Abstract
Nanotechnology is considered to be a key enabling technology and in recent years there has been much growth in the use of nanostructured materials in industrial applications and in consumer products. It is, therefore, important that prior to being commercialized in consumer products, engineered nanomaterials are subjected to a thorough physico-chemical characterization as part of broader risk assessment to evaluate their possible effects on human health and the environment. The proper dispersion of nanomaterials sourced as powders becomes a first crucial step in the characterization process. This paper focuses on the dispersion of multiwall carbon nanotubes - often hydrophobic and tangled - since it may be challenging to re-disperse them effectively in aqueous media prior to characterization. A comparison has been made of non-contact vial sonication and immersion probe sonication using tannic acid as a dispersant. Transmission electron microscopy and UV-Vis spectroscopy were the techniques used to evaluate the dispersions. We used High Content Imaging and Colony Forming Efficiency to perform in vitro cytotoxicity studies on Human Alveolar Epithelial cells. It was found that both sonication treatments produce equivalent stable dispersions. No cytotoxic effects from MWCNTs were observed although some toxicity was observed and attributed to excess of the tannic acid dispersant.
Introduction
Manufacturing of engineered nanomaterials is rapidly growing, and with it the presence in the market place of consumer products containing nanomaterials. In particular, during the last ten years the commercialization of products containing carbon nanotubes (CNTs) has significantly increased as manufacturers begin to exploit the exceptional mechanical, electrical and thermal properties of CNTs (De Volder et al. Citation2013). Currently, CNTs are found in a wide range of industrial applications, such as energy storage devices, enhanced coatings, high-strength and conductive composites, as well as components of medical devices and biosensors; although such materials have been the subject of much intensive study their special properties and relative novelty means there is still a great need to adequately assess possible health and environmental effects and to manage any potential risks of these new forms of materials.
An important starting point in such an assessment is to undertake a detailed physico-chemical characterization of the engineered nanomaterial and for this many instrumental methods require the material to be dispersed in a suitable liquid (Hurt et al. Citation2006). If the material is not supplied as a stable dispersion, then the dry material needs to be dispersed into a liquid, possibly with the addition of a surfactant and, in most cases, the input of energy by high intensity sonication (Hartmann et al. Citation2015; Taurozzi et al. Citation2011). This step is of great importance since the majority of nanomaterial interactions with living systems will occur in liquid biological media where the nature and degree of dispersion and aggregation may vary the physical properties of the nanomaterials (Handy et al. Citation2008; Taurozzi et al. Citation2012; Vietti et al. Citation2016) as well as influencing the way they interact with, e.g. cells, proteins, and membranes. Understanding this behaviour and the possible changes that nanomaterials may undergo in biological media will help to better design experimental protocols not only for characterizing different nanomaterials but also for reliable sample preparation prior to in vitro testing of their biological activity.
CNTs tend to exist as tightly bound bundles and are a good example of a nanomaterial whose dispersion into individual fibers is not trivial due to their strong tendency to aggregate in many common solvents and aqueous media in particular. In the as-synthesised state, CNTs are generally hydrophobic with strong Van der Waals forces along the axis of the tubes which effectively hinder their dispersion in hydrophilic media complicating their use in many practical applications (Hyung et al. Citation2007; Ikeda et al. Citation2006; Krause et al. Citation2009). Different approaches for the dispersion of CNTs can be found in literature (Fujigaya and Nakashima Citation2015; Nilsson et al. Citation2015; Vaisman et al. Citation2006) such as (i) oxidation of the CNTs surface to increase their dispersibility in water at the expense of modifying some CNTs properties, (ii) covalent functionalization conferring high stability to the CNTs although it may also cause damage which alters their original properties, (iii) noncovalent interactions with polymers or surfactants which better preserve the intrinsic features of the CNTs. This last option is usually performed via sonication treatment in a solution of (a) functionalized polymers resulting in stable polymer wrapping or (b) a low molecular weight surfactant which may be easily washed off causing the formation of CNTs bundles again. Among the most common surfactants used, it is possible to find sodium cholate (SC) (Ishibashi and Nakashima Citation2006), sodium dodecyl sulfate (SDS) (Datsyuk et al. Citation2009; Krause et al. Citation2009; Moore et al. Citation2003; Rastogi et al. Citation2008; Richard et al. Citation2003; Yu et al. Citation2007), sodium dodecylbenzene sulfonate (SDBS) (Klumpp et al., Citation2006), Triton X (Moore et al. Citation2003; Rastogi et al. Citation2008), ammonium laurate (Nilsson et al. Citation2015), Tween (Datsyuk et al. Citation2009; Moore et al. Citation2003; Rastogi et al. Citation2008), Brij (Moore et al. Citation2003), pluronic (Moore et al. Citation2003; Vaisman et al. Citation2006) and cethyltrimethylammonium bromide (CTAB) (Moore et al. Citation2003) many of which can be toxic to living cells. Dimethyl sulfoxide (DMSO) is an example of a solvent widely used to increase the solubility of poorly soluble nonpolar and polar compounds, CNTs among them (Buford et al. Citation2007; Ponti et al. Citation2013). DMSO has recently been reported to induce cellular toxicity at concentrations lower than 5% v/v (Galvao et al. Citation2014; Yuan et al. Citation2014). This highlights the importance of choosing a dispersant which will avoid generating false positive results in toxicity tests.
CNT length is an important parameter which has a remarkable impact on their properties. For example, Shen et al. Citation2017 have recently discussed how carbon nanotube length affects the permeability and the viscoelasticity of Bucky papers. With respect to their bioreactivity (Ema et al. Citation2017; Luanpitpong et al. Citation2014; Mercer et al. Citation2011; Poland et al. Citation2008; Poulsen et al. Citation2016; Sweeney et al. Citation2014; Vietti et al. Citation2016), CNTs have a fibrous asbestos-like structure and their high aspect ratio creates concern about their potential harmful effects when inhaled. The length of carbon nanotube fibers is a major factor affecting pulmonary toxicity resulting in different cell responses which, depending on the length of the carbon nanotubes (Chatterjee et al. Citation2016; Cheng and Cheng Citation2012), may cause pro-inflammatory responses and potentially a longer term pulmonary pathology. Therefore, morphological characterization of CNTs is essential although it remains a challenge because they are difficult to obtain in the form of separate fibers and suitable dispersions are needed. In practice, characterizing CNTs with their fibrous appearance is usually only possible by microscopic techniques such as Atomic force microscopy (AFM), scanning tunneling microscopy (STM) and transmission electron microscopy (TEM) which are the most commonly used (Belin and Epron Citation2005; Gao et al. Citation2015; Gommes et al. Citation2003; Krause et al. Citation2011).
The aim of this study is to present a protocol based on two sonication methods, able to produce CNT suspensions stable for a long time. Both methods resulted suitable to obtain stable suspensions at higher or lower stock concentrations. Advantages and disadvantages are hereafter explained and leave the final operator free to decide the best approach to be used for the physico-chemical characterization and in vitro testing. The protocol takes into consideration the use of a solvent considered biocompatible and shows the issue of the selection of nontoxic solvent in vitro concentration and its interaction with CNTs.
This study has considered the use of tannic acid as a low toxicity surfactant which, combined with ultrasonic treatments, can be used to effectively disperse CNTs for both structural characterization and in vitro cytotoxicity studies. Tannic acid is a naturally occurring polyphenol compound which has surface-active active properties (Lin et al. Citation2009; Lin and Xing Citation2008). It is produced by many plants and trees and can be found in wood as well as some nuts, cereals, fruits, herbs, and spices (Bravo Citation1998). It is used in the food industry as a preservative and colorant. It has also been used as a model for natural organic matter dissolved in rivers which by interacting with CNTs could influence their transport and eventual fate in the environment (Hyung et al. Citation2007; Lin et al. Citation2009; Lin and Xing Citation2008). As a dispersant for CNTs, tannic acid shows the important advantage of being effective at low concentration yet non-foaming.
In this study, aqueous tannic acid solution has been combined with two different types of sonicators (vial sonicator and probe sonicator) to prepare dispersions of MWCNTs whose quality was then characterized using UV-Vis spectroscopy and transmission electron microscopy. Subsequently, the dispersions have been used for a series of in vitro cytotoxicity studies on Human Alveolar Epithelial cells (A549) performed to assess any differences in potential toxicity of MWCNTs due to the different dispersion protocols used. For this study, lung epithelial cells have been chosen as they are among the first group of cells that interact with CNTs via inhalation which is likely to be the most relevant exposure route for humans. In this context, understanding the different cell uptake and interaction mechanisms behavior of CNT’s is particularly important as they are one of the few nanostructured materials which have been reported to have a strong carcinogenic potential (Stueckle et al. Citation2016). To avoid possible interferences of MWCNTs with the test reagents or the assay, two techniques with different assay principles were employed; a non-colorimetric technique and a fluorescence-based assay. Colony forming efficiency (CFE) and high content imaging (HCI) were the methods chosen. CFE is a clonogenic cell survival assay to establish the reproduction capability of a cell, that is, the dependence between the dose of nanomaterial used and the portion of cells able to proliferate (Herzog et al. Citation2007; Ponti et al. Citation2010). HCI was chosen to perform an automatized cell count based on the imaging and analysis of DAPI-fluorescently stained nuclei.
Experimental
MWCNT dispersion preparation
The multiwall carbon nanotubes which were studied in this paper, hereafter called MWCNTs, were supplied by the JRC Nanomaterials Repository facility (new code JRC-NM 04000a; previous code NM400). For this material, the manufacturer declares an average length of 846 nm and an average diameter of 11 nm although the details of exactly how these values were obtained have not been specified by the supplier (Rasmussen Citation2014).
Dispersions of MWCNTs (0.2 mg/mL and 1 mg/mL) were prepared in aqueous solutions of tannic acid (300 mg/L) [Sigma-Aldrich, Italy]. All solutions were prepared using 18.2 MΩ cm ultrahigh purity water. These samples were sonicated as described below using the conditions noted in .
Table 1. Description of the sonication conditions used.
Probe sonicator
The MWCNTs were dispersed in solution by using an ultrasonic immersion probe sonicator (Hielscher UP200S, Germany) equipped with a titanium probe head of 7 mm diameter. The sonicator power (maximum nominal power of 200 W) was adjusted by varying amplitude and cycle time. The MWCNTs dispersions (6 mL each) were prepared at concentrations of 0.2 mg/mL and 1.0 mg/mL in tannic acid (a concentration of 300 mg/L was selected as being the most suitable for MWCNTs dispersion based on analysis done by UV-vis and TEM, data not shown) in 22 mL flat bottom glass vials. The use of a constant (6 mL) volume of suspension served to ensure that all the samples received the same specific acoustic energy. The vials were then placed in an ice bath to cool the sample during sonication and then, after immersing the probe head in the suspension to a depth of ca. 1 cm, the mixture was sonicated at 50% amplitude and 50% cycle time for 30 min (PSa and PSb ()).
The energy delivered to the suspensions by probe sonicator is reported in .
Vial sonicator (intensive vial sonicator for small volumes)
The MWCNT dispersions (1.5 mL each) were prepared in 2 mL polypropylene centrifuge tubes at a concentration of 0.2 mg/mL in tannic acid (300 mg/L). These were then ultrasonically treated in a vial sonicator (Hielscher VialTweeter model UIS250v) using the sample holder position which, according to the manufacturer's manual, provides the highest intensity input. The samples were sonicated for 60 min at 50% amplitude and 50% cycle time and then for an additional 30 min at 75% amplitude and 50% cycle time (VSc ()). In all cases a fixed sample volume (1.5 mL) served to ensure that all the samples received the same specific acoustic energy.
The energy delivered to suspension by vial sonicator is reported in .
Evaluation of dispersions
The dispersions of MWCNTs in tannic acid obtained via both types of sonicator were evaluated using UV-Vis spectroscopy and transmission electron microscopy.
UV-Vis spectroscopy
The stability of the MWCNTs dispersions in tannic acid was monitored using an Evolution 300 UV-Vis spectrophotometer (Thermo Scientific, USA) functioning between 200 and 1000 nm at room temperature. Samples were appropriately diluted to make them suitable for studies by UV-Vis spectroscopy, that is, to provide absorbance units under 0.8 and keep linearity between absorbance and concentration according to the Beer-Lambert’s law. Absorbance values were recorded at 800 nm (Lin and Xing Citation2008). UV-Vis spectra were regularly run to check the temporal stability of the dispersed samples and the extent of dispersion.
As support to the in vitro tests and to reproduce the filtration conditions carried out during such tests, additional experiments were run to check the availability of free tannic acid in solution after separation of the CNTs. The absorbance of a tannic acid solution (300 mg/L) was measured at 275 nm. Subsequently, the absorbance of the tannic acid solution was recorded after filtering it through a 0.2 µm filter and also through an Amicon 10 KDa to mimic the filtration procedure carried out in the colony forming efficiency assays. A sonicated sample of MWCNTs in tannic acid (1 mg/mL) was filtered through a 0.2 µm filter and the absorbance of the transparent filtrate was recorded at 275 nm to check the concentration of tannic acid remaining in solution after filtration of the solution previously equilibrated with the MWCNT sample.
Transmission electron microscopy
Morphology and size distribution of the MWCNTs were studied using a JEOL JEM-2100 HR-Transmission Electron Microscope (TEM) at 120 kV (JEOL, Italy).
Material stock suspensions were prepared at concentrations of 0.2 or 1.0 mg/mL of MWCNTs in tannic acid (300 mg/L) and diluted with MilliQ water or complete culture medium to the final testing concentration of 20 µg/mL.
For the preparation of TEM samples, 3 µL of suspensions were manually spotted on Cu-Formvar carbon coated (200 mesh, grids Agar Scientific, USA) grids pre-coated with Alcian blue solution 2% (w/w) (Sigma, Italy) in MilliQ water and let to dry overnight (Mast and Demeestere Citation2009).
Length and thickness of more than 3000 fibers in each sample were measured using Gatan Digital Micrograph software at (i) PSa, PSb and VSc; (ii) VSc and VSc; after 1 month of storage, (iii) VSc and VSd, and (iv) VSc and VSe (). Results were statistically analyzed by the Gaussian model fits peaks (OriginPro 2015).
Cell culture conditions
Human alveolar epithelial A549 cells were purchased mycoplasma free from ECACC (www.ecacc.org.uk). Cells were cultured in Ham’s F12 medium supplemented with 10% (v/v) Fetal Calf serum and 1% (v/v) Penicillin (10000 U/mL) Streptomycin (10000 μg/mL) (Gibco Life Technologies, Italy). Cells were grown under normal cell culture conditions (37 °C; 5% CO2 and 95% humidity) and passaged weekly in tissue culture-treated flasks (Falcon, Italy).
Colony forming efficiency assay
Colony forming efficiency (CFE) assay was performed as previously detailed elsewhere (Ponti et al. Citation2013) but modifying the culture dish area, to study the cytotoxicity induced by the MWCNTs after 48 hours of exposure. Cells were seeded at a density of 200 cells/well in 2 mL complete culture medium (12 well plate, BD Falcon™). After 24 h, culture medium was removed and cells exposed to MWCNTs in concentrations ranging from 0.31 to 200 μg/mL.
Negative control (untreated cells); solvent control (Tannic acid 300 mg/L diluted to the same concentration as the exposure suspensions) and positive control (Na2CrO4 1000 µM) for complete cell death were also included. During the course of each assay the cell medium was substituted by fresh medium, after 48 hours of exposure and after other 4 days. Finally, after a total time of 12 days from the cell seeding, the cells were fixed with 3.7% (v/v) of formaldehyde solution (Sigma-Aldrich, Milan, Italy) in phosphate buffered saline (PBS) (1X) without calcium, magnesium and sodium bicarbonate (Gibco), and stained with 10% (v/v) Giemsa solution (Sigma-Aldrich) in MilliQ water. Colonies were scored by GelCount™ (Oxford Optronix Ltd., UK). The results were expressed as CFE (%) = [(average of treatment colonies/average of negative control colonies) × 100] and the corresponding standard error mean [SEM % = SD/√(number of treatments)].
A549 cells were also exposed to the volume of tannic acid corresponding to the highest concentration tested after Amicon 10 KDa filter (Millipore, Italy) filtration.
High content imaging
For the assessment of cell viability after exposure to MWCNTs dispersed using different protocols, A549 cells were seeded in 96 well plates (Corning™ Costar™ 96-Well Black). Cells were cultured until 60% confluence was reached after which they were exposed to different concentrations of MWCNTs for 48 h. Cells were fixed and stained with DAPI using standard protocols. DAPI-stained nuclei were imaged using the IN Cell Analyzer 2200 (GE Healthcare, UK). During acquisition, a minimum of 12 fields per well were imaged, using a 10x objective. Data analysis was performed on the IN Cell Investigator Software (GE Healthcare, UK) using in-house developed protocols to count the number of nuclei in each experimental condition, with a minimum of 10 000 analyzed cells in the negative control. Data are presented as the mean ± standard error of at least three independent experiments with triplicates for each experimental condition with the corresponding standard errors being represented by error bars. The percentage of cell viability was calculated by determining the number of nuclei in the exposure conditions compared to the number of nuclei in the negative control. IC50 values were calculated by nonlinear regression with an inhibitory dose-response model using GraphPad Prism5 software. Concentrations were transformed using natural log for linear regression and regression models were adjusted for replicates and assay data.
Cell interaction
For TEM analysis, A549 cells were seeded in 75 cm2 flask (Corning Costar, Italy) at a density of 5 × 105 cells in 10 mL of complete cell culture medium. After 24 h, cells were exposed to 20 µg/mL of MWCNTs for 48 h.
After exposure, the medium was removed and cells were thoroughly washed with PBS buffer, detached using 1 mL trypsin-EDTA (Invitrogen, Italy) and centrifuged at 200 rcf for 5 min to obtain the cell pellet. The supernatant was removed and cells were fixed using a Karnovsky 2% v/v solution (glutaraldehyde and paraformaldehyde in 0.05 M cacodilate, pH 7.3, Sigma Aldrich, Italy) overnight.
Cells were then washed 3 times with 0.05 M cacodilate pH 7.3 (Sigma Aldrich, Italy) and post-fixed in osmium tetroxide solution in 0.1 M cacodilate pH 7.3 (Sigma Aldrich, Italy) for 1 h. After 3 washes in cacodilate 0.05 M of 10 min each, cells were dehydrated in a graded series of ethanol solutions in MilliQ water (30%; 50%; 75%; 95% for 15 min each, and 100% for 30 min), incubated in absolute propylene oxide (Sigma Aldrich, Italy) for 20 min and embedded in a solution of 1:1 epoxy resin (Sigma Aldrich, Italy) and propylene oxide for 90 min. This mixture was renewed with pure epoxy resin (Sigma Aldrich, Italy) overnight at room temperature and later polymerized at 60 °C for 48 h. Ultrathin sections (60–90 nm) were obtained with using Leica UCT ultramicrotome (Leica, Italy). They were then collected on Formvar Carbon coated 200 mesh copper grids (Agar Scientific, USA), stained for 25 min with uranyl acetate (Sigma Aldrich, Italy) and lead citrate for 20 min, washed and dried. Cells were imaged by JEOL JEM-2100 HR-transmission electron microscope at 120 kV (JEOL, Italy).
Results and discussion
MWCNT dispersion stability
Quantitatively evaluating the dispersion state of MWCNTs is complex. It has been reported in literature (Rastogi et al. Citation2008; Yu et al. Citation2007) that discrete and entangled CNTs have optical activity in the UV-visible range, while bundled carbon nanotubes are not active, and thus UV-Vis spectrometry may offer a method to quantify the degree of dispersion and the temporal stability of MWCNTs in solution. While it has been reported that the amount of discrete CNTs in suspension can be correlated with the intensity of UV-Vis absorbance the actual quantification of CNTs in the dispersion via UV-Vis spectroscopy remains a challenge due to the presence of contaminants such as amorphous carbon, metal catalysts and carbon nanoparticles all of which can contribute to the measured molar extinction coefficient. In practice, the contribution of the metal contaminants to the spectra should be subtracted before the concentration of carbon in the sample can be estimated from the UV-Vis absorption (Zhao et al. Citation2004). To support UV-Vis observations Transmission Electron Microscopy (TEM) studies were also performed.
The sonication power and time parameters were optimized to yield the lowest mean particle size distribution. In order to establish the relationship between the instrument power settings and the effective energy supplied to the samples a calorimetric calibration of the delivered acoustic power was performed for the probe sonicator (see Supplementary Information). As reported in literature (Betts et al. Citation2013; Taurozzi et al. Citation2011), it has also been observed in our laboratory that operating a probe sonicator in the presence of nanoparticle materials can rapidly degrade the titanium probe tip resulting in contamination of the sample by the release of titanium micro/nano particulates. In order to avoid this contamination, vial sonication was also evaluated as a potential alternative to probe sonication. The vial sonicator technique, in contrast to that of probe sonication, is a cleaner and safer technique as the sample is treated in a capped vial which protects the operator while also preventing the sample from being contaminated by probe degradation and reducing the risk of biological contamination. Moreover, the fixed geometry of the sample holder in the vial sonicator improves reproducibility in comparison to the probe sonicator where variations in, for example, probe position, immersion depth or vessel shape can influence the final dispersion quality. In contrast, conventional probe sonication permits more efficient energy transfer as the probe is directly in contact with the suspension while in the vial sonicator the sample tube acts as a partial barrier to both the input of ultrasonic energy and the dissipation of heat (). In this study both the probe sonicator and the vial sonicator were operated in a pulsed manner with 50% cycle time and approximately 1 s/cycle. In this way the vial sonicated samples could be treated with high (amplitude) intensity sonication, while the heat dissipation during the off-period of the cycle permitted the temperature to remain below 50 °C.
Table 2. Summary of features of probe and vial sonicator sonication.
MWCNTs were mixed with tannic acid solution and prepared as stock suspensions using the two different sonication methods at two different concentrations of 0.2 and 1.0 mg/mL (). Each suspension was analyzed to assess morphology and size distribution of the material in the as-prepared state (PSa, PSb and VSc) and VSc after 1 month of storage to check their stability. The VSc suspension was also analyzed after 6 (VSd) and 12 hours (VSe) of sonication using vial sonicator to evaluate whether the long sonication treatments could cut or damaged the MWCNTs as reported in literature (Yu et al. Citation2007). The equivalent experiment could not be conducted using the immersion probe sonication as such long operating times would badly degrade the titanium probe and release an excessive amount of titanium particulates into solution. Additionally, experiments to disperse higher concentrations of MWCNTs (1.0 mg/mL) in tannic acid via sonication in the vial sonicator were not efficient, and longer sonication times did not produce adequate dispersions as MWCNT bundles did not disentangle resulting in quick sedimentation. To evaluate the stability of the dispersions, the particle size distributions were measured after sonication and after a rest period of 1 month.
Results reported in show no specific differences in the mean length of the MWCNTs between the two preparation methods (using a vial sonicator and probe sonicator) and between the two stock concentrations prepared (0.2 and 1.0 mg/mL sonicated as described ()) that remain also stable after 1 month of storage indicating that there is little or no tendency to agglomeration in this time frame ( and ) (Hennrich et al. Citation2007; Nilsson et al. Citation2015; Pagani et al. Citation2012; Yu et al. Citation2007).
Figure 1. TEM images of 0.2 mg/mL MWCNT material stock suspension in tannic acid sonicated in a vial sonicator by the sonication methods: (a) VSc (b) VSd, (c) VSd, (d) variation in the % of fiber length with time (1< fiber length (µm)<5). (e–f) length size distribution after 6 (VSd) and 12 h (VSe) of sonication.
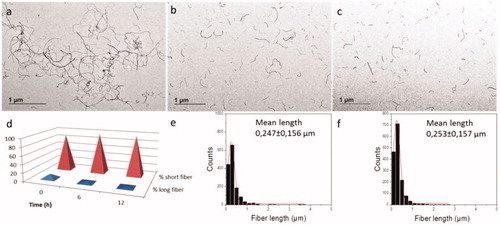
Table 3. Mean fiber length, corresponding standard deviation and % of total individual fibers counted: “short: 0.15 µm Length 0.9 µm” and “long: 1 µm Length 5 µm” of samples subject to probe sonication and vial sonicator sonication (PSa: probe 0.2 mg/mL, PSb: probe 1 mg/mL, and VSc: vial sonicator 0.2 mg/mL), persistent vial sonicator sonication for 6 (VSd) and 12 h (VSe) and subsequent storage for 1 month after sonication (PSa, PSb and VSc).
illustrates the variation of the absorbance of the supernatant suspensions with sedimentation time from probe sonication and vial sonicator treatment. The temporal stability of both samples is comparable and no tendency to sediment during the evaluated time was observed. In addition to the UV-Vis studies, TEM analysis were performed which showed that, immediately after probe sonication and after 1 month of storage, around 90% of fibers analyzed had a length which was between 0.15 and 1 µm with a mean length of 0.27–0.28 µm and a thickness between 10 and 30 nm. Considering longer times of sonication in a vial sonicator of the 0.2 mg/mL stock preparation (VSc), a decrease in the % of longer fibers is evident in terms of percentage of total analyzed fibers (dropping from 11.2% to 3.5% and 2.4% after 6 (VSd) and 12 hours (VSe) of sonication, respectively), even if the mean size was little affected. In fact, after 12 hours (VSe) it was 0.25 ± 0.16 µm, while for VSc it was 0.28 ± 0.17 µm ( and ).
Figure 2. Temporal variation of UV-Vis absorption at 800 nm of MWCNTs dispersed with Tannic acid (300 mg/L).
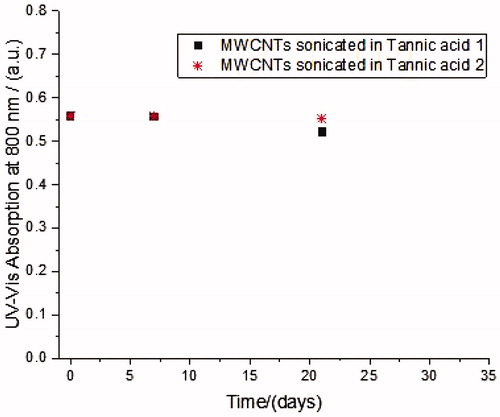
Once dispersed in complete culture medium for cell exposure, the material maintained the same morphology as the stock suspensions and remained well dispersed ().
Figure 3. TEM images of MWCNT material stock suspension in tannic acid as prepared (a,c,d) and after 48 h in culture medium (b,d,f) as follows: (a,b) PSb, (c,d) PSa, (e,f) VSc. Corresponding graphs of stocks suspensions show the length measured and the Gaussian distribution with mean length calculated.
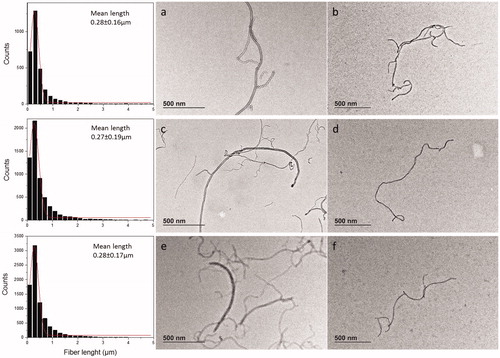
In vitro testing
In vitro toxicity experiments were performed in order to evaluate the effect of the dispersion protocol used for MWCNTs on the viability of A549 cells. The colony forming assay and the HCI analysis were the techniques used. Results obtained with both techniques showed a dose-dependent toxicity after 48 h of exposure to MWCNTs for stock concentrations of 0.2 mg/mL (PSa and VSc), (), ) and also for the corresponding unfiltered solvent controls (, ). However, the dose-dependent effect was not seen for the stock concentration of 1 mg/mL (PSb) (), ) but was observed for its unfiltered solvent control (), ). These results indicate that the cytotoxicity observed for MWCNTs was due to tannic acid concentrations corresponding to MWCNT suspensions in the range of 5–200 µg/mL. In the case of the stock suspension with the higher concentration of MWCNTs (1 mg/mL) no cytotoxicity was observed. From the data obtained in vitro shown in , we estimated the IC50 for each experimental condition using nonlinear regression of an inhibitory dose-response model (). The calculated values show that the two non-colorimetric in vitro assays, in agreement with previous literature reports, both showed tannic acid-related cytotoxicity. IC50 estimations showed that the two techniques gave comparable results, with the CFE being the more sensitive technique. This is probably due to the fact that in the CFE assay the number of cells exposed to the nanomaterials is much lower and therefore are more sensitive to the effect of nanomaterials.
Figure 4. A549 in vitro toxicity experiments using CFE (A,C) and HCI (B,D). Cells exposed to different concentrations of probe and vial sonicated MWCNTs from stock 0.2 mg/mL (PSa and VSc) and the corresponding solvent controls (A,B). Cells exposed to probe-sonicated MWNCTs from stock 1 mg/mL (PSb) and its solvent control (C,D). Cell viability was calculated by counting colonies formed after exposure as compared with the negative control for CFE assay and by determining the number of nuclei in the exposed conditions as compared to the number of nuclei in the negative control for HCl test. Results are expressed in cell viability as percentage of negative control. The tannic acid concentrations corresponding to each MWCNT concentration tested are expressed in µg/mL.
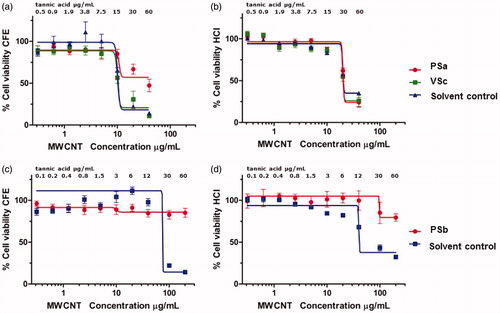
Figure 5. Nuclei-stained A549 cells exposed to MWCNTs for in vitro toxicity experiments using HCI. After incubation for 48 h with the corresponding controls or MWCNTs, cells were fixed, stained with Hoechst and imaged using INCell Analyzer. Negative control (A), solvent control for 0.2 mg/mL (B), solvent control for 1.0 mg/mL (C), PSb stock at 200 µg/mL. (D), PSa stock at 40 µg/mL concentration (E), VSc stock at 40 µg/mL concentration (F).
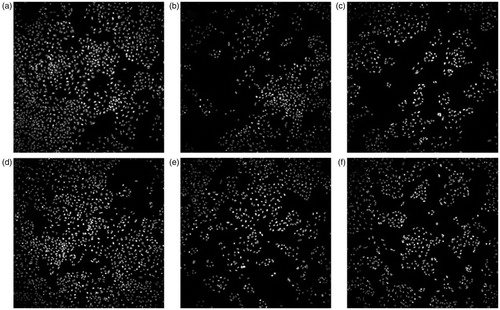
Table 4. IC50 estimated in vitro values for MWCNTs dispersed using a probe and a vial sonicator and corresponding solvent control (tannic acid).
To understand these observations, the investigation of the tannic acid effect was a key point as described earlier. UV-Vis experiments were run to elucidate whether the tannic acid was likely to be retained by the filters used in our biological tests and also whether the concentration of tannic acid in solution after sonication had decreased. The toxicity of the solvent was examined by mimicking the filtration conditions normally used to obtain solvents directly from the nanomaterial suspensions and to carry out in vitro toxicity experiments. The toxicity of the tannic acid solution obtained after Amicon 10 KDa filtration was also assessed. Results showed that, even when using the highest concentrations in each sonication protocol little or no toxicity could be observed (). To understand this behavior the UV-Vis spectra of the tannic acid solutions were recorded and showed 49.6% and 78.1% lower concentrations after filtration through a 0.2 µm filter and an Amicon 10 KDa filter, respectively. Moreover, when the absorbance of the transparent filtrate of a sonicated sample of MWCNTs (PSb) was measured, it also showed that the concentration of tannic acid had decreased.
Figure 6. (a) UV-Vis spectra of tannic acid solution (300 mg/L) before and after Amicon 10 KDa filtration, 0.2 µm filtration and filtrate of sonicated MWCNTs (PSb), (b) Cell viability percentage by CFE of A549 exposed to tannic acid after filtration and corresponding to 60 µg/mL of tannic acid.
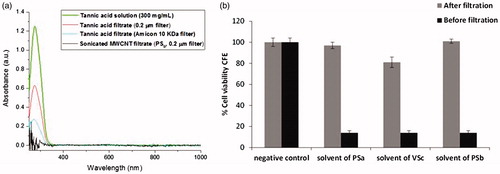
In this way it was possible to explain the apparently paradoxical toxicity results as being a consequence of two separate experimental phenomena which influence the quantity of free tannic acid present in the dispersion media. The first was the retention of tannic acid on the filters when preparing the solvent controls as often applied in biological tests - the effect was observed following filtration of pure tannic acid and after incubation with MWCNTs. The second is the additional reduction of free tannic acid due to absorption on the surface of the MWCNT -as the concentration of MWCNTs increases so does the available surface area for the absorption of the tannic acid. This reduces the concentration of free tannic acid in solution and consequently produces a less toxic solvent in agreement with the observation that higher toxicity occurred with samples prepared by sonication of a lower concentration of MWCNTs. It was, therefore, concluded that toxicity, if observed, was primarily the result of higher concentrations of free tannic acid which under our experimental conditions could be considered safe up to 6 µg/mL in the assays used in this study. These experiments were important for showing that the choice of the correct protocol for nanomaterials testing is fundamental to obtain realistic and comparable results.
To evaluate the direct interactions between the cells with the MWCNTs, their uptake was investigated by TEM. Results obtained for A549 cells incubated with MWCNTs suggested that the intracellular trafficking transport mechanism observed for these materials was both an endocytic pathway and a direct MWCNTs penetration of the cellular membrane ().
Figure 7. Uptake mechanisms observed after A549 exposure to MWCNTs; this material is clearly internalized by cells mainly by 2 different mechanisms: (i) directly passing through the membrane for single MWCNTs of around 300 nm length, that are later found inside lysosomes or late endosomes (a,b) or (ii) via endocytic pathway after membrane invagination mainly for MWCNT bundles (c) or both mechanisms together (d). (a) PSb; (b) VSc; (c,d) PSa.
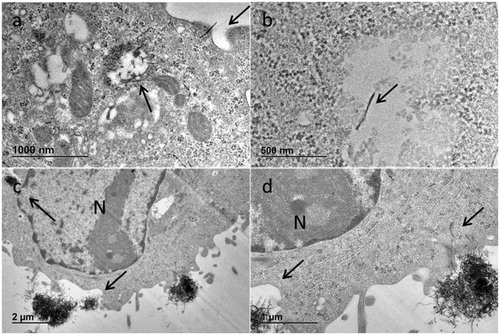
As previously described, passive uptake of CNTs is due to Van der Waals forces and CNT surface/lipid membrane interactions (e.g. hydrophobicity) resulting in CNT puncturing cells and persisting in the cytoplasm of cells (Stueckle et al. Citation2016). The two probe sonication protocols and the stock suspension concentrations did not affect the mechanism of MWCNT uptake showing both direct and indirect bio-persistent cellular internalization of both single fibers and bundles. In our study, cells showed normal healthy morphology with well-defined nucleus and organelles. MWCNTs both well dispersed and also in bundles were present in endosomes and lysosomes.
Conclusions
An efficient dispersion protocol for MWCNTs has been developed and tailored for use with two different types of sonicator -an immersion probe sonicator and a vial sonicator. When compared, both sonication treatments were found to be effective in producing dispersions for low concentrations of MWCNTs (0.2 mg/mL (PSa and VSc)) while for higher concentrations only the probe sonicator gave adequate dispersions with a high proportion of free MWCNTs and no tendency to sedimentation. The use of aqueous tannic acid as a dispersant combined with appropriate sonication was effective in firstly untangling MWCNT bundles and then stabilizing the individual fibers for up to 1 month of storage. It was found that the different sonication treatments applied to the MWCNT suspension did not have any influence on the outcome of the biological tests performed. Moreover, our biological studies have not shown cytotoxic effects from MWCNTs alone. Conversely, the aqueous tannic acid used as a dispersant was observed to produce toxic effects when present at concentrations above 6 µg/mL. This shows the relevance of considering also the effects of any free dispersant and not only the nanomaterial in question as a potential toxic compound at certain concentrations.
Such considerations are important to improving the preparation procedures of MWCNT samples so that researchers can have access to the reproducible, well defined samples which are a pre-requisite for obtaining reliable and accurate data for use in risk and hazard assessments. Possible false positive or negative toxic effects may result from different causes unless thorough physico-chemical characterization studies accompany the design and performance of toxicity testing (Betts et al. Citation2013; Petersen et al. Citation2014; Schwab et al. Citation2011; Spohn et al. Citation2009; Taurozzi et al. Citation2011; Wörle-Knirsch et al. Citation2006). For instance, this may include variations in material properties due to the unsuitable storage of the nanomaterial to be tested, the presence of impurities, unreliable dispersion processes, inappropriate test method due to the unique features of nanomaterials or, as we have observed in this work, depletion of dissolved species in culture medium because of its adsorption on the carbon nanotubes surface. In this study, depletion of the dispersant was observed to reduce toxic effects but, as other studies have shown (Casey et al. Citation2008; Horie and Iwahashi Citation2014), a similar process could result in an indirect toxicity by the nutrient depletion effect, resulting in a diminution of the ability to proliferate of the cells in the poorer culture medium. The observation that the same basic physico-chemical process, physisorption, can potentially produce opposite effects in a toxicity test emphasizes that the safety assessment of nanomaterials should be multidisciplinary in all its phases and that appropriate physico-chemical characterization also during biological testing can help to avoid false positive/false negative results due to experiment artifacts.
TNAN-2018-0178-File004.docx
Download MS Word (58.5 KB)Disclosure statement
None of the authors have any conflict of interest in the work described in this manuscript.
References
- Belin, T., and F. Epron. 2005. “Characterization Methods of Carbon Nanotubes: A Review.” Materials Science and Engineering: B 119 (2):105–118. doi:10.1016/j.mseb.2005.02.046.
- Betts, J. N., M. G. Johnson, P. T. Rygiewicz, G. A. King, and C. P. Andersen. 2013. “Potential for Metal Contamination by Direct Sonication of Nanoparticle Suspensions.” Environmental Toxicology and Chemistry 32 (4):889–893. doi:10.1002/etc.2123.
- Bravo, L. 1998. “Polyphenols: Chemistry, Dietary Sources, Metabolism, and Nutritional Significance.” Nutrition Reviews 56 (11):317–333. doi:10.1111/j.1753-4887.1998.tb01670.x.
- Buford, M. C., R. F. Hamilton, Jr, and A. Holian. 2007. “A Comparison of Dispersing Media for Various Engineered Carbon Nanoparticles.” Particle and Fibre Toxicology 4 (1):6. doi:10.1186/1743-8977-4-6.
- Casey, A., E. Herzog, F. M. Lyng, H. J. Byrne, G. Chambers, and M. Davoren. 2008. “Single Walled Carbon Nanotubes Induce Indirect Cytotoxicity by Medium Depletion in A549 Lung Cells.” Toxicology Letters 179 (2):78–84. doi:10.1016/j.toxlet.2008.04.006.
- Chatterjee, N., J. Yang, S. Kim, S. W. Joo, and J. Choi. 2016. “Diameter Size and Aspect Ratio as Critical Determinants of Uptake, Stress Response, Global Metabolomics and Epigenetic Alterations in Multi-Wall Carbon Nanotubes.” Carbon 108:529–540. doi:10.1016/j.carbon.2016.07.031.
- Cheng, J., and S. H. Cheng. 2012. “Influence of Carbon Nanotube Length on Toxicity to Zebrafish Embryos.” International Journal of Nanomedicine 7:3731–3739.
- Datsyuk, V., P. Landois, J. Fitremann, A. Peigney, A. M. Galibert, B. Soula, and E. Flahaut. 2009. “Double-Walled Carbon Nanotube Dispersion via Surfactant Substitution.” Journal of Materials Chemistry 19 (18):2729–2736. doi:10.1039/b814122n.
- De Volder, M. F. L., S. H. Tawfick, R. H. Baughman, and A. J. Hart. 2013. “Carbon Nanotubes: Present and Future Commercial Applications.” Science 339 (6119):535–539. doi:10.1126/science.1222453.
- Ema, M., H. Takehara, M. Naya, H. Kataura, K. Fujita, and K. Honda. 2017. “Length Effects of Single-Walled Carbon Nanotubes on Pulmonary Toxicity after Intratracheal Instillation in Rats.” The Journal of Toxicological Sciences 42 (3):367–378. doi:10.2131/jts.42.367.
- Fujigaya, T., and N. Nakashima. 2015. “Non-Covalent Polymer Wrapping of Carbon Nanotubes and the Role of Wrapped Polymers as Functional Dispersants.” Science and Technology of Advanced Materials 16 (2):024802. doi:10.1088/1468-6996/16/2/024802.
- Galvao, J., B. Davis, M. Tilley, E. Normando, M. R. Duchen, and M. F. Cordeiro. 2014. “Unexpected Low-Dose Toxicity of the Universal Solvent DMSO.” FASEB Journal: Official Publication of the Federation of American Societies for Experimental Biology 28 (3):1317–1330. doi:10.1096/fj.13-235440.
- Gao, Z., L. Oudjedi, R. Faes, F. Moroté, C. Jaillet, P. Poulin, B. Lounis, and L. Cognet. 2015. “Optical Detection of Individual Ultra-Short Carbon Nanotubes Enables Their Length Characterization down to 10 nm.” Scientific Reports 5:17093.
- Gommes, C., S. Blacher, K. Masenelli-Varlot, C. Bossuot, E. McRae, A. Fonseca, J.-B. Nagy, and J.-P. Pirard. 2003. “Image Analysis Characterization of Multi-Walled Carbon Nanotubes.” Carbon 41 (13):2561–2572. doi:10.1016/S0008-6223(03)00375-0.
- Handy, R. D., T. B. Henry, T. M. Scown, B. D. Johnston, and C. R. Tyler. 2008. “Manufactured Nanoparticles: their Uptake and Effects on Fish—a Mechanistic Analysis.” Ecotoxicology 17 (5):396–409. doi:10.1007/s10646-008-0205-1.
- Hartmann, N. B., K. Alstrup Jensen, A. Baun, K. Rasmussen, H. Rauscher, R. Tantra, D. Cupi, D. Gilliland, F. Pianella, and J. M. Riego Sintes. 2015. “Techniques and Protocols for Dispersing Nanoparticle Powders in Aqueous Media—Is There a Rationale for Harmonization?” Journal of Toxicology and Environmental Health, Part B: Critical Reviews 18 (6):299–326.
- Hennrich, F., R. Krupke, K. Arnold, J. A. R. Stütz, S. Lebedkin, T. Koch, T. Schimmel, and M. M. Kappes. 2007. “The Mechanism of Cavitation-Induced Scission of Single-Walled Carbon Nanotubes.” The Journal of Physical Chemistry B 111 (8):1932–1937. doi:10.1021/jp065262n.
- Herzog, E., A. Casey, F. M. Lyng, G. Chambers, H. J. Byrne, and M. Davoren. 2007. “A New Approach to the Toxicity Testing of Carbon-Based Nanomaterials: The Clonogenic Assay.” Toxicology Letters 174 (1–3):49–60. doi:10.1016/j.toxlet.2007.08.009.
- Horie, M., and H. Iwahashi. 2014. “The Impact of the Physiochemical Properties of Manufactured Nanoparticles on In Vitro and In Vivo Evaluation of Particle Toxicity.” Physical Chemistry & Biophysics 4 (2):1–4.
- Hurt, R. H., M. Monthioux, and A. Kane. 2006. “Toxicology of Carbon Nanomaterials: Status Trends, and Perspectives on the Special Issue.” Carbon 44 (6):1028–1033. doi:10.1016/j.carbon.2005.12.023.
- Hyung, H.,. J. D. Fortner, J. B. Hughes, and J. H. Kim. 2007. “Natural Organic Matter Stabilizes Carbon Nanotubes in the Aqueous Phase.” Environmental Science & Technology 41 (1):179–184. doi:10.1021/es061817g.
- Ikeda, A., T. Hamano, K. Hayashi, and J. I. Kikuchi. 2006. “Water-Solubilization of Nucleotides-Coated Single-Walled Carbon Nanotubes Using a High-Speed Vibration Milling Technique.” Organic Letters 8 (6):1153–1156. doi:10.1021/ol053089s.
- Ishibashi, A., and N. Nakashima. 2006. “Individual Dissolution of Single-Walled Carbon Nanotubes in Aqueous Solutions of Steroid or Sugar Compounds and Their Raman and near-IR Spectral Properties.” Chemistry - A European Journal 12 (29):7595–7602. doi:10.1002/chem.200600326.
- Klumpp, C., K. Kostarelos, M. Prato, and A. Bianco. 2006. “Functionalized Carbon Nanotubes as Emerging Nanovectors for the Delivery of Therapeutic.” Biochimica et Biophysica Acta (BBA) - Biomembranes 1758 (3):404–412. doi:10.1016/j.bbamem.2005.10.008.
- Krause, B., R. Boldt, and P. Potschke. 2011. “A Method for Determination of Length Distributions of Multiwalled Carbon Nanotubes before and after Melt Processing.” Carbon 49 (4):1243–1247. doi:10.1016/j.carbon.2010.11.042.
- Krause, B., G. Petzold, S. Pegel, and P. Potschke. 2009. “Correlation of Carbon Nanotube Dispersability in Aqueous Surfactant Solutions and Polymers.” Carbon 47 (3):602–612. doi:10.1016/j.carbon.2008.10.040.
- Lin, D., N. Liu, K. Yang, L. Zhu, Y. Xu, and B. Xing. 2009. “The Effect of Ionic Strength and pH on the Stability of Tannic Acid-Facilitated Carbon Nanotube Suspensions.” Carbon 47 (12):2875–2882. doi:10.1016/j.carbon.2009.06.036.
- Lin, D., and B. Xing. 2008. “Tannic Acid Adsorption and Its Role for Stabilizing Carbon Nanotube Suspensions.” Environmental Science & Technology 42 (16):5917–5923. doi:10.1021/es800329c.
- Luanpitpong, S., L. Wang, and Y. Rojanasakul. 2014. “The Effects of Carbon Nanotubes on Lung and Dermal Cellular Behaviors.” Nanomedicine (London, England) 9 (6):895–912. doi:10.2217/nnm.14.42.
- Mast, J., and L. Demeestere. 2009. “Electron Tomography of Negatively Stained Complex Viruses: Application in Their Diagnosis.” Diagnostic Pathology 4 (5):1–7. doi:10.1186/1746-1596-4-5.
- Mercer, R. R., A. F. Hubbs, J. F. Scabilloni, L. Wang, L. A. Battelli, S. Friend, V. Castranova, and D. W. Porter. 2011. “Pulmonary Fibrotic Response to Aspiration of Multi-Walled Carbon Nanotubes.” Particle and Fibre Toxicology 8 (21):1–11. doi:10.1186/1743-8977-8-21.
- Moore, V. C., M. S. Strano, E. H. Haroz, R. H. Hauge, R. E. Smalley, J. Schmidt, and Y. Talmon. 2003. “Individually Suspended Single-Walled Carbon Nanotubes in Various Surfactants.” Nano Letters 3 (10):1379–1382. doi:10.1021/nl034524j.
- Nilsson, H. M., B. Meany, J. Ticey, C. F. Sun, Y. Wang, and J. Cumings. 2015. “Ammonium Laurate Surfactant for Cleaner Deposition of Carbon Nanotubes.” Langmuir 31 (25):6948–6955. doi:10.1021/acs.langmuir.5b01175.
- Pagani, G., M. J. Green, P. Poulin, and M. Pasquali. 2012. “Competing Mechanisms and Scaling Laws for Carbon Nanotube Scission by Ultrasonication.” Proceedings of the National Academy of Sciences of the United States of America 109 (29):11599–11604. doi:10.1073/pnas.1200013109.
- Petersen, E. J., T. B. Henry, J. Zhao, R. I. MacCuspie, T. L. Kirschling, M. A. Dobrovolskaia, V. Hackley, B. Xing, and J. C. White. 2014. “Identification and Avoidance of Potential Artifacts and Misinterpretations in Nanomaterial Ecotoxicity Measurements.” Environmental Science & Technology 48 (8):4226–4246. doi:10.1021/es4052999.
- Poland, C. A., R. Duffin, I. Kinloch, A. Maynard, W. A. H. Wallace, A. Seaton, V. Stone, S. Brown, W. MacNee, and K. Donaldson. 2008. “Carbon Nanotubes Introduced into the Abdominal Cavity Ofmice Show Asbestos-like Pathogenicity in a Pilot Study.” Nature Nanotechnology 3 (7):423–428. doi:10.1038/nnano.2008.111.
- Ponti, J., F. Broggi, V. Mariani, L. De Marzi, R. Colognato, P. Marmorato, S. Gioria., et al. 2013. “Morphological Transformation Induced by Multiwall Carbon Nanotubes on Balb/3T3 Cell Model as an In Vitro End Point of Carcinogenic Potential.” Nanotoxicology 7 (2):221–233. doi:10.3109/17435390.2011.652681.
- Ponti, J., R. Colognato, H. Rauscher, S. Gioria, F. Broggi, F. Franchini, C. Pascual García, G. Giudetti, and F. Rossi. 2010. “Colony Forming Efficiency and Microscopy Analysis of Multi-Wall Carbon Nanotubes Cell Interaction.” Toxicology Letters 197 (1):29–37. doi:10.1016/j.toxlet.2010.04.018.
- Poulsen, S. S., P. Jackson, K. Kling, K. B. Knudsen, V. Skaug, Z. O. Kyjovska, B. L. Thomsen., et al. 2016. “Multi-Walled Carbon Nanotube Physicochemical Properties Predict Pulmonary Inflammation and Genotoxicity.” Nanotoxicology 10 (9):1263–1275. doi:10.1080/17435390.2016.1202351.
- Rasmussen, K. 2014. “Multi-Walled Carbon Nanotubes, NM400, NM401, NM402, NM403: Characterisation and Physico-Chemical Properties.” EUR-Scientific and Technical Research series-ISSN 1831-9424. doi: 10.2788/10986.
- Rastogi, R.,. R. Kaushal, S. K. Tripathi, A. L. Sharma, I. Kaur, and L. M. Bharadwaj. 2008. “Comparative Study of Carbon Nanotube Dispersion Using Surfactants.” Journal of Colloid and Interface Science 328 (2):421–428. doi:10.1016/j.jcis.2008.09.015.
- Richard, C., F. Balavoine, P. Schultz, T. W. Ebbesen, and C. Mioskowski. 2003. “Supramolecular Self-Assembly of Lipid Derivatives on Carbon Nanotubes.” Science (New York, N.Y.) 300 (5620):775–778. doi:10.1126/science.1080848.
- Schwab, F., T. D. Bucheli, L. P. Lukhele, A. Magrez, B. Nowack, L. Sigg, and K. Knauer. 2011. “Are Carbon Nanotube Effects on Green Algae Caused by Shading and Agglomeration?” Environmental Science & Technology 45 (14):6136–6144. doi:10.1021/es200506b.
- Shen, Z., M. Röding, M. Kröger, and Y. Li. 2017. “Carbon Nanotube Length Governs the Viscoelasticity and Permeability of Buckypaper.” Polymers 9 (12):115. doi:10.3390/polym9040115.
- Spohn, P., C. Hirsch, F. Hasler, A. Bruinink, H. F. Krug, and P. Wick. 2009. “C60 Fullerene: A Powerful Antioxidant or a Damaging Agent? the Importance of an In-Depth Material Characterization Prior to Toxicity Assays.” Environmental Pollution 157 (4):1134–1139. doi:10.1016/j.envpol.2008.08.013.
- Stueckle, T. A., L. Sargent, Y. Rojanasakul, and L. Wang. 2016. Genotoxicity and Carcinogenic Potential of Carbon Nanomaterials. In: Biomedical Applications and Toxicology of Carbon Nanomaterials. Edited by C. Chen, H. Wang. Weinheim: Wiley-VCH Verlag GmbH & Co.
- Sweeney, S.,. D. Berhanu, S. K. Misra, A. J. Thorley, E. Valsami-Jones, and T. D. Tetley. 2014. “Multi-Walled Carbon Nanotube Length as a Critical Determinant of Bioreactivity with Primary Human Pulmonary Alveolar Cells.” Carbon 78:26–37. doi:10.1016/j.carbon.2014.06.033.
- Taurozzi, J. S., V. A. Hackley, and M. R. Wiesner. 2011. “Ultrasonic Dispersion of Nanoparticles for Environmental, Health and Safety Assessment – Issues and Recommendations.” Nanotoxicology 5 (4):711–729. doi:10.3109/17435390.2010.528846.
- Taurozzi, J. S., V. A. Hackley, and M. R. Wiesner. 2012. Preparation of Nanoparticle Dispersions from Powdered Material Using Ultrasonic Disruption. vol. NIST Special Publication 1200-2. Washington DC: U.S. Department of Commerce/National Institute of Standards and Technology.
- Vaisman, L., H. D. Wagner, and G. Marom. 2006. “The Role of Surfactants in Dispersion of Carbon Nanotubes.” Advances in Colloid and Interface Science 128–130:37–46. doi:10.1016/j.cis.2006.11.007.
- Vietti, G., D. Lison, and S. van den Brule. 2016. “Mechanisms of Lung Fibrosis Induced by Carbon Nanotubes: Towards an Adverse Outcome Pathway (AOP).” Particle and Fibre Toxicology 13 (11)1–23.
- Wörle-Knirsch, J. M., K. Pulskamp, and H. F. Krug. 2006. “Oops They Did It Again! Carbon Nanotubes Hoax Scientists in Viability Assays.” Nano Letters 6 (6):1261–1268. doi:10.1021/nl060177c.
- Yu, J., N. Grossiord, C. E. Koning, and J. Loos. 2007. “Controlling the Dispersion of Multi-Wall Carbon Nanotubes in Aqueous Surfactant Solution.” Carbon 45 (3):618–623. doi:10.1016/j.carbon.2006.10.010.
- Yuan, C., J. Gao, J. Guo, L. Bai, C. Marshall, Z. Cai, L. Wang, and M. Xiao. 2014. “Dimethyl Sulfoxide Damages Mitochondrial Integrity and Membrane Potential in Cultured Astrocytes.” PLoS ONE 9 (9):e107447. doi:10.1371/journal.pone.0107447.
- Zhao, B., M. E. Itkis, S. Niyogi, H. Hu, J. Zhang, and R. C. Haddon. 2004. “Study of the Extinction Coefficients of Single-Walled Carbon Nanotubes and Related Carbon Materials.” The Journal of Physical Chemistry B 108 (24):8136–8141. doi:10.1021/jp037402o.