines and Their Follow-on Similars.” Nanomed 10 (4): 659–674. doi:10.2217/nnm.14.189
- Nair, A. B., and S. Jacob. 2016. “A Simple Practice Guide for Dose Conversion between Animals and Human.” Journal of Basic and Clinical Pharmacy 7 (2): 27–31. doi:10.4103/0976-0105.177703
- Navya, P. N., and H. K. Daima. 2016. “Rational Engineering of Physicochemical Properties of Nanomaterials for Biomedical Applications with Nanotoxicological Perspectives.” Nano Converg 3 (1): 1. doi:10.1186/s40580-016-0064-z
- Neagu, M., Z. Piperigkou, K. Karamanou, A. B. Engin, A. O. Docea, C. Constantin, C. Negrei, D. Nikitovic, and A. Tsatsakis. 2017. “Protein Bio-Corona: critical Issue in Immune Nanotoxicology.” Archives of Toxicology 91 (3): 1031–1048. doi:10.1007/s00204-016-1797-5
- Nel, A., T. Xia, L. Mädler, and N. Li. 2006. “Toxic Potential of Materials at the Nanolevel.” Science 311 (5761): 622–627. doi:10.1126/science.1114397
- Nemmar, A., P. Yuvaraju, S. Beegam, J. Yasin, E. E. Kazzam, and B. H. Ali. 2016. “Oxidative Stress, Inflammation, and DNA Damage in Multiple Organs of Mice Acutely Exposed to Amorphous Silica Nanoparticles.” International Journal of Nanomedicine 11: 919–928. doi:10.2147/IJN.S92278
- NSCT/CoT/NSET. 2014. “2014 NNI Strategic Plan | Nano.” [WWW Document]. Accessed August 23 2017. https://www.nano.gov/node/1113
- Oberdörster, G. 2010. “Safety Assessment for Nanotechnology and Nanomedicine: concepts of Nanotoxicology.” Journal of Internal Medicine 267 (1): 89–105. doi:10.1111/j.1365-2796.2009.02187.x
- Oberdörster, G., V. Stone, and K. Donaldson. 2007. “Toxicology of Nanoparticles: A Historical Perspective.” Nanotoxicology 1 (1): 2–25. doi:10.1080/17435390701314761
- Oh, N., and J.-H. Park. 2014. “Endocytosis and Exocytosis of Nanoparticles in Mammalian Cells.” International Journal of Nanomedicine 9: 51–63. doi:10.2147/IJN.S26592
- Oomen, A. G., E. A. J. Bleeker, P. M. J. Bos, F. van Broekhuizen, S. Gottardo, M. Groenewold, D. Hristozov, K. Hund-Rinke, M.-A. Irfan, A. Marcomini, et al. 2015. “Grouping and Read-across Approaches for Risk Assessment of Nanomaterials.” International Journal of Environmental Research and Public Health 12 (10): 13415–13434. doi:10.3390/ijerph121013415
- Panchal, J., J. Kotarek, E. Marszal, and E. M. Topp. 2014. “Analyzing Subvisible Particles in Protein Drug Products: A Comparison of Dynamic Light Scattering (DLS) and Resonant Mass Measurement (RMM).” The AAPS Journal 16 (3): 440–451. doi:10.1208/s12248-014-9579-6
- Park, J.-B. 2003. “Phagocytosis Induces Superoxide Formation and Apoptosis in Macrophages.” Experimental & Molecular Medicine 35 (5): 325–335. doi:10.1038/emm.2003.44
- Park, M. V. D. Z., A. M. Neigh, J. P. Vermeulen, L. J. J. de la Fonteyne, H. W. Verharen, J. J. Briedé, H. van Loveren, and W. H. de Jong. 2011. “The Effect of Particle Size on the Cytotoxicity, Inflammation, Developmental Toxicity and Genotoxicity of Silver Nanoparticles.” Biomaterials 32 (36): 9810–9817. doi:10.1016/j.biomaterials.2011.08.085
- Pelaz, B., C. Alexiou, R. A. Alvarez-Puebla, F. Alves, A. M. Andrews, S. Ashraf, L. P. Balogh, L. Ballerini, A. Bestetti, C. Brendel, et al. 2017. “Diverse Applications of Nanomedicine.” ACS Nano 11 (3): 2313–2381. doi:10.1021/acsnano.6b06040
- Potočnik, J. 2011. “Commission Recommendation of 18 October 2011 on the Definition Of Nanomaterial 2011/696/EU.” Accessed 28 August 2017. https://eur-lex.europa.eu/legal-content/EN/ALL/?uri=CELEX:32011H0696
- Puri, A., K. Loomis, B. Smith, J.-H. Lee, A. Yavlovich, E. Heldman, and R. Blumenthal. 2009. “Lipid-Based Nanoparticles as Pharmaceutical Drug Carriers: From Concepts to Clinic.” Critical Reviews in Therapeutic Drug Carrier Systems 26 (6): 523–580. doi:10.1615/CritRevTherDrugCarrierSyst.v26.i6.10
- Pyrz, W. D., and D. J. Buttrey. 2008. “Particle Size Determination Using TEM: A Discussion of Image Acquisition and Analysis for the Novice Microscopist.” Langmuir 24 (20): 11350–11360. doi:10.1021/la801367j
- Rao, A., M. Schoenenberger, E. Gnecco, T. Glatzel, E. Meyer, D. Brändlin, and L. Scandella. 2007. “Characterization of Nanoparticles Using Atomic Force Microscopy.” Journal of Physics: Conference Series 61: 971. doi:10.1088/1742-6596/61/1/192
- Roberson, S., and G. J. Weltje. 2014. “Inter-Instrument Comparison of Particle-Size Analysers.” Sedimentology 61 (4): 1157–1174. doi:10.1111/sed.12093
- Robertson, J. D., L. Rizzello, M. Avila-Olias, J. Gaitzsch, C. Contini, M. S. Magoń, S. A. Renshaw, and G. Battaglia. 2016. “Purification of Nanoparticles by Size and Shape.” Scientific Reports 6 (1): 27494. doi:10.1038/srep27494
- Ros, P. R., P. C. Freeny, S. E. Harms, S. E. Seltzer, P. L. Davis, T. W. Chan, A. E. Stillman, L. R. Muroff, V. M. Runge, and M. A. Nissenbaum. 1995. “Hepatic MR Imaging with Ferumoxides: A Multicenter Clinical Trial of the Safety and Efficacy in the Detection of Focal Hepatic Lesions.” Radiology 196 (2): 481–488. doi:10.1148/radiology.196.2.7617864
- Sabella, S. R. P. Carney, V. Brunetti, M. A. Malvindi, N. Al-Juffali, G. Vecchio, S. M. Janes, O. M. Bakr, R. Cingolani, F. Stellacci, and P. P. Pompa. 2014. “A General Mechanism for Intracellular Toxicity of Metal-Containing Nanoparticles.” [†Electronic Supplementary Information (ESI) available. See doi:10.1039/c4nr01234h Click here for additional data file.] Nanoscale 6: 7052–7061.
- Saraiva, C., C. Praça, R. Ferreira, T. Santos, L. Ferreira, and L. Bernardino. 2016. “Nanoparticle-Mediated Brain Drug Delivery: Overcoming Blood–Brain Barrier to Treat Neurodegenerative Diseases.” Journal of Controlled Release 235: 34–47. doi:10.1016/j.jconrel.2016.05.044
- Sayes, C. M., N. Banerjee, and A. A. Romoser. 2009. “The Role of Oxidative Stress in Nanotoxicology.”In General, Applied and Systems Toxicology, edited by B. Ballantyne, T. C. Marrs, T. Syversen, D. A. Casciano and S. C. Sahu. Hoboken, New Jersey: John Wiley & Sons, Ltd. doi:10.1002/9780470744307.gat245
- Sayes, C. M., P. A. Smith, and I. V. Ivanov. 2013. “A Framework for Grouping Nanoparticles Based on Their Measurable Characteristics.” International Journal of Nanomedicine 8: 45–56. doi:10.2147/IJN.S40521
- Scott-Fordsmand, J. J., S. Pozzi-Mucelli, L. Tran, K. Aschberger, S. Sabella, U. Vogel, C. Poland, D. Balharry, T. Fernandes, S. Gottardo, et al. 2014. “A Unified Framework for Nanosafety Is Needed.” Nano Today 9 (5): 546–549. doi:10.1016/j.nantod.2014.07.001
- Seaton, A., L. Tran, R. Aitken, and K. Donaldson. 2010. “Nanoparticles, Human Health Hazard and Regulation.” Journal of the Royal Society Interface 7 (Suppl_1): S119–S129. doi:10.1098/rsif.2009.0252.focus
- Serpone, N., and S. Horikoshi. 2013. “Microwaves in Nanoparticle Synthesis: Fundamentals and Applications.” [WWW Document]. Accessed August 28 2017. http://www.wiley.com/WileyCDA/WileyTitle/productCd-3527331972.html
- Shannahan, J. 2017. “The Biocorona: A Challenge for the Biomedical Application of Nanoparticles.” Nanotechnology Reviews 6: 345–353. doi:10.1515/ntrev-2016-0098
- Shimizu, K., H. Nakamura, and S. Watano. 2016. “MD Simulation Study of Direct Permeation of a Nanoparticle across the Cell Membrane under an External Electric Field.” Nanoscale 8 (23): 11897–11906. doi:10.1039/C6NR02051H
- Shvedova, A. A., V. E. Kagan, and B. Fadeel. 2010. “Close Encounters of the Small Kind: adverse Effects of Man-Made Materials Interfacing with the Nano-Cosmos of Biological Systems.” Annual Review of Pharmacology and Toxicology 50 (1): 63–88. doi:10.1146/annurev.pharmtox.010909.105819
- Siegrist, S., H. Kettiger, E. Fasler-Kan, and J. Huwyler. 2017. “Selective Stimulation of the JAK/STAT Signaling Pathway by Silica Nanoparticles in Human Endothelial Cells.” Toxicology In Vitro 42: 308–318. doi:10.1016/j.tiv.2017.05.002
- Simkó, M., D. Nosske, and W. G. Kreyling. 2014. “Metrics, Dose, and Dose Concept: The Need for a Proper Dose Concept in the Risk Assessment of Nanoparticles.” International Journal of Environmental Research and Public Health 11 (4): 4026–4048. doi:10.3390/ijerph110404026
- Simkó, M., S. Tischler, and M.-O. Mattsson. 2015. “Pooling and Analysis of Published in Vitro Data: A Proof of Concept Study for the Grouping of Nanoparticles.” International Journal of Molecular Sciences 16 (11): 26211–26236. doi:10.3390/ijms161125954
- Singh, A. K. 2015. Engineered Nanoparticles: Structure, Properties and Mechanisms of Toxicity. Cambridge, Massachusetts: Academic Press.
- Singh, N., G. J. Jenkins, R. Asadi, and S. H. Doak. 2010. “Potential Toxicity of Superparamagnetic Iron Oxide Nanoparticles (SPION).” Nano Rev 1 (1): 5358. doi:10.3402/nano.v1i0.5358
- Stone, V., S. Pozzi-Mucelli, L. Tran, K. Aschberger, S. Sabella, U. Vogel, C. Poland, D. Balharry, T. Fernandes, S. Gottardo, et al. 2014. “ITS-NANO - Prioritising Nanosafety Research to Develop a Stakeholder Driven Intelligent Testing Strategy.” Particle and Fibre Toxicology 11 (1): 9. doi:10.1186/1743-8977-11-9
- Szebeni, J., S. Fishbane, M. Hedenus, S. Howaldt, F. Locatelli, S. Patni, D. Rampton, G. Weiss, and J. Folkersen. 2015. “Hypersensitivity to Intravenous Iron: classification, Terminology, Mechanisms and Management.” British Journal of Pharmacology 172 (21): 5025–5036. doi:10.1111/bph.13268
- Tämm, K., L. Sikk, J. Burk, R. Rallo, S. Pokhrel, L. Mädler, J. J. Scott-Fordsmand, P. Burk, and T. Tamm. 2016. “Parametrization of Nanoparticles: development of Full-Particle Nanodescriptors.” Nanoscale 8 (36): 16243–16250. doi:10.1039/C6NR04376C
- Teeguarden, J. G., P. M. Hinderliter, G. Orr, B. D. Thrall, and J. G. Pounds. 2007. “Particokinetics in Vitro: dosimetry Considerations for in Vitro Nanoparticle Toxicity Assessments.” Toxicological Sciences 95 (2): 300–312. doi:10.1093/toxsci/kfl165
- Tiwari, D., J. Takashi, and J. Behari. 2010. “Dose-Dependent In-Vivo Toxicity Assessment of Nanoparticle in Wistar Rats.” Toxicology Mechanisms and Methods 21 (1): 13–24. doi:10.3109/15376516.2010.529184
- Toy, R., L. Bauer, C. Hoimes, K. B. Ghaghada, and E. Karathanasis. 2014. “Targeted Nanotechnology for Cancer Imaging.” Advanced Drug Delivery Reviews 76: 79–97. doi:10.1016/j.addr.2014.08.002
- Treuel, L., D. Docter, M. Maskos, and R. H. Stauber. 2015. “Protein Corona – from Molecular Adsorption to Physiological Complexity.” Beilstein Journal of Nanotechnology 6: 857–873. doi:10.3762/bjnano.6.88
- Turner, P. V., T. Brabb, C. Pekow, and M. A. Vasbinder. 2011. “Administration of Substances to Laboratory Animals: Routes of Administration and Factors to Consider.” Journal of the American Association for Laboratory Animal Science 50 (5): 600–613.
- Unfried, K., C. Albrecht, L.-O. Klotz, A. Von Mikecz, S Grether-Beck, R.P.F. Schins. 2007. “Cellular responses to nanoparticles: Target structures and mechanisms.” Nanotoxicology 1: 52–71. doi:10.1080/00222930701314932
- Unterweger, H., C. Janko, M. Schwarz, L. Dézsi, R. Urbanics, J. Matuszak, E. Őrfi, T. Fülöp, T. Bäuerle, J. Szebeni. et al. 2017. “Non-Immunogenic Dextran-Coated Superparamagnetic Iron Oxide Nanoparticles: A Biocompatible, Size-Tunable Contrast Agent for Magnetic Resonance Imaging.” International Journal of Nanomedicine 12: 5223–5238. doi:10.2147/IJN.S138108
- Uskoković, V. 2013. “Entering the Era of Nanoscience: Time to Be so Small.” Journal of Biomedical Nanotechnology 9 (9): 1441–1470. doi:10.1166/jbn.2013.1642
- van Teunenbroek, T. 2017. “NANoREG, A Common European Approach to the Regulatory Testing of Nanomaterials.” [WWW Document]. www.nanoreg.eu. Accessed December 21 2017. http://www.rivm.nl/en/About_RIVM/Mission_and_strategy/International_Affairs/International_Projects/Completed/NANoREG/NANoREG_Results_Repository_sub_page_Final_Report
- Verhoef, J. J., and T. J. Anchordoquy. 2013. “Questioning the Use of PEGylation for Drug Delivery. Drug Deliv.” Drug Delivery and Translational Research 3 (6): 499–503. doi:10.1007/s13346-013-0176-5
- Vermeij, E. A., M. I. Koenders, M. B. Bennink, L. A. Crowe, L. Maurizi, J.-P. Vallée, H. Hofmann, W. B. Berg, L. van den, P. L. E. M. van, Loo, and F. A. J. van de. 2015. “The in-Vivo Use of Superparamagnetic Iron Oxide Nanoparticles to Detect Inflammation Elicits a Cytokine Response but Does Not Aggravate Experimental Arthritis.” PLoS One 10 (5): e0126687. doi:10.1371/journal.pone.0126687
- Vetten, M. A., C. S. Yah, T. Singh, and M. Gulumian. 2014. “Challenges Facing Sterilization and Depyrogenation of Nanoparticles: effects on Structural Stability and Biomedical Applications.” Nanomedicine: Nanotechnology, Biology, and Medicine 10 (7): 1391–1399. doi:10.1016/j.nano.2014.03.017
- Vippola, M., M. Valkonen, E. Sarlin, M. Honkanen, and H. Huttunen. 2016. “Insight to Nanoparticle Size Analysis—Novel and Convenient Image Analysis Method versus Conventional Techniques.” Nanoscale Research Letters 11 (1): 169. doi:10.1186/s11671-016-1391-z
- Weinstein, J. S., C. G. Varallyay, E. Dosa, S. Gahramanov, B. Hamilton, W. D. Rooney, L. L. Muldoon, and E. A. Neuwelt. 2010. “Superparamagnetic Iron Oxide Nanoparticles: diagnostic Magnetic Resonance Imaging and Potential Therapeutic Applications in Neurooncology and Central Nervous System Inflammatory Pathologies, A Review.” Journal of Cerebral Blood Flow & Metabolism 30 (1): 15–35. doi:10.1038/jcbfm.2009.192
- Weissig, V., T. K. Pettinger, and N. Murdock. 2014. “Nanopharmaceuticals (Part 1): Products on the Market.” International Journal of Nanomedicine 9: 4357–4373. doi:10.2147/IJN.S46900
- Weissleder, R., M. Nahrendorf, and M. J. Pittet. 2014. “Imaging Macrophages with Nanoparticles.” Nature Materials 13 (2): 125–138. doi:10.1038/nmat3780
- Wen, H., M. Dan, Y. Yang, J. Lyu, A. Shao, X. Cheng, L. Chen, and L. Xu. 2017. “Acute Toxicity and Genotoxicity of Silver Nanoparticle in Rats.” PLoS One 12 (9): e0185554. doi:10.1371/journal.pone.0185554
- Whitesides, G. M. 2003. “The “Right” Size in Nanobiotechnology.” Nature Biotechnology 21 (10): 1161–1165. doi:10.1038/nbt872
- Witzigmann, D., M. Camblin, J. Huwyler, and V. Balasubramanian. 2015. “Nanomaterials: Therapeutic Applications.”In The Encyclopedia of Biomedical Polymers & Polymeric Biomaterials, edited by M. Mishra, 5364–5378. New York: Taylor & Francis.
- Wolfram, J., M. Zhu, Y. Yang, J. Shen, E. Gentile, D. Paolino, M. Fresta, G. Nie, C. Chen, H. Shen. et al. 2015. “Safety of Nanoparticles in Medicine.” Current Drug Targets 16 (14): 1671–1681. doi:10.2174/1389450115666140804124808
- Wu, X., Z.-R. Lu. 2016. “Nanoparticle–Tissue Interaction..” In Nanomaterials in Pharmacology. Methods in Pharmacology and Toxicology, edited by Z.-R. Lu, S. Sakuma, 201–218. New York, NY: Humana Press. doi:10.1007/978-1-4939-3121-7
- Xie, J., S. Lee, and X. Chen. 2010. “Nanoparticle-Based Theranostic Agents.” Advanced Drug Delivery Reviews 62 (11): 1064–1079. doi:10.1016/j.addr.2010.07.009
- Yameen, B., W. I. Choi, C. Vilos, A. Swami, J. Shi, and O. C. Farokhzad. 2014. “Insight into Nanoparticle Cellular Uptake and Intracellular Targeting.” 30th Anniversary Special Issue 190: 485–499. doi:10.1016/j.jconrel.2014.06.038
- Yang, Y., Z. Qin, W. Zeng, T. Yang, Y. Cao, C. Mei, and Y. Kuang. 2017. “Toxicity Assessment of Nanoparticles in Various Systems and Organs.” Nanotechnology Reviews 6: 279. doi:10.1515/ntrev-2016-0047
- Yildirimer, L., N. T. K. Thanh, M. Loizidou, and A. M. Seifalian. 2011. “Toxicology and Clinical Potential of Nanoparticles.” Nano Today 6 (6): 585–607. doi:10.1016/j.nantod.2011.10.001
- Yuan, S. Y., and R. R. Rigor. 2010. The Endothelial Barrier. San Rafael, California: Morgan & Claypool Life Sciences.
- Zaloga, J., C. Janko, J. Nowak, J. Matuszak, S. Knaup, D. Eberbeck, R. Tietze, H. Unterweger, R. P. Friedrich, S Duerr, et al. 2014. “Development of a Lauric Acid/Albumin Hybrid Iron Oxide Nanoparticle System with Improved Biocompatibility.” International Journal of Nanomedicine 9: 4847–4866. doi:10.2147/IJN.S68539
- Zhang, H., Z. Ji, T. Xia, H. Meng, C. Low-Kam, R. Liu, S. Pokhrel, S. Lin, X. Wang, Y.-P. Liao, et al. 2012. “Use of Metal Oxide Nanoparticle Band Gap to Develop a Predictive Paradigm for Oxidative Stress and Acute Pulmonary Inflammation.” ACS Nano 6 (5): 4349–4368. doi:10.1021/nn3010087
- Zhang, S., J. Li, G. Lykotrafitis, G. Bao, and S. Suresh. 2009. “Size-Dependent Endocytosis of Nanoparticles.” Advanced Materials 21 (4): 419–424. doi:10.1002/adma.200801393
- Zhao, L., A. Seth, N. Wibowo, C.-X. Zhao, N. Mitter, C. Yu, and A. P. J. Middelberg. 2014. “Nanoparticle Vaccines.” Vaccine 32 (3): 327–337. doi:10.1016/j.vaccine.2013.11.069
- Zheng, J., J. D. Clogston, A. K. Patri, M. A. Dobrovolskaia, and S. E. McNeil. 2011. “Sterilization of Silver Nanoparticles Using Standard Gamma Irradiation Procedure Affects Particle Integrity and Biocompatibility.” Journal of Nanomedicine & Nanotechnology S5 (01): 001. doi:10.4172/2157-7439.S5-001