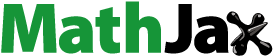
ABSTRACT
MXene is a novel type of 2D sheet nanomaterial that shows great application potentials in a variety of fields due to its advantages, such as high electrical conductivities, high surface areas, superior mechanical strength, functional transition metal surfaces, tunable surface chemistries, and excellent dispersion in various solvents. Additionally, due to its adaptability for a diverse range of applications, such as electrochemical energy storage devices, electrocatalysis, sensors, biomedical applications, membranes, flexible and wearable devices, etc., MXene is emerging as a "rising star" material. The scientific community will benefit from having a summary of the most recent research on this family of materials as these applications encourage greater interest in MXene research. In this review, we began by thoroughly summarizing the structures, characteristics, and production methods of materials based on MXenes. Then, a summary of MXenes-based materials' uses for metal air batteries has also been provided. Additionally, in order to inform the readers on the current state of MXenes' growth, we also shared our thoughts and explored their future directions. This review's data should make it easier to translate lab-scale discoveries into products that are marketable.
1. Introduction
Green, renewable energies like wind, sun, and tides are being investigated as energy use and environmental concerns rise (Citation1–3). Additionally, the outputs of those renewable energies are very weather- and climate-dependent (Citation4). In the meantime, the development of hybrid electric vehicles (HEVs) and battery electric cars (BEVs), as well as the rising demand for portable electronic products, have significantly sped up research on renewable energy storage technologies (Citation5, Citation6). Metal-air batteries (MABs) are the most anticipated batteries of the future, holding enormous promise for broad use in grid energy storage, HEVs, BEVs, and other energy storage applications (Citation7). Because of their open structure and high power and energy density, MABs hold great promise for renewable electrochemical energy storage. However, the slow kinetics of the oxygen electrode significantly limit their performance (Citation8). To overcome the problems these batteries confront, it is crucial to identify an electrode material with the right physical and chemical characteristics and great electrochemical performance (Citation9). Currently, noble metals with excellent catalytic activity include platinum and ruthenium has been deployed. The large-scale application has been severely constrained, nevertheless, by poor reserves and expensive costs (Citation10). Therefore, the creation of effective catalysts made of non-noble metals is crucial for electrocatalysis. Two-dimensional (2D) materials have received a lot of attention among the non-noble metal catalysts, such as transition-metal oxides, hydroxides, sulphides, phosphides, and others, due to their distinctive electronic and structural properties, high specific surface area, and structural adaptability (Citation11). In the field of catalysis for energy conversion, materials such as graphite-like carbon nitride (g-C3N4), 2D transition metal dichalcogenides (TMDs), black phosphorus (BP), and layered double hydroxides (LDHs) have been used (Citation12). These newly created catalysts do, however, have some glaring shortcomings, such as poor electrical conductivity, sluggish charge transfer, and unstable active sites (Citation13). Due to their exceptional physicochemical properties, which include physicochemical stability, excellent electrical conductivity, superior hydrophilicity, and large specific surface area, 2D materials have recently found wide-ranging uses (Citation14). This has sparked more research into the preparation, application and modification of 2D materials (Citation15). Transition metal (TM) carbides and nitrides, or carbonitrides, make up the novel and rapidly developing class of 2D materials known as MXenes (M n + 1XnTx, n = 1–3) (Citation16). V,Ti, Mo, Nb, and other early TMs are represented by M. Tx (-OH, -F, and -O) represents the surface functional groups added from solutions throughout the preparation process, as shown in (Citation17). X stands for the nonmetallic components of C, N, or C and N. MXenes have a wide range of potential applications due to their distinctive 2D layered structure that is similar to graphene (Citation18). Reviews have summarized and reported their use in fields like energy storage, electrocatalysis, biomedical applications, electromagnetic interference shielding, and photothermal conversion (Citation19). MXene materials possess several distinct qualities that make them highly relevant to battery applications. Some of these qualities include (Citation20):
High Electrical Conductivity: MXenes are appropriate for usage as conductive additions in battery electrodes due to their exceptional electrical conductivity. Better charge and discharge rates result from this property's ability to facilitate effective electron movement within the electrode material (Citation22).
Mechanical Strength: Because of MXenes’ exceptional mechanical strength, battery electrode structural integrity is preserved even after numerous cycles of charge and discharge. This characteristic helps the battery materials remain stable and durable throughout time (Citation23).
Surface Chemistry: MXene materials’ surfaces can be functionalized with a variety of groups to alter their electrochemical characteristics, such as regulating surface reactivity or improving ion intercalation. Because of their tunability, electrode designs can be customized to match certain battery needs (Citation21).
Large Surface Area: MXenes have considerable surface area due to their 2D layered structure. Because it offers more active sites for electrochemical reactions, this property is beneficial for battery applications as it increases energy storage capacity and improves performance (Citation24).
Ion and electronic conductivity: Ion-accessible channels are formed by the interlayer gaps in MXene structures, which aid in the diffusion of ions during the charge–discharge process. Fast ion transport inside the electrode material is encouraged by this characteristic, which enhances battery performance (Citation25). Moreover, the 2D channel separating the 2D MXene sheets can offer ions and electrons a rapid path of diffusion, giving the material exceptional conductivities for both ions and electrons (Citation26).
Chemical Stability: Chemical stability is a feature of MXene materials, especially in watery settings. For batteries to operate safely and effectively over the long term, stability is essential, particularly in applications where exposure to various electrolytes is frequent (Citation27).
Figure 1. Locations on the periodic table where the elements in MXenes, their intercalated ions, and MAX phases have their possible formulas. Three common MXene structural diagrams are included at the bottom of the page. Reproduced with permission from Ref. (Citation21).
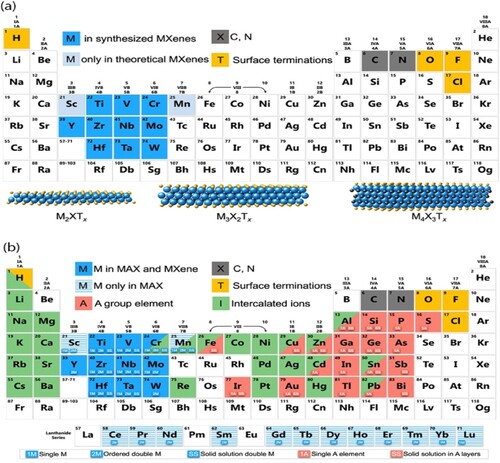
Hence, MXene materials are very relevant for next-generation battery technologies, such as lithium-ion batteries, sodium-ion batteries, and other energy storage devices, because of these unique properties (Citation28). MXenes are an attractive field of study for the advancement of energy storage technologies because of their special mix of features, which have the potential to greatly improve battery performance, energy density, and cycle life (Citation29). This article provides a systematic summary of the most recent developments in the study of MXenes and their composites in MABs. We also talk about the current difficulties and future potential of MXenes and their composites for MABs.
2. MXenes
MXene materials are a class of two-dimensional (2D) transition metal carbides, nitrides, and carbonitrides that have garnered significant attention in the field of advanced materials (Citation30). Mn + 1 X nTx is its general formula, where M stands for a number of transition metal elements, including Sc, Ti, V, Cr, Mo, Hf, Nb, Ta, and W () (Citation31). They were first discovered in 2011 and have since attracted widespread interest due to their unique combination of properties, including excellent electrical conductivity, high mechanical strength, and exceptional chemical stability. Moreover, MXenes are typically composed of transition metal carbide, nitride, or carbonitride layers, which are interleaved with functional groups such as hydroxyl (-OH) or oxygen (-O) on their surfaces (Citation32). The layers are held together by weak van der Waals forces, making them relatively easy to delaminate into individual nanosheets. MXenes exhibit a range of remarkable properties, including high electrical conductivity, good mechanical strength, excellent thermal stability, and high surface area (Citation33). These properties make them appealing for a wide array of applications, including energy storage, electromagnetic interference shielding, catalysis, sensors, and more. It can be applied for use in supercapacitors and batteries due to their high electrical conductivity and large surface area (Citation34). Additionally, MXenes have been investigated for their potential in water purification, electromagnetic interference shielding, and as catalysts for various chemical reactions (Citation35).
Figure 2. The bulk phase and MXene structures of Ti 2C (a, c) and Ti3C2 (b, d) are shown in the side view. Reproduced with permission (Citation36). Copyright 2021, Elsevier.
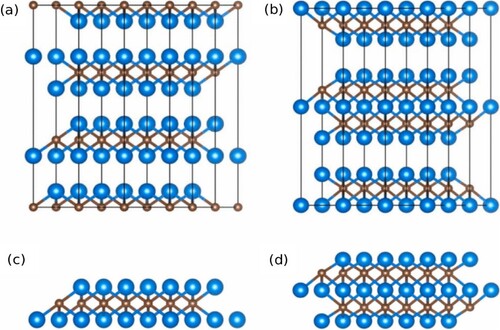
3. Properties of MXenes
MXenes have a wide and flexible surface interaction because to the abundance of electrons linked to transition metal atoms (Citation37). The MAX phase, etching and raw materials, as well as delamination and etching techniques, are responsible for controlling the intriguing features of MXenes, including their physical, chemical, magnetic, mechanical, electrical, surface, thermal, and redox capabilities (Citation38). Additionally, by adjusting the composition, choosing different ‘M’ and ‘X’ elements, and changing the surface terminal functions, the properties of MXenes can be altered for the right applications (Citation39). Recently, computer-based theoretical assessments have characterized the characteristics of MXenes. Here are some of the most alluring aspects of energy storage (Citation40).
3.1. Mechanical properties
The mechanical properties of MXene materials are an important aspect of their overall potential and performance applications (Citation41). Because of their exceptional strength, stiffness, elasticity, wear resistance, fracture toughness, thermal stability, ductility, and adhesion, MXenes are excellent options for a variety of protective, functional, and structural uses. The possible uses of MXenes in these fields are anticipated to grow with more investigation and development work focused on comprehending and enhancing their mechanical characteristics (Citation42). MXenes have the following important mechanical properties:
Wear Resistance: MXenes are appropriate for applications where materials are subjected to friction, abrasion, or erosion because of their shown exceptional wear resistance. This feature is especially beneficial for components, composites, and coatings used in abrasive environments (Citation43).
Adhesion and Bonding: MXenes are advantageous for applications like coatings, adhesives, and bonding surfaces in composite materials because they can show high adhesion to a variety of substrates (Citation44).
Thermal Stability: MXenes can withstand high temperatures without losing their mechanical integrity since they generally show good thermal stability. Applications involving high temperatures, such those in the automotive, aerospace, and industrial sectors, require this feature (Citation45).
Strength and Stiffness: Due to their excellent stiffness and strength, MXenes are a good choice for structural applications. Strong covalent connections within the MXene layers and the metallic character of the transition metal carbide or nitride layers are the sources of their exceptional mechanical capabilities (Citation46).
Ductility and Workability: It has been discovered that certain MXenes have ductile behavior, which enables them to undergo plastic deformation without breaking. This feature is useful in manufacturing operations where complicated geometries involving shaping and forming of MXene materials are required (Citation47).
Fracture Toughness: MXenes typically exhibit high fracture toughness, a property that quantifies a material's resistance to the spread of cracks. This characteristic is essential for guaranteeing the dependability and robustness of structures and parts made of MXene (Citation48).
Elasticity and Flexibility: Certain MXenes have high levels of flexibility and elasticity, which enable them to resist deformation and regain their original shape. For applications where materials must withstand mechanical stress without suffering irreversible damage, this feature is crucial (Citation49).
Depending on the surface terminations, MXenes’ mechanical properties might vary greatly (Citation50). One distinguishing feature of MXenes is their exceptional mechanical strength (Citation51). Ti3C2Tx has been actively researched for a range of applications due to its remarkable conductivity, mechanical properties, and high electro-magnetic shielding effect, despite the substantial advancements in the manufacturing of MXene nanostructures (Citation52, Citation53). During synthesis, MXene invariably bears terminal groups Tx (-F, -OH, and -O), which is helpful for stabilizing bare MXene compounds (Citation54). Additionally, it can prevent the tensile strain-induced collapse of the atomic layer on the surface of bare MXene materials, increasing the materials’ mechanical flexibility (Citation55). Therefore, it is critical to investigate how terminal groups affect performance (Citation56). Numerous investigations have shown that while the elastic coefficient of intrinsic MXene is significantly reduced by the terminal group, it is still higher than that of the original MAX phase and other two-dimensional materials, including graphene-free materials like MoS2 (Citation56). By measuring the Young's modulus of Ti3C2Tx via nanoindentation tests, it is shown that the typical terminal groups’ elastic coefficient relationship is -O > -F > -OH (Citation57). The bending capabilities of MXene can also be significantly improved by the presence of surface terminal groups, giving MXene materials excellent application possibilities in composite structures and flexible electronics (Citation58).
3.2. Optical properties
MXene materials’ optical characteristics are yet another crucial factor that adds to their adaptability and possible uses (Citation59). Furthermore, MXenes are interesting candidates for a variety of optoelectronic, photonic, and photothermal applications due to their combination of adjustable optical characteristics, nonlinear optical activity, plasmonic behavior, transparency, and photothermal conversion. Within the visible and near-infrared portions of the electromagnetic spectrum, certain MXenes show outstanding transparency (Citation60). Because of this characteristic, they can be used in transparent conductive coatings for solar cells, displays, touch screens, and other optoelectronic devices (Citation61). MXenes are also interesting for photodetection and photovoltaic applications due to their significant light absorption in the visible and ultraviolet spectrums. Furthermore, it has been demonstrated that MXenes display plasmonic activity, which means that when they interact with light, they can sustain surface plasmon resonances. This characteristic holds significance in fields like as plasmonics, metamaterials, and sensing technologies, where nanoscale light manipulation plays a crucial role (Citation46). Some MXenes have shown effective photothermal conversion capabilities because of their high near-infrared absorption capability. This makes them attractive options for use in photothermal therapy, water filtration, and other processes that take advantage of light-induced heating. Through a variety of techniques, including heterostructure creation, surface functionalization, and intercalation, the optical characteristics of MXenes can be adjusted. These tactics enable their optical behavior to be altered to satisfy certain application needs (Citation62). Furthermore, it has been discovered that MXenes display nonlinear optical behavior. This is noteworthy for nonlinear optics applications such ultrafast optical data processing, frequency conversion, and optical switching (Citation63). Ti3C2Tx MXenes have exceptional transparency and a photothermal effect as optical characteristics (Citation64). Strong surface plasmonic effects are a crucial characteristic of Ti3C2Tx films (Citation65). Despite the fact that transparency reduces as the spin rate of the coating increases, Ying et al. were able to achieve transparency of more than 75% in the visible and near-infrared bands. A V2CTX transmittance investigation shows that the produced film is very transparent at 550 nm and that the transmittance diminishes with increasing film thickness (Citation66). An 11-nm-thick layer with a maximum transmittance of 89% was achieved. At 550 nm, the film's absorbance coefficient was 1.22 0.05 105/cm. In comparison to the Ti2CTx (2.7 0.1 105/cm) and Ti3C2Tx (2.4 0.1 105/cm) MXenes, this value is lower. This experiment shows that neither the transmittance nor the absorption coefficient are affected by the annealing temperature of the MXenes film (Citation67).
3.3. Thermal and electrical properties
MXenes show intriguing electrical and thermal characteristics that bode well for a variety of uses (Citation68). The exceptional properties of MXenes, including thermal stability, electrical conductivity, and electrical characteristics that may be customized, make them highly versatile materials for a variety of applications in electronics, thermal management, and energy storage. Future applications for MXenes are expected to grow as a result of ongoing research into understanding and enhancing these attributes (Citation37). The following are some salient features of their electrical and thermal behavior:
3.3.1. Thermal properties
Thermal Conductivity: MXenes are recognized for their excellent heat conductivity, which is a result of their metallic makeup and two-dimensional layered structure. Because of this characteristic, MXenes are appealing for uses where effective heat dissipation is necessary, including in thermal interface materials and electronic devices (Citation69).
Thermal Stability: MXenes may tolerate high temperatures without experiencing appreciable deterioration since they typically show good thermal stability. Applications in extreme heat conditions, such thermal management systems or high-temperature catalysis, benefit from this property (Citation70).
Thermoelectric Characteristics: Promising thermoelectric qualities that allow for the conversion of heat into electrical energy and vice versa have been demonstrated by several MXenes. Because of this, they could be suitable options for thermoelectric devices that are used to generate energy and recover waste heat (Citation62).
3.3.2. Electrical properties
Electrical Conductivity: Due to its layered structure and metallic nature, MXenes are well known for having a high electrical conductivity. Their ability to function as conductive additives, electrodes in energy storage devices, and parts of electronic circuits makes them useful in a variety of electrical and electronic applications (Citation71).
Tunable Electrical Properties: By changing their surface terminations, intercalating other species between the layers, or constructing heterostructures, MXenes’ electrical characteristics can be adjusted. Because of its tunability, electrical characteristics can be customized to match the demands of certain applications (Citation72).
Ti3AlC2 has a strong thermal conductivity and thermal oxidation. In comparison to the Ti3C2Ox monolayer, the Ti3C2Fx monolayer has a thermal conductivity of around 11 W/m K (Citation67). However, in studies, it is discovered to be roughly 2.84 W/(mK) for Ti3C2Tx films. Ti3C2Tx sheets have a 2400 S cm1 metallic conductivity and are very flexible (Citation73). A kind of MXene called V2C has been examined, and it was discovered to have a conductivity of 3300 S cm−1 (Citation74). Because of its adaptability, it is ideal for uses like wearable electronics. The layer thickness and calcination temperature have a significant impact on the conductivities of the sheets (Citation75).
3.4. Electronic and structures properties
The typical electrical and structural characteristics of the MXene family of two-dimensional (2D) transition metal carbides, nitrides, and carbonitrides make them appealing for a variety of uses in electronics, energy storage, catalysis, and other fields (Citation76). Due to its exceptional mechanical, surface chemistry, tunable band structure, high electrical conductivity, and structural flexibility, MXenes are incredibly flexible materials with great potential to advance electronic and structural applications in a variety of sectors (Citation77). It is anticipated that additional investigation into the production, modification, and integration of MXene will further unlock their potential for use in next-generation structural and electrical materials. Among MXenes’ essential structural and electrical characteristics are:
Tunable Band Structure: By altering the surface terminations of MXenes and intercalating other species between the layers, one can adjust their electrical characteristics. Due to its tunability, band gaps, carrier concentrations, and electronic states can be changed to create customized electronic characteristics for various uses. For instance, MXenes’ band structure can be modified to improve how well they function in energy storage devices or to enable particular catalytic features (Citation78).
Electronic Conductivity: The high electrical conductivity of MXenes is a result of their metallic composition (Citation79). This metallic characteristic is caused by transition metal layers present in the MXene structure, which enable effective charge transfer and conductivity. Because of this characteristic, MXenes are attractive options for electrical and electronic uses, including conductive additives in composites and electrodes in energy storage devices (Citation80).
Surface Chemistry: The electrical characteristics and reactivity of MXenes are largely determined by their surface terminations (Citation81). MXenes’ surface functional groups can affect how they interact with other materials, such as reactants in catalytic applications or electrolytes in energy storage devices. Furthermore, surface functionalization can affect MXenes’ performance and stability in a range of settings (Citation82).
Structural Flexibility: The layered structure of MXenes allows for the intercalation of various ions, molecules, and functional groups between the layers due to its high degree of structural flexibility (Citation83). Because of its structural flexibility, MXene's properties like its surface chemistry and electrical conductivity can be changed to suit the needs of a variety of applications (Citation84).
MXenes with great structural and chemical stability can work as both carriers of intrinsic active and/or other functional materials for many applications, including energy conversion and storage, due to their superior conductivity and rich surface functions (Citation85). The disadvantages of ultrathin 2D materials, on the other hand, are MXenes’ considerable tendency to restack and the absence of a constrained porosity structure. Porous MXenes have undergone extensive design and modification during the past three years (Citation86). To date, several porous MXenes with acceptable topologies have been created utilizing a variety of synthetic processes and used to a wide range of applications, all of which have shown improved performances (Citation87). In order to further research and provide a solid foundation for real-world applications, Fanxing Bu pioneer aims to provide a new understanding of the interaction between the fundamental synthesis, composition, structure, and performance of porous MXenes (Citation88). Due to their advantageous architectures and customizable physicochemical properties, 2D porous MXenes have also been used for a variety of applications. The unit cell of the bare monolayer MXene typically resembles a hexagon. An edge-shared M6X octahedron is formed by three M atomic layers intercalated with two X atomic layers, which is what quintuple layers in an M3X2 system typically look like ((a)). The M2X system forms an edge-shared M6X octahedron by sandwiching the X layer between the M bilayer ((b)). In particular, n-biphenyl was found in TiC3 MXenes ((c)), which not only lowers the energy of the monolayer but also increases the number of C atoms on the surface. Additionally, since one M ion typically has a coordination number of six, it has been deduced that there are six chemical bonds between M-X and the surface chemical groups, which results in the formation of M2XF2, M2XO2, and M2X(OH)2 (Citation89). Two different sorts of hollow sites can be seen on the surface of M2X in (b), with hollow sites A and B each having an accessible X atom. As a result, four potential configurations for the surface groups of the M2X system have been proposed ((d)), including Model 1's two surface groups on top of two M atoms, Model 2's surface groups on top of hollow site A, Model 3's surface groups on top of hollow site A and B, and Model 4's surface groups on top of hollow site B (Citation90). While this was going on, DFT simulations showed that the stability of the M2X system decreased with the addition of the -OH, -O, and -F terminations, indicating that the more advantageous -O and -OH surface groups would likely replace the unfavorable -F surface groups (Citation91).
Figure 3. (a) A side view of the naked Ti3C2 monolayer with a quintuple layer made up of the stacking modes Ti(1)-C-Ti(2)-C-Ti(1) is shown on the left, and the calculated spin density distribution is shown on the right. (b) Top and side views of the 2D M2X layer that was produced. The A-type and B-type hollow sites shown by the dotted lines are those that are mentioned in the text. (c) The TiC3 monolayer's zigzag Ti atom chain and n-biphenyl structural unit. (d) Top and side views of the Model 1, Model 2, and Model 4 of the functionalized MXene systems. (e) Left to right side views of I-Ti3C2F2, II-Ti3C2F2, and III-Ti3C2F2 (f) From left to right, I-Ti3C2(OH), II-Ti3C2(OH), and III-Ti3C2(OH). Reproduced with permission (Citation92). Copyright 2021, Wiley.
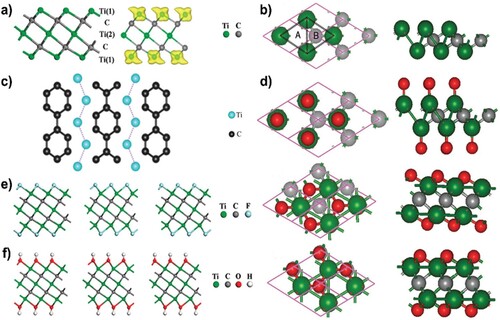
4. Mxene synthesis mechanism
Etching the MAX precursor is the most common way to make MXene. The formula for MAX is Mn + 1AXn, where n is a number between 1 and 4, and M and X are the same compositions as previously indicated; A is primarily made up of elements of the main group 13–15, such as Si, Al, Ge, Sn, etc. ((b)) (Citation93). Three different types of unit cells make up the six-square, densely packed MAX phase, which belongs to the space group P63/mmc (Citation94). In the MAX phase, the X atom is located in the octahedral position and is sandwiched between the tightly packed M layers and the A atomic layer (Citation95). According to the MAX crystal structure, Mn + 1AXn is also known to have a layered structure, where the A layer connects the two-dimensional Mn + 1AXn layer. The covalent and ionic bond qualities of M-X make it more stable than the covalent bond properties of M-A, which is a metal bond. This property enables the production of MXene by removing the A atoms from the MAX phase (Citation96). Due to the strong metallic connections between the ‘M’ and ‘A’ atoms, MXene cannot be produced in the same way as other 2D materials while having the inherent benefits of 2D materials, such as a high specific surface area and good processability (Citation97). Early research involved the use of strong acids (HF aqueous solution, for example) to dissolve the Ti-Al bonds, which were then followed by the selective removal of the Al-element layers to create multilayered Ti3C2 flakes (Citation98). The successful synthesis of Ti3C2 opens the door to the potential of etching numerous MAX phases, which make up a wide family of phases (Citation99). The majority of synthesis techniques rely primarily on water as the primary solvent and fluoride-based substances as etchants for selective etching. These techniques can result in the production of toxic fumes, intercalated water, and an abundance of hydroxyl groups on the surfaces of MXene (Citation100). To get around these problems, new techniques have been developed that make the exfoliation process more efficient, environmentally friendly, and full of terminations containing MXenes. These techniques mainly involve the use of fluoride-containing acidic solutions and its derivatives, chemical vapor deposition (CVD), hydrothermal synthesis, and alkali etching (Citation101). However, acid etching and its offshoot techniques are still often used in modern research. The morphology, surface groups, and structural flaws of MXenes will be directly impacted by the etching agents and synthesis process (). The types and ratios of M to X elements can also be used to modify the properties of MXenes. The enhanced characterizations of the MXene-based materials also disclose other potential features, which aid in and direct research on them as well as processing (Citation102). There are generally three different MXene synthesis methods: bottom-up, top-down,and etching (Citation103).
Figure 4. Diagrammatic representations of (a) a technique for creating MXenes that involves selective etching and delamination. (b) The handling of MXene clays and a secure etching technique. (c) MXene synthesis via electrochemical etching. Reproduced with permission (Citation1). Copyright 2023, John Wiley and Sons.
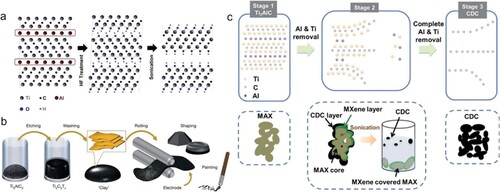
4.1. Top-down approach
The top-down approach has a long history of success, particularly in the synthesis and design of nanomaterials. The fragmentation of 2D or 3D bulky precursor material into vitally small quantum particles is a common step in these processes (Citation104). The top-down method has been successfully used to create quantum dots from a variety of three-dimensional and two-dimensional bulk precursors, including graphite, carbon nanotubes, graphene, MoS2 crystals, WS2 powder, black phosphorus, and g-C3N4 (Citation105). A few of these are ball-milling, liquid exfoliation, chemical etching, electrochemical, intercalation, hydrothermal/solvothermal treatment, ultrasonication, and microwave irradiation (Citation106). Most top-down techniques create the initial O2-containing functions on catalytic surfaces, which makes it easier for defects to form in the catalysts (Citation107). The bulk molecules can divide into tiny quantum pieces thanks to the surface imperfections acting as reactive sites. Because it may be used at low temperatures, this approach is very important (Citation108). Additionally, this method allows for the use of a significant quantity of raw materials and wide-scale production. However, there are downsides, including the limited yield and the necessity for certain treatments (Citation109). The top-down fabrication techniques for MXene that are most frequently used are ultrasonication, ball-milling, intercalation, hydrothermal, solvothermal, micro-explosion, and acid reflux (Citation110).
4.2. Bottom-up approach
In this procedure, the precursor film of Mxene is crystallised by starting from an atom and using CVD, the self-templating method, or plasma-enhanced deposition (Citation111). The bottom-up methods use molecular material as antecedents as opposed to the top-down techniques, which use bulk material as their starting point. Bottom-up methods have a variety of benefits, which improve the structures and characteristics of QDs (Citation112). These benefits include increased atomic use, structural and morphological control, and quicker functionalization (Citation113). An excellent foundation for bottom-up MXene preparation is provided by the earlier investigations (Citation114). However, simple, highly effective precursors with low toxicity, mild reaction conditions, outstanding crystallinity, monodispersity, and high yields need to be addressed for large-scale manufacturing. One-pot bottom-up strategies will probably be used to get MXene ready in the future to meet the incremental application needs because to their more easy operating conditions compared to the top-down approaches (Citation115). summarizes the key distinctions between the material attributes addressed and the bottom-up and top-down synthesis techniques of MXene.
Table 1. A comparison between the top-down and bottom-up synthesis techniques for MXene (Citation116).
4.3. Etching method
There are several methods for preparing MXenes (Citation46). Different terminal functions can be added to the M atoms to complete their coordination spheres and reduce their surface Gibbs free energy as a result of modifications in their etching techniques (Citation117). The A atomic layer is selectively eliminated from the precursor during top-down MXene production, leaving the M n + 1Xn layers unharmed. Since the nominal oxidation state of the A element in MAX is close to zero (for instance, Al0 in Ti3AlC2), the etching process involves oxidizing the A atom to another state, such as Al3+ or Si4+ (Citation118). The strength of the M-A bond in comparison to the M-X bond, along with other factors like the Gibbs free energy of by-product formation, affect the etching reaction's Gibbs free energy, which, in the case of HF etching of Al-based MAX phases, determines the ability to remove the A element (Citation119). In a broader sense, exfoliation also relies on the A element's oxidation and subsequent ligation into soluble byproducts to be removed from the precursor (Citation120). The latter is crucial because if the oxidized byproducts and/or the byproducts of the oxidized species’ subsequent hydrolysis-specifically, aluminium oxides and hydroxides-restrict the etchant's access to the precursor reaction sites (subnanometer 2D galleries) for further etching, the etching process stops right away (Citation121). The MAX-phase particles’ protective native oxide coating should be able to be removed by the etching solution as well (Citation122).
5. MXene-Based materials for MABs
5.1. Lithium-air batteries (LABs)
LABs are potentially another next-generation electrochemical technology for sustainable energy storage because they have a theoretical specific energy greater than 3500 Wh kg −1 (Citation123). This is because it has a higher specific energy than the LIBs that are already in use. However, at this point, O2 is frequently utilized as a cathode input in place of air as a proof of concept. An O2 environment can directly increase the amount of dissolved oxygen in the electrolyte, lowering the cathode catalyst's operating pressure (Citation124). For typical operation in air, high-performance catalysts are therefore much sought after, and this is also the area of interest for next-stage research. However, there are significant issues that prevent the commercialization of LABs, including the poor chemical stability of electrodes and electrolytes, low round-trip efficiency, poor rate capability, and severe capacity degradation (Citation125). More stable electrodes and catalysts can be employed to enhance electrochemical performance, reduce overpotential, and accelerate the oxygen evolution reaction (OER) during the charge process and the oxygen reduction reaction (ORR) during the discharge process (Citation126). With remarkable performance, MXenes and their composites have recently been employed extensively in LABs. A promising strategy for enhancing catalytic activity is the functionalization of 2D materials. DFT computations are used. Using in situ hybridization of LaSrCoO with Ti3C2Tx nanosheets, Sun et al. produced perovskite La0.5Sr0.5CoO3 (LaSrCoO)/Ti3C2Tx cathodes. The exceptionally low overpotential of the as-prepared hybrid catalyst is 0.87 V, which is around 540 and 430 mV lower than that of Ti3C2Tx and LaSrCoO, respectively. The 500 mAh/g−1 LaSrCoO/Ti3C2Tx cathodes exhibit outstanding cycle stability and a high initial discharge capacity (Citation127). The results show that perovskite performance in LABs is undoubtedly improved by MXene nanosheets acting as a substrate. Ni/Ti3C2Tx hybrid material was successfully synthesized by Wen et al. and used as a superior cathode in Li-O2 batteries. High surface area and superior electronic conductivity may be produced by the layer structure of Ti3C2 MXene, which is advantageous for the deposition and decomposition of Li2O2, the discharge product of LABs (Citation128). Additionally, nickel nanoparticles with a size of about 20 nm have unique catalytic properties that can noticeably speed up the breakdown of the produced Li2O2. The Ni/Ti3C2Tx hybrid material utilizes the two different types of materials to obtain maximum specific capacities of up to 20 264 mAh/g at a rate of 0.1 A/g and 10 699 mAh/g at a rate of 0.5 A/g for the initial cycle (Citation129).
5.2. Zinc-air batteries
A device with a high theoretical energy density of 1 084 Wh/kg−1, the rechargeable zinc-air battery (ZAB) is ecologically benign and has a wide range of development opportunities. However, similar to LABs, the ORR and OER both experience severe sluggish kinetics issues and high energy barriers during the discharge and charge processes, respectively (Citation130–133). For the creation of rechargeable ZABs, a properly constructed air electrode with dual-functional ORR/OER catalytic activity is essential (Citation134, Citation135). MXenes, which are new 2D nanosheets, have drawn a lot of attention and are frequently used as powerful catalysts in ZABs. Because MXenes materials have special qualities that can solve a number of significant issues related to this kind of battery technology, they have shown a lot of promise for use in Zinc-Air batteries (Citation136). Zinc-Air batteries have a high energy density, are inexpensive, and are environmentally friendly, making them appealing for use in energy storage applications. Nevertheless, the slow kinetics of the oxygen reduction reaction and the unstable air electrode provide challenges. MXenes can potentially address these challenges in the following ways (Citation3):
High Surface Area: The high surface area of MXenes can facilitate efficient gas diffusion and electrolyte interaction within the air electrode, improving the overall electrochemical performance of the battery (Citation137).
ORR Catalyst: For the oxygen reduction reaction, mxenes have shown good catalytic activity, especially when functionalized with certain groups. This characteristic can greatly increase the air electrode's efficiency in zinc-air batteries, improving both their overall performance and energy density (Citation138).
High Electrical Conductivity: Because MXenes have a high electrical conductivity, the air electrode can transmit charges more effectively. This characteristic can improve the battery's overall conductivity, which will increase its power output and charge/discharge rates (Citation139).
Mechanical Robustness: MXenes provide mechanical stability and robustness, which can support the air electrode's long-term performance and endurance in zinc-air batteries. This can help with problems with structural integrity and electrode deterioration over several cycles of charge and discharge (Citation130).
By taking advantage of these characteristics, MXenes can greatly improve Zinc-Air batteries’ longevity, performance, and efficiency, increasing their competitiveness for a variety of energy storage uses (Citation140). It's crucial to remember that additional study and development are required to guarantee cost-effectiveness for real-world application, optimize the integration of MXenes into Zinc-Air battery systems, and solve any potential stability and scalability issues. In spite of this, research into MXenes’ potential for Zinc-Air batteries is worthwhile given the wider field of energy storage technologies (Citation141). In order to create a superior bifunctional electrocatalyst suitable for both the ORR and OER processes, Wu et al. successfully created a NiFeMn trimetallic nitride electrocatalyst with a unique sheet shape stabilized by Ti3C2 sheets. High capacity of 570 mAgZn−1 at 5 mAcm−3 may be attained while operating as the air cathode material in a flexible fiber-shaped ZAB with gel as the electrolyte (Citation142). The volumetric energy density is calculated to be 44.0 mWh/cm−3, and the associated specific energy density is determined to be 627 Wh/kgZn−1 (Citation143).
N-CoSe2/3D Ti3C2Tx composites were successfully created by Zeng et al. (Citation144) by fusing 3D MXene structures with nitrogen-doped cobalt selenide nanocrystals. The 3D MXene architecture's high porosity, huge SSA, and superior conductivity allow it to anchor many CoSe2 nanocrystals and prevent their buildup between MXene sheets. While N doping further lowers the energy barriers for the OER and ORR, the strong contact between CoSe2 and MXene results in charge transfer at the interface and amplification of the catalytically active Co sites (Citation145). Hence, N-CoSe2/3D Ti3C2Tx electrode has high energy and power density, steady voltage output, and outstanding rechargeable stability. With the use of a MOF-based method, Zou et al. successfully coupled layered porous NiCoS with a stripped Ti3C2Tx MXene sheet (Citation146). The NiCoS/Ti3C2Tx hybrid material exhibits high electrical conductivity and can efficiently facilitate electron transport thanks to the stable interfacial interaction between NiCoS and Ti3C2Tx. The Ti3C2Tx matrix can be utilized to modify the composite material's structure to produce additional active sites (Citation147). As a result, a NiCoS/Ti3C2Tx-based rechargeable ZAB exhibits a very tiny charge/discharge voltage gap and excellent operation durability for long-term operation (Citation148).
5.3. Sodium-air batteries
Recent research has mostly focused on two varieties of sodium metal-based air battery systems: hybrid batteries, also known as Na-air batteries, and nonaqueous batteries, also known as Na-O2 batteries (Citation149). shows how the design and functionality of the cell structures of the two types differ slightly. A particular kind of metal-air battery called a sodium-air battery uses sodium as the anode and airborne oxygen as the cathode (Citation150). The electrochemical reactions that produce electrical energy between sodium and oxygen are the basis for the operation of these batteries. Sodium serves as the anode in sodium-air batteries (Citation151). In the discharge process, sodium atoms undergo electron loss, resulting in the formation of sodium ions (Na+). These sodium ions move from the electrolyte to the cathode. In sodium-air batteries, airborne oxygen functions as the cathode. Sodium oxide (Na2O) is produced at the cathode when oxygen (O2) from the surrounding air combines with sodium ions during battery discharge. Electrons released by this process flow through an external circuit to produce electrical current (Citation152).
Figure 5. Diagrammatic representation of (a) nonaqueous Na–O2 cell and (b) hybrid Na–air cell. Reproduced with permission (Citation153). Copyright 2020, John Wiley and Sons.
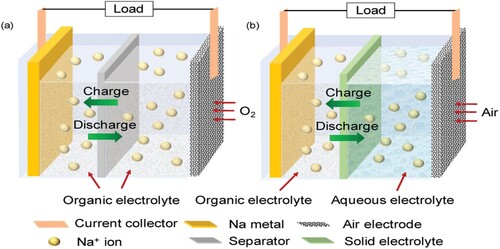
These two electrodes are submerged in an organic electrolyte and kept apart by a polymeric separator ((a)). The hybrid Na-air cell, on the other hand, uses two electrolytes: an aqueous electrolyte for the catholyte and an organic electrolyte for the anolyte. In sodium-air batteries, the electrolyte is essential for allowing sodium ions to move more easily between the anode and cathode during charge–discharge cycles. Additionally, it aids in preserving the battery's overall electrochemical equilibrium (Citation154). A Na-ion conducting solid electrolyte membrane that divides the positive and negative compartments with various electrolytes is part of the arrangement that is being shown ((b)). The positive electrode is placed in an aqueous electrolyte, whereas the negative compartment is constructed similarly to traditional Na–O2 batteries. In order to prevent a significant energy loss from the spontaneous exothermic reaction, the solid electrolyte membrane is used to prevent direct contact between the metallic Na and the aqueous electrolyte (Citation155). Overall Reaction: A sodium-air battery's entire reaction can be shown as follows:
Compared to conventional lithium-ion batteries, sodium-air batteries provide a number of benefits, such as a possible high energy density, inexpensive cost because sodium is abundant, and environmental friendliness (Citation156). Despite its potential, sodium-air batteries have drawbacks such a short cycle life, problems with the electrolyte's stability, and the need for more study to maximize their scalability and performance for real-world use (Citation157). All things considered, sodium-air batteries are a promising technology for energy storage applications, particularly when affordability and high energy density are important considerations. The issues surrounding sodium-air batteries are the main focus of ongoing research and development activities in an attempt to fully realize their promise as future energy storage options. Because of these special qualities, MXenes, a type of two-dimensional transition metal carbides and nitrides, have shown considerable promise for a variety of energy storage applications (Citation116). Sodium-air batteries, a kind of metal-air battery that uses sodium as the anode and oxygen from the air as the cathode, are one possible usage for MXenes. MXenes are appropriate for use in sodium-air batteries due to a number of features:
ORR Activity: MXenes have demonstrated strong catalytic activity for this important metal-air battery process. This may contribute to raising the battery system's overall efficiency (Citation158).
Large surface area: The huge surface area of mXenes can result in an increased number of active sites for electrochemical processes. This may improve the cycle stability and capacity of the battery.
Good chemical stability: MXenes have good chemical stability across a broad variety of electrolytes, which is critical for preserving the electrode materials’ structural integrity over time through multiple cycles of charge and discharge (Citation159).
High Electrical Conductivity: MXenes have a high electrical conductivity, which is essential for effective battery system charge transfer. This may enhance sodium-air batteries’ overall functionality and energy economy (Citation160).
MXenes possess the capability to improve sodium-air batteries’ performance, increasing their durability, cost-effectiveness, and efficiency. Sustained investigation and advancement in this field will be essential to enhance the utilization of MXenes in sodium-air batteries and hasten their commercialization for useful energy storage uses (Citation161).
6. Perspectives
MXenes materials have the potential to completely transform the energy storage industry and beyond in the near future. Their exceptional electrical conductivity, huge surface area, and mechanical toughness, along with their unique combination of qualities, make them extremely intriguing prospects for a variety of applications. MXenes have previously shown remarkable performance in the field of energy storage in lithium-ion batteries and supercapacitors, providing high specific capacitance, energy density, and reversible capacity. This implies that they might be essential in furthering the creation of energy storage systems that are more effective and durable. Furthermore, MXenes’ adaptability goes beyond energy storage, since they may find use in electromagnetic shielding, sensors, catalysis, and water purification, among other areas. This emphasizes how they may influence a variety of businesses and tackle a broad range of technology issues. It's crucial to recognize that there are still challenges to be solved, such as production scalability, long-term stability, and cost-effectiveness. In order for MXenes to reach their full potential and be widely used in real-world applications, certain obstacles must be overcome. In general, there is a lot of promise and possibility for MXenes materials. Sustained investigation and advancement endeavors will be crucial in unleashing the complete potential of MXenes and capitalizing on their revolutionary influence throughout diverse technological fields. MXene materials have intriguing qualities for battery applications, but in order to reach their full potential, a number of issues must be resolved. Among the main difficulties with MXene materials are the following:
Stability Issues: When exposed to air and moisture, MXene materials are prone to oxidation and hydrolysis, which results in the deterioration of their characteristics. Maintaining MXenes’ performance in real-world battery applications requires strategies like surface passivation or encapsulation to increase their stability in ambient environments.
Electrochemical Mechanisms: Even with all of the study done on MXene materials, more needs to be done to fully comprehend the mechanisms underlying charge storage processes and the electrochemical behavior of these materials. To better understand MXenes’ basic electrochemical characteristics and maximize their effectiveness in battery applications, more research is required.
Interlayer Swelling: When exposed to specific electrolytes, the interlayer gap in MXene frameworks can inflate significantly, which can cause mechanical instability and impede ion transport. The structural integrity and electrochemical performance of MXene-based electrodes must be preserved by addressing interlayer swelling through structural alterations or electrolyte engineering.
Binder Compatibility: To guarantee adequate adhesion and mechanical stability during electrode production, MXene materials may require appropriate binders. To achieve high-performance battery electrodes, appropriate binder methods that can successfully incorporate MXene particles into sturdy electrode structures must be developed.
Scalability: Scalable manufacture of high-quality MXene materials is one of the main obstacles. Large-scale production may be hampered by the current synthesis methods’ frequent use of harsh chemical treatments and strict control over reaction conditions. Achieving commercial viability for MXene-based battery materials requires the development of synthesis techniques that are both scalable and economical.
To address these challenges, the following strategies have been forwarded:
Materials development and characterization: Developing improved synthesis methods that enable the scalable production of high-quality MXene materials with controlled properties and compositions. Moreover, Employing advanced characterization techniques to gain deeper insights into the electrochemical behavior and mechanisms of MXene-based electrodes, guiding the design of improved battery materials.
Surface and structural Engineering: Implementing surface functionalization or coating techniques to enhance the stability of MXenes in different environments, including air, moisture, and electrolytes. Additionally, Exploring structural modifications and intercalation strategies to mitigate interlayer swelling while maintaining ion accessibility and mechanical strength.
Electrode formulation: Innovating electrode formulation approaches that optimize the integration of MXene materials with suitable binders and conductive additives for enhanced electrochemical performance.
By addressing these challenges and implementing effective strategies, researchers aim to unlock the full potential of MXene materials for next-generation energy storage technologies, contributing to the advancement of high-performance and sustainable battery systems.
7. Conclusions
We address the chemical, mechanical, electrical, thermal, and optical characteristics of MXenes in this review along with an introduction to general preparation techniques. Next, we provide an overview of MXenes materials in energy storage applications. In the perspectives section, there is also an overview and summary. Hence, MXenes have high reversible capacity and long-term stability, they have also been employed as anode materials in lithium-ion batteries. Moreover, MXenes materials possess a unique set of features that could transform energy storage systems. They can be used in a variety of energy storage devices, such as supercapacitors, lithium-ion batteries, and more, due to their high conductivity and vast surface area. The stability of MXene-based electrodes over extended cycling and the scalability of MXene synthesis are two issues that still need to be resolved. Nevertheless, continuous research and development initiatives are still underway to fully investigate the energy storage potential of MXenes, with the goal of commercializing these materials for use in real-world applications soon.
Acknowledgements
This work reported in this paper was supported by Bahir Dar Energy Center, Bahir Dar Institute of Technology, Bahir Dar University, Ethiopia.
Disclosure statement
No potential conflict of interest was reported by the author(s).
References
- Li, J.; Wang, C.; Yu, Z.; Chen, Y.; Wei, L. MXenes for Zinc-Based Electrochemical Energy Storage Devices. Small 2023, 2304543, 1–17. doi:10.1002/smll.202304543.
- Lökçü, E.; Toparli, Ç; Anik, M. Electrochemical Performance of (MgCoNiZn)1-xLixO High-entropy Oxides in Lithium-ion Batteries. ACS Appl. Mater. Interfaces 2020, 12, 23860–23866. doi:10.1021/acsami.0c03562.
- Han, X.; Li, N.; Xiong, P.; Jung, M.G.; Kang, Y.; Dou, Q.; Liu, Q.; Lee, J.Y.; Park, H.S. Electronically Coupled Layered Double Hydroxide/MXene Quantum dot Metallic Hybrids for High-performance Flexible Zinc–air Batteries. InfoMat 2021, 3, 1134–1144. doi:10.1002/inf2.12226.
- Huang, P.; Ying, H.; Zhang, S.; Zhang, Z.; Han, W.Q. In Situ Fabrication of MXene/CuS Hybrids with Interfacial Covalent Bonding via Lewis Acidic Etching Route for Efficient Sodium Storage. J. Mater. Chem. A 2022, 10, 22135–22144. doi:10.1039/D2TA06695E.
- Liu, H.J.; Dong, B. Recent Advances and Prospects of MXene-based Materials for Electrocatalysis and Energy Storage. Mater. Today Phys. 2021, 20, 100469. doi:10.1016/j.mtphys.2021.100469.
- Worku, A.K.; Ayele, D.W.; Habtu, N.G.; Admasu, B.T.; Alemayehu, G.; Taye, B.Z.; Yemata, T.A. Energy Storage Technologies; Recent Advances, Challenges, and Prospectives. In Planning of Hybrid Renewable Energy Systems, Electric Vehicles and Microgrid: Modeling, Control and Optimization; Bohre, A.K., Chaturvedi, P., Kolhe, M.L., Singh, S.N., Eds., Springer Nature Singapore: Singapore, 2022; pp. 125–150. doi:10.1007/978-981-19-0979-5_7.
- Medvedeva, A.; Makhonina, E.; Pechen, L.; Politov, Y.; Rumyantsev, A.; Koshtyal, Y.; Goloveshkin, A.; Maslakov, K.; Eremenko, I. Effect of Al and Fe Doping on the Electrochemical Behavior of Li1.2Ni0.133Mn0.534Co0.133O2 Li-Rich Cathode Material. Materials (Basel) 2022, 15, 8225. doi:10.3390/ma15228225.
- Salas, A.; Pazniak, H.; Gonzalez-Julian, J.; Bianco, S.; Amici, J.; Ouisse, T.; Roppolo, I.; Cocuzza, M. Development of Polymeric/MXenes Composites Towards 3D Printable Electronics. Compos. Part B Eng. 2023, 263, 110854. doi:10.1016/j.compositesb.2023.110854.
- Ponnalagar, D.; Hang, D.R.; Islam, S.E.; Te Liang, C.; Chou, M.M.C. Recent Progress in Two-dimensional Nb2C MXene for Applications in Energy Storage and Conversion. Mater. Des. 2023, 231, 112046. doi:10.1016/j.matdes.2023.112046.
- Jiao, Z.; Liu, M.; Wang, Z.; Ning, X.; Yang, Y.; Li, S.; Liu, T. Ti3C2OH MXene Supported NiPtP Nanoparticles with Low Noble Metal Content as Hydrazine Dehydrogenation Catalysts. J. Taiwan Inst. Chem. Eng. 2023, 146, 104794. doi:10.1016/j.jtice.2023.104794.
- Xu, D.; Kang, Z.; Zhao, H.; Ji, Y.; Yao, W.; Ye, D.; Zhang, J. Coupling Heterostructured CoP-NiCoP Nanopin Arrays with MXene (Ti3C2Tx) as an Efficient Bifunctional Electrocatalyst for Overall Water Splitting. J. Colloid Interface Sci. 2023, 639, 223–232. doi:10.1016/j.jcis.2023.02.052.
- Zhang, Q.; Guo, D.; ling Yang, Y.; ming Hong, J. Enhanced Electrocatalytic Performance of 2D/2D MXene/Reduced Graphene Oxide(RGO) Heterostructures for Paracetamol (APAP) Degradation: The Critical Contributions of Charge Transfer and Ti-OH Edges for Active Species Generation. J. Environ. Chem. Eng. 2023, 11, 110193. doi:10.1016/j.jece.2023.110193.
- Peng, X.; Li, T.; Zhong, L.; Lu, J. Flexible Metal–air Batteries: An Overview. SmartMat 2021, 2, 123–126. doi:10.1002/smm2.1044.
- Sharma, K.; Hasija, V.; Patial, S.; Singh, P.; Nguyen, V.H.; Nadda, A.K.; Thakur, S.; Nguyen-Tri, P.; Nguyen, C.C.; Kim, S.Y.; Van Le, Q.; Raizada, P. Recent Progress on MXenes and MOFs Hybrids: Structure, Synthetic Strategies and Catalytic Water Splitting. Int. J. Hydrogen Energy 2023, 48, 6560–6574. doi:10.1016/j.ijhydene.2022.01.004.
- Ferrara, C.; Gentile, A.; Marchionna, S.; Ruffo, R. Ti3C2Tx MXene Compounds for Electrochemical Energy Storage. Curr. Opin. Electrochem. 2021, 29, 100764. doi:10.1016/J.COELEC.2021.100764.
- Kailasa, S.K.; Joshi, D.J.; Koduru, J.R.; Malek, N.I. Review on MXenes-based Nanomaterials for Sustainable Opportunities in Energy Storage, Sensing and Electrocatalytic Reactions. J. Mol. Liq. 2021, 342, 117524. doi:10.1016/j.molliq.2021.117524.
- Zhang, Y.; Ma, C.; He, W.; Zhang, C.; Zhou, L.; Wang, G.; Wei, W. MXene and MXene-based Materials for Lithium-sulfur Batteries. Prog. Nat. Sci. Mater. Int. 2021, 31, 501–513. doi:10.1016/j.pnsc.2021.07.003.
- Chen, J.; Long, Q.; Xiao, K.; Ouyang, T.; Li, N.; Ye, S.; Liu, Z.Q. Vertically-interlaced NiFeP/MXene Electrocatalyst with Tunable Electronic Structure for High-efficiency Oxygen Evolution Reaction. Sci. Bull. 2021, 66, 1063–1072. doi:10.1016/j.scib.2021.02.033.
- Meng, W.; Liu, X.; Song, H.; Xie, Y.; Shi, X.; Dargusch, M.; Chen, Z.G.; Tang, Z.; Lu, S. Advances and Challenges in 2D MXenes: From Structures to Energy Storage and Conversions. Nano. Today. 2021, 40, 101273. doi:10.1016/j.nantod.2021.101273.
- Xu, H.; Zhu, W.; Sun, F.; Qi, H.; Zou, J.; Laine, R.; Ding, W. Turning Trash into Treasure: MXene with Intrinsic LiF Solid Electrolyte Interfaces Performs Better and Better During Battery Cycling. Adv. Mater. Technol. 2021, 6. doi:10.1002/ADMT.202000882.
- Gogotsi, Y.; Anasori, B. The Rise of MXenes. ACS Nano 2019, 13, 8491–8494. doi:10.1021/acsnano.9b06394.
- Eames, C.; Islam, M.S. Ion Intercalation into Two-dimensional Transition-metal Carbides: Global Screening for New High-capacity Battery Materials. J. Am. Chem. Soc. 2014, 136, 16270–16276. doi:10.1021/ja508154e.
- Lu, Y.; Wang, L.; Cheng, J.; Goodenough, J.B. Prussian Blue: A New Framework of Electrode Materials for Sodium Batteries. Chem. Commun. 2012, 48, 6544–6546. doi:10.1039/c2cc31777j.
- Bao, Z.; Lu, C.; Cao, X.; Zhang, P.; Yang, L.; Zhang, H.; Sha, D.; He, W.; Zhang, W.; Pan, L.; Sun, Z. Role of MXene Surface Terminations in Electrochemical Energy Storage: A Review. Chinese Chem. Lett. 2021, 32, 2648–2658. doi:10.1016/j.cclet.2021.02.012.
- Naguib, M.; Mochalin, V.N.; Barsoum, M.W.; Gogotsi, Y. 25th Anniversary Article: MXenes: A New Family of Two-dimensional Materials. Adv. Mater. 2014, 26, 992–1005. doi:10.1002/adma.201304138.
- Wei, C.; Tao, Y.; An, Y.; Tian, Y.; Zhang, Y.; Feng, J.; Qian, Y. Recent Advances of Emerging 2D MXene for Stable and Dendrite-free Metal Anodes. Adv. Funct. Mater. 2020, 30. doi:10.1002/ADFM.202004613.
- Lu, Z.; Neupane, G.P.; Jia, G.; Zhao, H.; Qi, D.; Du, Y.; Lu, Y.; Yin, Z. 2D Materials Based on Main Group Element Compounds: Phases, Synthesis, Characterization, and Applications. Adv. Funct. Mater. 2020, 30. doi:10.1002/ADFM.202001127.
- Moreau, P.; Guyomard, D.; Gaubicher, J.; Boucher, F. Structure and Stability of Sodium Intercalated Phases in Olivine FePO 4. Chem. Mater. 2010, 22, 4126–4128. doi:10.1021/cm101377h.
- Li, K.; Liang, M.; Wang, H.; Wang, X.; Huang, Y.; Coelho, J.; Pinilla, S.; Zhang, Y.; Qi, F.; Nicolosi, V.; Xu, Y. 3D MXene Architectures for Efficient Energy Storage and Conversion. Adv. Funct. Mater. 2020, 30. doi:10.1002/ADFM.202000842.
- Chen, C.; Xie, X.; Anasori, B.; Sarycheva, A.; Makaryan, T.; Zhao, M.; Urbankowski, P.; Miao, L.; Jiang, J.; Gogotsi, Y. MoS2-on-MXene Heterostructures as Highly Reversible Anode Materials for Lithium-ion Batteries. Angew. Chemie - Int. Ed. 2018, 57, 1846–1850. doi:10.1002/anie.201710616.
- Lim, K.R.G.; Handoko, A.D.; Nemani, S.K.; Wyatt, B.; Jiang, H.Y.; Tang, J.; Anasori, B.; Seh, Z.W. Rational Design of two-Dimensional Transition Metal Carbide/Nitride (MXene) Hybrids and Nanocomposites for Catalytic Energy Storage and Conversion. ACS Nano 2020, 14, 10834–10864. doi:10.1021/acsnano.0c05482.
- Tang, K.; Fu, L.; White, R.J.; Yu, L.; Titirici, M.M.; Antonietti, M.; Maier, J. Hollow Carbon Nanospheres with Superior Rate Capability for Sodium-based Batteries. Adv. Energy Mater. 2012, 2, 873–877. doi:10.1002/aenm.201100691.
- Huang, Z.; Farahmandjou, M.; Marlton, F.; Guo, X.; Gao, H.; Sun, B.; Wang, G. Surface and Structure Engineering of MXenes for Rechargeable Batteries Beyond Lithium. J. Mater. 2024, 10, 253–268. doi:10.1016/J.JMAT.2023.10.001.
- Wang, X.; Shen, X.; Gao, Y.; Wang, Z.; Yu, R.; Chen, L. Atomic-scale Recognition of Surface Structure and Intercalation Mechanism of Ti3C2X. J. Am. Chem. Soc. 2015, 137, 2715–2721. doi:10.1021/ja512820k.
- Zhou, J.; Zha, X.; Zhou, X.; Chen, F.; Gao, G.; Wang, S.; Shen, C.; Chen, T.; Zhi, C.; Eklund, P.; Du, S.; Xue, J.; Shi, W.; Chai, Z.; Huang, Q. Synthesis and Electrochemical Properties of Two-dimensional Hafnium Carbide. ACS Nano 2017, 11, 3841–3850. doi:10.1021/acsnano.7b00030.
- Gautam, R.; Marriwala, N.; Devi, R. A Review: Study of Mxene and Graphene Together. Meas. Sensors 2023, 25, 100592. doi:10.1016/j.measen.2022.100592.
- Lukatskaya, M.R.; Mashtalir, O.; Ren, C.E.; Dall’Agnese, Y.; Rozier, P.; Taberna, P.L.; Naguib, M.; Simon, P.; Barsoum, M.W.; Gogotsi, Y. Cation Intercalation and High Volumetric Capacitance of Two-dimensional Titanium Carbide. Science 2013, 341, 1502–1505. doi:10.1126/science.1241488.
- Li, Z.; Wu, Y. 2D Early Transition Metal Carbides (MXenes) for Catalysis. Small 2019, 15. doi:10.1002/SMLL.201804736.
- Anasori, B.; Gogotsi, Y. Introduction to 2D Transition Metal Carbides and Nitrides (MXenes), 2D met. Carbides Nitrides Struct. Prop. Appl. 2019, 3–12. doi:10.1007/978-3-030-19026-2_1.
- Xiu, L.; Wang, Z.; Yu, M.; Wu, X.; Qiu, J. Aggregation-resistant 3D MXene-based Architecture as Efficient Bifunctional Electrocatalyst for Overall Water Splitting. ACS Nano 2018, 12, 8017–8028. doi:10.1021/acsnano.8b02849.
- Qian, J.; Xiong, Y.; Cao, Y.; Ai, X.; Yang, H. Synergistic Na-storage Reactions in Sn4P3 as a High-capacity, Cycle-stable Anode of Na-ion Batteries. Nano Lett. 2014, 14, 1865–1869. doi:10.1021/nl404637q.
- Hong, W.; Wyatt, B.C.; Nemani, S.K.; Anasori, B. Double Transition-Metal MXenes: Atomistic Design of Two-dimensional Carbides and Nitrides. MRS Bull. 2020, 45, 850–861. doi:10.1557/mrs.2020.251.
- Zhu, Y.; Han, X.; Xu, Y.; Liu, Y.; Zheng, S.; Xu, K.; Hu, L.; Wang, C. Electrospun Sb/C Fibers for a Stable and Fast Sodium-ion Battery Anode. ACS Nano 2013, 7, 6378–6386. doi:10.1021/nn4025674.
- Yang, J.; Wang, T.; Guo, X.; Sheng, X.; Li, J.; Wang, C.; Wang, G. Flexible Sodium-ion Capacitors Boosted by High Electrochemically-Reactive and Structurally-Stable Sb2S3 Nanowire/Ti3C2Tx MXene Film Anodes. Nano Res. 2023, 16, 5592–5600. doi:10.1007/s12274-021-3933-7.
- Bao, W.; Shuck, C.E.; Zhang, W.; Guo, X.; Gogotsi, Y.; Wang, G. Boosting Performance of Na-S Batteries Using Sulfur-doped Ti3C2Tx MXene Nanosheets with a Strong Affinity to Sodium Polysulfides. ACS Nano 2019, 13, 11500–11509. doi:10.1021/acsnano.9b04977.
- Verger, L.; Natu, V.; Carey, M.; Barsoum, M.W. MXenes: An Introduction of their Synthesis, Select Properties, and Applications. Trends Chem. 2019, 1, 656–669. doi:10.1016/j.trechm.2019.04.006.
- Zhang, L.; Wang, Z.; Chen, W.; Yuan, R.; Zhan, K.; Zhu, M.; Yang, J.; Zhao, B. Fe3O4nanoplates Anchored on Ti3C2T: XMXene with Enhanced Pseudocapacitive and Electrocatalytic Properties. Nanoscale. 2021, 13, 15343–15351. doi:10.1039/D1NR04383H.
- Naguib, M.; Kurtoglu, M.; Presser, V.; Lu, J.; Niu, J.; Heon, M.; Hultman, L.; Gogotsi, Y.; Barsoum, M.W. Two-dimensional Nanocrystals Produced by Exfoliation of Ti 3AlC 2. Adv. Mater. 2011, 23, 4248–4253. doi:10.1002/adma.201102306.
- Darwiche, A.; Marino, C.; Sougrati, M.T.; Fraisse, B.; Stievano, L.; Monconduit, L. Better Cycling Performances of Bulk sb in na-ion Batteries Compared to li-ion Systems: An Unexpected Electrochemical Mechanism. J. Am. Chem. Soc. 2012, 134, 20805–20811. doi:10.1021/ja310347x.
- Li, Y.; Yang, S.; Liang, Z.; Xue, Y.; Cui, H.; Tian, J. 1T-MoS2 Nanopatch/Ti3C2 MXene/TiO2 Nanosheet Hybrids for Efficient Photocatalytic Hydrogen Evolution. Mater. Chem. Front. 2019, 3, 2673–2680. doi:10.1039/C9QM00608G.
- Huang, X.; Wu, P. A Small Amount of Delaminated Ti3C2 Flakes to Greatly Enhance the Thermal Conductivity of Boron Nitride Papers by Assembling a Well-designed Interface. Mater. Chem. Front. 2020, 4, 292–301. doi:10.1039/C9QM00616H.
- Tang, X.; Liu, H.; Guo, X.; Wang, S.; Wu, W.; Mondal, A.K.; Wang, C.; Wang, G. A Novel Lithium-ion Hybrid Capacitor Based on an Aerogel-like MXene Wrapped Fe2O3 Nanosphere Anode and a 3D Nitrogen Sulphur Dual-Doped Porous Carbon Cathode. Mater. Chem. Front. 2018, 2, 1811–1821. doi:10.1039/C8QM00232K.
- Byeon, A.; Hatter, C.B.; Park, J.H.; Ahn, C.W.; Gogotsi, Y.; Lee, J.W. Molybdenum Oxide/Carbon Composites Derived from the CO2 Oxidation of Mo2CTx (MXene) for Lithium ion Battery Anodes. Electrochim. Acta 2017, 258, 979–987. doi:10.1016/j.electacta.2017.11.149.
- Feng, W.; Luo, H.; Zeng, S.; Chen, C.; Deng, L.; Tan, Y.; Zhou, X.; Peng, S.; Zhang, H. Ni-modified Ti3C2 MXene with Enhanced Microwave Absorbing Ability. Mater. Chem. Front. 2018, 2, 2320–2326. doi:10.1039/C8QM00436F.
- Li, X.; Lao, J.; Li, G.; Song, J.; Luo, J. A bio-Inspired Transpiration ion Pump Based on MXene. Mater. Chem. Front. 2020, 4, 3361–3367. doi:10.1039/D0QM00569J.
- Yu, H.; Wang, Y.; Jing, Y.; Ma, J.; Du, C.F.; Yan, Q. Surface Modified MXene-Based Nanocomposites for Electrochemical Energy Conversion and Storage. Small 2019, 15. doi:10.1002/SMLL.201901503.
- Liu, A.; Liang, X.; Ren, X.; Guan, W.; Gao, M.; Yang, Y.; Yang, Q.; Gao, L.; Li, Y.; Ma, T. Recent Progress in MXene-Based Materials: Potential High-performance Electrocatalysts. Adv. Funct. Mater. 2020, 30. doi:10.1002/ADFM.202003437.
- Yoon, Y.; Tiwari, A.P.; Lee, M.; Choi, M.; Song, W.; Im, J.; Zyung, T.; Jung, H.K.; Lee, S.S.; Jeon, S.; An, K.S. Enhanced Electrocatalytic Activity by Chemical Nitridation of Two-dimensional Titanium Carbide MXene for Hydrogen Evolution. J. Mater. Chem. A 2018, 6, 20869–20877. doi:10.1039/C8TA08197B.
- An, Y.; Tian, Y.; Man, Q.; Shen, H.; Liu, C.; Xiong, S.; Feng, J. Fluorine- and Acid-free Strategy Toward Scalable Fabrication of two-dimensional MXenes for Sodium-ion Batteries. Nano Lett. 2023, 23, 5217–5226. doi:10.1021/acs.nanolett.3c01201.
- Zhao, D.; Clites, M.; Ying, G.; Kota, S.; Wang, J.; Natu, V.; Wang, X.; Pomerantseva, E.; Cao, M.; Barsoum, M.W. Alkali-induced Crumpling of Ti3C2T: X (MXene) to Form 3D Porous Networks for Sodium ion Storage. Chem. Commun. 2018, 54, 4533–4536. doi:10.1039/C8CC00649K.
- Huang, H.; Cui, J.; Liu, G.; Bi, R.; Zhang, L. Carbon-Coated MoSe 2 /MXene Hybrid Nanosheets for Superior Potassium Storage. ACS Nano 2019, 13, 3448–3456. doi:10.1021/acsnano.8b09548.
- Zhao, S.; Liu, Z.; Xie, G.; Guo, X.; Guo, Z.; Song, F.; Li, G.; Chen, C.; Xie, X.; Zhang, N.; Sun, B.; Guo, S.; Wang, G. Achieving High-performance 3D K+-Pre-intercalated Ti3C2Tx MXene for Potassium-Ion Hybrid Capacitors via Regulating Electrolyte Solvation Structure. Angew. Chemie - Int. Ed. 2021, 60, 26246–26253. doi:10.1002/anie.202112090.
- Palomares, V.; Casas-Cabanas, M.; Castillo-Martínez, E.; Han, M.H.; Rojo, T. Update on Na-Based Battery Materials. A Growing Research Path. Energy Environ. Sci. 2013, 6, 2312–2337. doi:10.1039/c3ee41031e.
- Mishra, I.K.; Zhou, H.; Sun, J.; Dahal, K.; Ren, Z.; He, R.; Chen, S.; Ren, Z. Highly Efficient Hydrogen Evolution by Self-standing Nickel Phosphide-based Hybrid Nanosheet Arrays Electrocatalyst. Mater. Today Phys. 2018, 4, 1–6. doi:10.1016/j.mtphys.2018.01.001.
- Dai, C.; Chen, Y.; Jing, X.; Xiang, L.; Yang, D.; Lin, H.; Liu, Z.; Han, X.; Wu, R. Two-dimensional Tantalum Carbide (MXenes) Composite Nanosheets for Multiple Imaging-Guided Photothermal Tumor Ablation. ACS Nano 2017, 11, 12696–12712. doi:10.1021/acsnano.7b07241.
- Djire, A.; Wang, X.; Xiao, C.; Nwamba, O.C.; Mirkin, M.V.; Neale, N.R. Basal Plane Hydrogen Evolution Activity from Mixed Metal Nitride MXenes Measured by Scanning Electrochemical Microscopy. Adv. Funct. Mater. 2020, 30. doi:10.1002/ADFM.202001136.
- Zhu, J.; Wang, M.; Lyu, M.; Jiao, Y.; Du, A.; Luo, B.; Gentle, I.; Wang, L. Two-dimensional Titanium Carbonitride Mxene for High-Performance Sodium Ion Batteries. ACS Appl. Nano Mater. 2018, 1, 6854–6863. doi:10.1021/acsanm.8b01330.
- Luo, J.; Zhang, W.; Yuan, H.; Jin, C.; Zhang, L.; Huang, H.; Liang, C.; Xia, Y.; Zhang, J.; Gan, Y.; Tao, X. Pillared Structure Design of MXene with Ultralarge Interlayer Spacing for High-performance Lithium-ion Capacitors. ACS Nano 2017, 11, 2459–2469. doi:10.1021/acsnano.6b07668.
- Luo, J.; Lu, X.; Matios, E.; Wang, C.; Wang, H.; Zhang, Y.; Hu, X.; Li, W. Tunable MXene-Derived 1D/2D Hybrid Nanoarchitectures as a Stable Matrix for Dendrite-Free and Ultrahigh Capacity Sodium Metal Anode. Nano Lett. 2020, 20, 7700–7708. doi:10.1021/acs.nanolett.0c03215.
- Guo, X.; Zhang, W.; Zhang, J.; Zhou, D.; Tang, X.; Xu, X.; Li, B.; Liu, H.; Wang, G. Boosting Sodium Storage in Two-dimensional Phosphorene/Ti3C2Tx MXene Nanoarchitectures with Stable Fluorinated Interphase. ACS Nano 2020, 14, 3651–3659. doi:10.1021/acsnano.0c00177.
- Li, J.; Guo, C.; Li, C.M. Recent Advances of Two-dimensional (2 D) MXenes and Phosphorene for High-performance Rechargeable Batteries. ChemSusChem. 2020, 13, 1047–1070. doi:10.1002/cssc.202000061.
- Xie, Y.; Dall’Agnese, Y.; Naguib, M.; Gogotsi, Y.; Barsoum, M.W.; Zhuang, H.L.; Kent, P.R.C. Prediction and Characterization of Mxene Nanosheet Anodes for Non-lithium-ion Batteries. ACS Nano 2014, 8, 9606–9615. doi:10.1021/nn503921j.
- Halim, J.; Kota, S.; Lukatskaya, M.R.; Naguib, M.; Zhao, M.Q.; Moon, E.J.; Pitock, J.; Nanda, J.; May, S.J.; Gogotsi, Y.; Barsoum, M.W. Synthesis and Characterization of 2D Molybdenum Carbide (MXene). Adv. Funct. Mater. 2016, 26, 3118–3127. doi:10.1002/adfm.201505328.
- Alhabeb, M.; Maleski, K.; Mathis, T.S.; Sarycheva, A.; Hatter, C.B.; Uzun, S.; Levitt, A.; Gogotsi, Y. Selective Etching of Silicon from Ti3SiC2 (MAX) To Obtain 2D Titanium Carbide (MXene). Angew. Chemie - Int. Ed. 2018, 57, 5444–5448. doi:10.1002/anie.201802232.
- Zhang, J.; Zhao, D.; Xiao, D.; Ma, C.; Du, H.; Li, X.; Zhang, L.; Huang, J.; Huang, H.; Jia, C.L.; Tománek, D.; Niu, C. Assembly of Ring-shaped Phosphorus within Carbon Nanotube Nanoreactors. Angew. Chemie - Int. Ed. 2017, 56, 1850–1854. doi:10.1002/anie.201611740.
- Fan, K.; Ying, Y.; Li, X.; Luo, X.; Huang, H. Theoretical Investigation of V3C2 MXene as Prospective High-capacity Anode Material for Metal-ion (Li, Na, K, and Ca) Batteries. J. Phys. Chem. C 2019, 123, 18207–18214. doi:10.1021/acs.jpcc.9b03963.
- Fang, Y.; Lian, R.; Li, H.; Zhang, Y.; Gong, Z.; Zhu, K.; Ye, K.; Yan, J.; Wang, G.; Gao, Y.; Wei, Y.; Cao, D. Induction of Planar Sodium Growth on MXene (Ti3C2Tx)-Modified Carbon Cloth Hosts for Flexible Sodium Metal Anodes. ACS Nano 2020, 14, 8744–8753. doi:10.1021/acsnano.0c03259.
- Lei, Y.J.; Yan, Z.C.; Lai, W.H.; Chou, S.L.; Wang, Y.X.; Liu, H.K.; Dou, S.X. Tailoring MXene-based Materials for Sodium-Ion Storage: Synthesis, Mechanisms, and Applications. Electrochem. Energy Rev. 2020, 3, 766–792. doi:10.1007/s41918-020-00079-y.
- Xie, X.; Kretschmer, K.; Anasori, B.; Sun, B.; Wang, G.; Gogotsi, Y. Porous Ti3C2TxMXene for Ultrahigh-rate Sodium-Ion Storage with Long Cycle Life. ACS Appl. Nano Mater. 2018, 1, 505–511. doi:10.1021/acsanm.8b00045.
- Naguib, M.; Adams, R.A.; Zhao, Y.; Zemlyanov, D.; Varma, A.; Nanda, J.; Pol, V.G. Electrochemical Performance of MXenes as K-ion Battery Anodes. Chem. Commun. 2017, 53, 6883–6886. doi:10.1039/C7CC02026K.
- Bao, W.; Wang, R.; Qian, C.; Zhang, Z.; Wu, R.; Zhang, Y.; Liu, F.; Li, J.; Wang, G. Porous Heteroatom-doped Ti3C2TxMXene Microspheres Enable Strong Adsorption of Sodium Polysulfides for Long-Life Room-temperature Sodium-Sulfur Batteries. ACS Nano 2021, 15, 16207–16217. doi:10.1021/acsnano.1c05193.
- Kamysbayev, V.; Filatov, A.S.; Hu, H.; Rui, X.; Lagunas, F.; Wang, D.; Klie, R.F.; Talapin, D.V. Covalent Surface Modifications and Superconductivity of Two-dimensional Metal Carbide MXenes. Science 2020, 369 (80-.), 979–983. doi:10.1126/science.aba8311.
- Ghidiu, M.; Halim, J.; Kota, S.; Bish, D.; Gogotsi, Y.; Barsoum, M.W. Ion-Exchange and Cation Solvation Reactions in Ti3C2 MXene. Chem. Mater. 2016, 28, 3507–3514. doi:10.1021/acs.chemmater.6b01275.
- Zhao, Y.; Zhang, J.; Guo, X.; Cao, X.; Wang, S.; Liu, H.; Wang, G. Engineering Strategies and Active Site Identification of MXene-based Catalysts for Electrochemical Conversion Reactions. Chem. Soc. Rev. 2023, 52, 3215–3264. doi:10.1039/D2CS00698G.
- Djire, A.; Bos, A.; Liu, J.; Zhang, H.; Miller, E.M.; Neale, N.R. Pseudocapacitive Storage in Nanolayered Ti2NTx MXene Using Mg-Ion Electrolyte. ACS Appl. Nano Mater. 2019, 2, 2785–2795. doi:10.1021/acsanm.9b00289.
- Zhang, L.; Yin, L.; Zhao, R.; Di, H.; Wang, C.; Hui, X.; Zhao, D.; Wang, R. Encapsulating Ultrafine Sb Nanoparticles in Na+ Pre-intercalated 3d Porous Ti3C2TxMXene Nanostructures for Enhanced Potassium Storage Performance. ACS Nano 2020, 14, 13938–13951. doi:10.1021/acsnano.0c06360.
- Djire, A.; Zhang, H.; Liu, J.; Miller, E.M.; Neale, N.R. Electrocatalytic and Optoelectronic Characteristics of the Two-dimensional Titanium Nitride Ti 4 N 3 T x MXene. ACS Appl. Mater. Interfaces 2019, 11, 11812–11823. doi:10.1021/acsami.9b01150.
- Zhao, M.Q.; Sedran, M.; Ling, Z.; Lukatskaya, M.R.; Mashtalir, O.; Ghidiu, M.; Dyatkin, B.; Tallman, D.J.; Djenizian, T.; Barsoum, M.W.; Gogotsi, Y. Synthesis of Carbon/Sulfur Nanolaminates by Electrochemical Extraction of Titanium from Ti2SC. Angew. Chemie - Int. Ed. 2015, 54, 4810–4814. doi:10.1002/anie.201500110.
- Lukatskaya, M.R.; Halim, J.; Dyatkin, B.; Naguib, M.; Buranova, Y.S.; Barsoum, M.W.; Gogotsi, Y. Room-temperature Carbide-derived Carbon Synthesis by Electrochemical Etching of MAX Phases. Angew. Chemie - Int. Ed. 2014, 53, 4877–4880. doi:10.1002/anie.201402513.
- Murthy, A.P.; Govindarajan, D.; Theerthagiri, J.; Madhavan, J.; Parasuraman, K. Metal-doped Molybdenum Nitride Films for Enhanced Hydrogen Evolution in Near-neutral Strongly Buffered Aerobic Media. Electrochim. Acta 2018, 283, 1525–1533. doi:10.1016/j.electacta.2018.07.094.
- Li, T.; Yao, L.; Liu, Q.; Gu, J.; Luo, R.; Li, J.; Yan, X.; Wang, W.; Liu, P.; Chen, B.; Zhang, W.; Abbas, W.; Naz, R.; Zhang, D. Fluorine-free Synthesis of High-purity Ti3C2Tx (T = OH, O) via Alkali Treatment. Angew. Chemie - Int. Ed. 2018, 57, 6115–6119. doi:10.1002/anie.201800887.
- Ma, P.; Fang, D.; Liu, Y.; Shang, Y.; Shi, Y.; Yang, H.Y. MXene-based Materials for Electrochemical Sodium-Ion Storage. Adv. Sci. 2021, 8, 1–25. doi:10.1002/advs.202003185.
- Sun, W.; Shah, S.A.; Chen, Y.; Tan, Z.; Gao, H.; Habib, T.; Radovic, M.; Green, M.J. Electrochemical Etching of Ti2AlC to Ti2CT:X (MXene) in Low-concentration Hydrochloric Acid Solution. J. Mater. Chem. A 2017, 5, 21663–21668. doi:10.1039/C7TA05574A.
- Wei, Y.; Soomro, R.A.; Xie, X.; Xu, B. Design of Efficient Electrocatalysts for Hydrogen Evolution Reaction Based on 2D MXenes. J. Energy Chem. 2021, 55, 244–255. doi:10.1016/j.jechem.2020.06.069.
- Li, M.; Lu, J.; Luo, K.; Li, Y.; Chang, K.; Chen, K.; Zhou, J.; Rosen, J.; Hultman, L.; Eklund, P.; Persson, POÅ; Du, S.; Chai, Z.; Huang, Z.; Huang, Q. Element Replacement Approach by Reaction with Lewis Acidic Molten Salts to Synthesize Nanolaminated MAX Phases and MXenes. J. Am. Chem. Soc. 2019, 141, 4730–4737. doi:10.1021/jacs.9b00574.
- Yang, S.; Zhang, P.; Wang, F.; Ricciardulli, A.G.; Lohe, M.R.; Blom, P.W.M.; Feng, X. Fluoride-free Synthesis of Two-dimensional Titanium Carbide (MXene) Using A Binary Aqueous System. Angew. Chemie - Int. Ed. 2018, 57, 15491–15495. doi:10.1002/anie.201809662.
- Peng, C.; Wei, P.; Chen, X.; Zhang, Y.; Zhu, F.; Cao, Y.; Wang, H.; Yu, H.; Peng, F. A Hydrothermal Etching Route to Synthesis of 2D MXene (Ti3C2, Nb2C): Enhanced Exfoliation and Improved Adsorption Performance. Ceram. Int. 2018, 44, 18886–18893. doi:10.1016/j.ceramint.2018.07.124.
- Gao, G.; O’Mullane, A.P.; Du, A. 2D MXenes: A New Family of Promising Catalysts for the Hydrogen Evolution Reaction. ACS Catal. 2017, 7, 494–500. doi:10.1021/acscatal.6b02754.
- Liu, J.; Liu, Y.; Xu, D.; Zhu, Y.; Peng, W.; Li, Y.; Zhang, F.; Fan, X. Hierarchical “Nanoroll” Like MoS2/Ti3C2Tx Hybrid with High Electrocatalytic Hydrogen Evolution Activity. Appl. Catal. B Environ. 2019, 241, 89–94. doi:10.1016/j.apcatb.2018.08.083.
- Wang, Y.; Mao, J.; Meng, X.; Yu, L.; Deng, D.; Bao, X. Catalysis with Two-dimensional Materials Confining Single Atoms: Concept, Design, and Applications. Chem. Rev. 2019, 119, 1806–1854. doi:10.1021/acs.chemrev.8b00501.
- Ortiz, G.F.; Hanzu, I.; Knauth, P.; Lavela, P.; Tirado, J.L.; Djenizian, T. TiO2 Nanotubes Manufactured by Anodization of Ti Thin Films for on-Chip Li-ion 2D Microbatteries. Electrochim. Acta 2009, 54, 4262–4268. doi:10.1016/j.electacta.2009.02.085.
- Zhou, J.; Lin, S.; Huang, Y.; Tong, P.; Zhao, B.; Zhu, X.; Sun, Y. Synthesis and Lithium ion Storage Performance of Two-dimensional V4C3 MXene. Chem. Eng. J. 2019, 373, 203–212. doi:10.1016/j.cej.2019.05.037.
- Ramalingam, V.; Varadhan, P.; Fu, H.C.; Kim, H.; Zhang, D.; Chen, S.; Song, L.; Ma, D.; Wang, Y.; Alshareef, H.N.; He, J.H. Heteroatom-Mediated Interactions between Ruthenium Single Atoms and an MXene Support for Efficient Hydrogen Evolution. Adv. Mater. 2019, 31. doi:10.1002/adma.201903841.
- Butt, R.; Siddique, A.H.; Bokhari, S.W.; Jiang, S.; Lei, D.; Zhou, X.; Liu, Z. Niobium Carbide/Reduced Graphene Oxide Hybrid Porous Aerogel as High Capacity and Long-life Anode Material for Li-ion Batteries. Int. J. Energy Res. 2019, 43, 4995–5003. doi:10.1002/er.4598.
- Shein, I.R.; Ivanovskii, A.L. Planar Nano-Block Structures Ti n+1Al 0.5C n and Ti n+1C n (n = 1, and 2) from MAX Phases: Structural, Electronic Properties and Relative Stability from First Principles Calculations. Superlattices Microstruct. 2012, 52, 147–157. doi:10.1016/j.spmi.2012.04.014.
- Wang, R.; Chen, C.; Zheng, Y.; Wang, H.; Liu, J.W.; Yu, S.H. Structure-property Relationship of Assembled Nanowire Materials. Mater. Chem. Front. 2020, 4, 2881–2903. doi:10.1039/D0QM00365D.
- Xia, Z.; Chen, X.; Ci, H.; Fan, Z.; Yi, Y.; Yin, W.; Wei, N.; Cai, J.; Zhang, Y.; Sun, J. Designing N-Doped Graphene/ReSe2/Ti3C2 MXene Heterostructure Frameworks as Promising Anodes for High-rate Potassium-ion Batteries. J. Energy Chem 2020, 53, 155–162. doi:10.1016/j.jechem.2020.04.071.
- Yoon, Y.; Tiwari, A.P.; Choi, M.; Novak, T.G.; Song, W.; Chang, H.; Zyung, T.; Lee, S.S.; Jeon, S.; An, K.S. Precious-metal-free Electrocatalysts for Activation of Hydrogen Evolution with Nonmetallic Electron Donor: Chemical Composition Controllable Phosphorous Doped Vanadium Carbide MXene. Adv. Funct. Mater. 2019, 29. doi:10.1002/ADFM.201903443.
- Feng, A.; Yu, Y.; Wang, Y.; Jiang, F.; Yu, Y.; Mi, L.; Song, L. Two-dimensional MXene Ti3C2 Produced by Exfoliation of Ti3AlC2. Mater. Des 2017, 114, 161–166. doi:10.1016/j.matdes.2016.10.053.
- Yuan, W.; Cheng, L.; An, Y.; Wu, H.; Yao, N.; Fan, X.; Guo, X. MXene Nanofibers as Highly Active Catalysts for Hydrogen Evolution Reaction. ACS Sustain. Chem. Eng. 2018, 6, 8976–8982. doi:10.1021/acssuschemeng.8b01348.
- Karlsson, L.H.; Birch, J.; Halim, J.; Barsoum, M.W.; Persson, P.O.Å. Atomically Resolved Structural and Chemical Investigation of Single MXene Sheets. Nano Lett. 2015, 15, 4955–4960. doi:10.1021/acs.nanolett.5b00737.
- Sambyal, P.; Iqbal, A.; Hong, J.; Kim, H.; Kim, M.K.; Hong, S.M.; Han, M.; Gogotsi, Y.; Koo, C.M. Ultralight and Mechanically Robust Ti3C2 T x Hybrid Aerogel Reinforced by Carbon Nanotubes for Electromagnetic Interference Shielding. ACS Appl. Mater. Interfaces 2019, 11, 38046–38054. doi:10.1021/acsami.9b12550.
- Zhao, J.; Wen, J.; Xiao, J.; Ma, X.; Gao, J.; Bai, L.; Gao, H.; Zhang, X.; Zhang, Z. Nb2CTx MXene: High Capacity and Ultra-long Cycle Capability for Lithium-ion Battery by Regulation of Functional Groups. J. Energy Chem. 2020, 53, 387–395. doi:10.1016/j.jechem.2020.05.037.
- Ma, Y.; Yue, Y.; Zhang, H.; Cheng, F.; Zhao, W.; Rao, J.; Luo, S.; Wang, J.; Jiang, X.; Liu, Z.; Liu, N.; Gao, Y. 3D Synergistical MXene/Reduced Graphene Oxide Aerogel for a Piezoresistive Sensor. ACS Nano 2018, 12, 3209–3216. doi:10.1021/acsnano.7b06909.
- Zhou, Z.; Pei, Z.; Wei, L.; Zhao, S.; Jian, X.; Chen, Y. Electrocatalytic Hydrogen Evolution under Neutral pH Conditions: Current Understandings, Recent Advances, and Future Prospects. Energy Environ. Sci. 2020, 13, 3185–3206. doi:10.1039/D0EE01856B.
- Zamhuri, A.; Lim, G.P.; Ma, N.L.; Tee, K.S.; Soon, C.F. MXene in the Lens of Biomedical Engineering: Synthesis, Applications and Future Outlook. Biomed. Eng. Online 2021, 20, 1–24. doi:10.1186/s12938-021-00873-9.
- Pei, Z.; Gu, J.; Wang, Y.; Tang, Z.; Liu, Z.; Huang, Y.; Huang, Y.; Zhao, J.; Chen, Z.; Zhi, C. Component Matters: Paving the Roadmap toward Enhanced Electrocatalytic Performance of Graphitic C3N4-based Catalysts via Atomic Tuning. ACS Nano 2017, 11, 6004–6014. doi:10.1021/acsnano.7b01908.
- Yang, J.; Mohmad, A.R.; Wang, Y.; Fullon, R.; Song, X.; Zhao, F.; Bozkurt, I.; Augustin, M.; Santos, E.J.G.; Shin, H.S.; Zhang, W.; Voiry, D.; Jeong, H.Y.; Chhowalla, M. Ultrahigh-current-density Niobium Disulfide Catalysts for Hydrogen Evolution. Nat. Mater. 2019, 18, 1309–1314. doi:10.1038/s41563-019-0463-8.
- Handoko, A.D.; Steinmann, S.N.; Seh, Z.W. Theory-guided Materials Design: Two-Dimensional MXenes in Electro- and Photocatalysis. Nanoscale Horiz. 2019, 4, 809–827. doi:10.1039/C9NH00100J.
- Roknuzzaman, M.; Hadi, M.A.; Abden, M.J.; Nasir, M.T.; Islam, A.K.M.A.; Ali, M.S.; Ostrikov, K.; Naqib, S.H. Physical Properties of Predicted Ti2CdN Versus Existing Ti2CDC MAX Phase: An ab Initio Study. Comput. Mater. Sci. 2016, 113, 148–153. doi:10.1016/j.commatsci.2015.11.039.
- Seh, Z.W.; Fredrickson, K.D.; Anasori, B.; Kibsgaard, J.; Strickler, A.L.; Lukatskaya, M.R.; Gogotsi, Y.; Jaramillo, T.F.; Vojvodic, A. Two-dimensional Molybdenum Carbide (MXene) as an Efficient Electrocatalyst for Hydrogen Evolution. ACS Energy Lett. 2016, 1, 589–594. doi:10.1021/acsenergylett.6b00247.
- Peng, J.; Chen, X.; Ong, W.J.; Zhao, X.; Li, N. Surface and Heterointerface Engineering of 2D MXenes and their Nanocomposites: Insights into Electro- and Photocatalysis. Chemistry 2019, 5, 18–50. doi:10.1016/j.chempr.2018.08.037.
- Anasori, B.; Xie, Y.; Beidaghi, M.; Lu, J.; Hosler, B.C.; Hultman, L.; Kent, P.R.C.; Gogotsi, Y.; Barsoum, M.W. Two-dimensional. Ordered, Double Transition Metals Carbides (MXenes). ACS Nano 2015, 9, 9507–9516. doi:10.1021/acsnano.5b03591.
- Pandey, M.; Thygesen, K.S. Two-dimensional MXenes as Catalysts for Electrochemical Hydrogen Evolution: A Computational Screening Study. J. Phys. Chem. C 2017, 121, 13593–13598. doi:10.1021/acs.jpcc.7b05270.
- Hui, X.; Ge, X.; Zhao, R.; Li, Z.; Yin, L. Interface Chemistry on MXene-based Materials for Enhanced Energy Storage and Conversion Performance. Adv. Funct. Mater. 2020, 30. doi:10.1002/ADFM.202005190.
- Jiang, Y.; Sun, T.; Xie, X.; Jiang, W.; Li, J.; Tian, B.; Su, C. Oxygen-functionalized Ultrathin Ti 3 C 2 T x MXene for Enhanced Electrocatalytic Hydrogen Evolution. ChemSusChem. 2019, 12, 1368–1373. doi:10.1002/cssc.201803032.
- Sang, X.; Xie, Y.; Lin, M.W.; Alhabeb, M.; Van Aken, K.L.; Gogotsi, Y.; Kent, P.R.C.; Xiao, K.; Unocic, R.R. Atomic Defects in Monolayer Titanium Carbide (Ti3C2Tx) MXene. ACS Nano 2016, 10, 9193–9200. doi:10.1021/acsnano.6b05240.
- Son, D.Y.; Bae, K.H.; Kim, H.S.; Park, N.G. Effects of Seed Layer on Growth of ZnO Nanorod and Performance of Perovskite Solar Cell. J. Phys. Chem. C 2015, 119, 10321–10328. doi:10.1021/acs.jpcc.5b03276.
- Wang, H.; Lin, Y.; Liu, S.; Li, J.; Bu, L.; Chen, J.; Xiao, X.; Choi, J.H.; Gao, L.; Lee, J.M. Confined Growth of Pyridinic N-Mo2C Sites on MXenes for Hydrogen Evolution. J. Mater. Chem. A 2020, 8, 7109–7116. doi:10.1039/D0TA01697G.
- Zhang, J.; Zhao, Y.; Guo, X.; Chen, C.; Dong, C.L.; Liu, R.S.; Han, C.P.; Li, Y.; Gogotsi, Y.; Wang, G. Single Platinum Atoms Immobilized on an MXene as an Efficient Catalyst for the Hydrogen Evolution Reaction. Nat. Catal. 2018, 1, 985–992. doi:10.1038/s41929-018-0195-1.
- Worku, A.K.; Ayele, D.W.; Habtu, N.G.; Ambaw, M.D. Engineering Nanostructured Ag Doped α-MnO2 Electrocatalyst for Highly Efficient Rechargeable Zinc-air Batteries. Heliyon 2022, 8, e10960. doi:10.1016/j.heliyon.2022.e10960.
- Worku, A.K. Engineering Techniques to Dendrite Free Zinc-based Rechargeable Batteries. Front. Chem. 2022, 10, 1–15. doi:10.3389/fchem.2022.1018461.
- Habtu, N.G.; Worku, A.K.; Ayele, D.W.; Teshager, M.A.; Workineh, Z.G. Facile Preparation and Electrochemical Investigations of Copper-Ion Doped α-MnO2 Nanoparticles. Lect. Notes Inst. Comput. Sci. Soc. Telecommun. Eng. LNICST 2022, 412 LNICST, 543–553. doi:10.1007/978-3-030-93712-6_36.
- Worku, A.K.; Ayele, D.W.; Habtu, N.G.; Teshager, M.A.; Workineh, Z.G. Recent Progress in MnO2-based Oxygen Electrocatalysts for Rechargeable Zinc-air Batteries. Mater. Today Sustain. 2021, 13, 100072. doi:10.1016/j.mtsust.2021.100072.
- Worku, A.K.; Ayele, D.W.; Habtu, N.G. Recent Advances and Future Perspectives in Engineering of Bifunctional Electrocatalysts for Rechargeable Zinc–air Batteries. Mater. Today Adv. 2021, 9, 100116. doi:10.1016/j.mtadv.2020.100116.
- Kajiyama, S.; Szabova, L.; Sodeyama, K.; Iinuma, H.; Morita, R.; Gotoh, K.; Tateyama, Y.; Okubo, M.; Yamada, A. Sodium-Ion Intercalation Mechanism in MXene Nanosheets. ACS Nano 2016, 10, 3334–3341. doi:10.1021/acsnano.5b06958.
- Yang, Q.; Yang, T.; Gao, W.; Qi, Y.; Guo, B.; Zhong, W.; Jiang, J.; Xu, M. An MXene-Based Aerogel with Cobalt Nanoparticles as an Efficient Sulfur Host for Room-temperature Na-S Batteries. Inorg. Chem. Front. 2020, 7, 4396–4403. doi:10.1039/D0QI00939C.
- Liu, A.; Liang, X.; Ren, X.; Guan, W.; Ma, T. Recent Progress in MXene-based Materials for Metal-sulfur and Metal-air Batteries: Potential High-Performance Electrodes. Electrochem. Energy Rev. 2022, 5, 112–144. doi:10.1007/s41918-021-00110-w.
- Aslam, M.K.; Xu, M. A Mini-review: MXene Composites for Sodium/Potassium-ion Batteries. Nanoscale. 2020, 12, 15993–16007. doi:10.1039/D0NR04111D.
- Ghidiu, M.; Naguib, M.; Shi, C.; Mashtalir, O.; Pan, L.M.; Zhang, B.; Yang, J.; Gogotsi, Y.; Billinge, S.J.L.; Barsoum, M.W. Synthesis and Characterization of Two-dimensional Nb4C3 (MXene). Chem. Commun. 2014, 50, 9517–9520. doi:10.1039/C4CC03366C.
- Li, J.; Yan, D.; Hou, S.; Li, Y.; Lu, T.; Yao, Y.; Pan, L. Improved Sodium-ion Storage Performance of Ti3C2TX MXenes by Sulfur Doping. J. Mater. Chem. A 2018, 6, 1234–1243. doi:10.1039/C7TA08261D.
- Worku, A.K.; Ayele, D.W. Advances and Challenges in the Fabrication of Porous Metal Phosphate and Phosphonate for Emerging Applications. In Metal Phosphates and Phosphonates: Fundamental to Advanced Emerging Applications; Gupta, R.K., Ed.; Springer International Publishing: Cham, 2023; pp. 393–407. doi:10.1007/978-3-031-27062-8_22.
- Ambissa Begaw, G.; Worku Ayele, D.; Ketema Worku, A.; Alemneh Wubieneh, T.; Atnafu Yemata, T.; Dagnew Ambaw, M. Recent Advances and Challenges of Cobalt-based Materials as Air Cathodes in Rechargeable Zn–Air Batteries. Results Chem. 2023, 5, 100896. doi:10.1016/J.RECHEM.2023.100896.
- Hou, T.; Jia, Z.; Wang, B.; Li, H.; Liu, X.; Chi, Q.; Wu, G. Metal-organic Framework-Derived NiSe2-CoSe2@C/Ti3C2Tx Composites as Electromagnetic Wave Absorbers. Chem. Eng. J. 2021, 422, 130079. doi:10.1016/j.cej.2021.130079.
- Alem, A.F.; Worku, A.K.; Ayele, D.W.; Wubieneh, T.A.; abebaw Teshager, A.; Tadele mihret kndie; Admasu, B.T.; Teshager, M.A.; Asege, A.A.; Ambaw, M.D.; Zeleke, M.A.; Shibesh, A.K.; Yemata, T.A. Ag Doped Co3O4 Nanoparticles for High-performance Supercapacitor Application. Heliyon 2023, 9, e13286. doi:10.1016/j.heliyon.2023.e13286.
- Alem, A.F.; Worku, A.K.; Ayele, D.W.; Habtu, N.G.; Ambaw, M.D.; Yemata, T.A. Enhancing Pseudocapacitive Properties of Cobalt Oxide Hierarchical Nanostructures via Iron Doping. Heliyon 2023, 9, e13817. doi:10.1016/j.heliyon.2023.e13817.
- Ketema Worku, A.; Worku Ayele, D. Recent Advances of Graphene-Based Materials for Emerging Technologies. Results Chem. 2023, 100971. doi:10.1016/j.rechem.2023.100971.
- Strmcnik, D.; Lopes, P.P.; Genorio, B.; Stamenkovic, V.R.; Markovic, N.M. Design Principles for Hydrogen Evolution Reaction Catalyst Materials. Nano Energy 2016, 29, 29–36. doi:10.1016/j.nanoen.2016.04.017.
- Enterría, M.; Reynaud, M.; Paredes, J.I.; Medinilla, L.; Younesi, R.; Ortiz-Vitoriano, N. Driving the Sodium-oxygen Battery Chemistry Towards the Efficient Formation of Discharge Products: The Importance of Sodium Superoxide Quantification. J. Energy Chem. 2022, 68, 709–720. doi:10.1016/j.jechem.2021.12.014.
- Wang, X.D.; Tang, M.; Yi, D.J.; Shen, Q.Y.; Ma, J.L. Cycling Na-O2 Batteries via Li2O2 Formation and Na Deposition. Chinese J. Anal. Chem. 2022, 50, 100100. doi:10.1016/j.cjac.2022.100100.
- Sadaqat, M.; Nisar, L.; Naeem Ashiq, M.; Tabassum, H.; Tianjie, Q.; Mahmood, A. Hierarchical Porous Carbon-Incorporated Metal-based Nanocomposites for Secondary Metal-ion Batteries. Met. Oxide-Carbon Hybrid Mater. Synth. Prop. Appl. 2022, 179–216. doi:10.1016/B978-0-12-822694-0.00005-3.
- Kang, Y.; Zou, D.; Zhang, J.; Liang, F.; Hayashi, K.; Wang, H.; Xue, D.; Chen, K.; Adair, K.R.; Sun, X. Dual–phase Spinel MnCo2O4 Nanocrystals with Nitrogen-doped Reduced Graphene Oxide as Potential Catalyst for Hybrid Na–Air Batteries. Electrochim. Acta 2017, 244, 222–229. doi:10.1016/j.electacta.2017.05.100.
- Khan, Z.; Vagin, M.; Crispin, X. Can Hybrid Na–Air Batteries Outperform Nonaqueous Na–O2 Batteries? Adv. Sci. 2020, 7. doi:10.1002/advs.201902866.
- Yi, X.; Liu, X.; Fang, J.; Huo, H.; Dou, R.; Wen, Z.; Zhou, W. An Atomic/Molecular-level Strategy for the Design of a Preferred Nitrogen-doped Carbon Nanotube Cathode for Li-O2 Batteries. Appl. Surf. Sci. 2023, 615, 156367. doi:10.1016/j.apsusc.2023.156367.
- Mao, P.; Arandiyan, H.; Mofarah, S.S. Energy Advances A Comprehensive Review of Cathode Materials for Na – Air Batteries. 2023. doi:10.1039/d2ya00340f.
- Elia, G.A.; Hasa, I.; Hassoun, J. Characterization of a Reversible, low-Polarization Sodium-oxygen Battery. Electrochim. Acta 2016, 191, 516–520. doi:10.1016/j.electacta.2016.01.062.
- Biswas, S.; Banerjee, P.; Raychaudhury, M.D. Correlation-driven Metal-Insulator Transition in Unconventional Magnetic Metal Superoxides. Mater. Res. Bull. 2023, 167, 112389. doi:10.1016/j.materresbull.2023.112389.
- Sun, Q.; Yang, Y.; Fu, Z.W. Electrochemical Properties of Room Temperature Sodium–air Batteries with non-Aqueous Electrolyte. Electrochem. Commun. 2012, 16, 22–25. doi:10.1016/j.elecom.2011.12.019.
- Liu, W.M.; Yin, W.W.; Ding, F.; Sang, L.; Fu, Z.W. NiCo2O4 Nanosheets Supported on Ni Foam for Rechargeable Nonaqueous Sodium-air Batteries. Electrochem. Commun. 2014, 45, 87–90. doi:10.1016/j.elecom.2014.05.021.
- Lin, X.; Sun, Q.; Kim, J.T.; Li, X.; Zhang, J.; Sun, X. Superoxide-based Na-O2 Batteries: Background, Current Status and Future Prospects. Nano Energy 2023, 112, 108466. doi:10.1016/j.nanoen.2023.108466.
- Novčić, K.A.; Dobrota, A.S.; Petković, M.; Johansson, B.; Skorodumova, N.V.; Mentus, S.V.; Pašti, I.A. Theoretical Analysis of Doped Graphene as Cathode Catalyst in Li-O2 and Na-O2 Batteries – the Impact of the Computational Scheme. Electrochim. Acta 2020, 354, 136735. doi:10.1016/j.electacta.2020.136735.