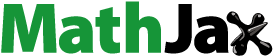
ABSTRACT
In this study, we evaluated the effect of the modification of biochar on the adsorption of Cr(VI) under weakly acidic conditions. Sesame straw biochar (BC) was modified by treatment with ferric chloride to produce iron-modified biochar (FBC) and ferric chloride and chlorapatite to produce iron-doped chlorapatite-modified biochar (FBCP). Analysis by scanning electron microscopy (SEM), Fourier-transform infrared spectroscopy (FTIR), and X-ray diffraction (XRD) showed that the adsorption mechanism of Cr(VI) by FBC and FBCP was reduction, physical adsorption, ion exchange, and functional group complexation. Experiments were performed to evaluate the kinetic, isothermal, and thermodynamic characteristics of adsorption, demonstrating that the adsorption of Cr(VI) by FBC and FBCP was a spontaneous endothermic reaction, consistent with the pseudo-second-order kinetic model and Langmuir model, and the process was dominated by monolayer chemical adsorption. The Langmuir model revealed maximum saturated adsorption capacities for Cr(VI) of 7.907 and 12.625 mg/g by FBC and FBCP, respectively, equating to 4.06 and 6.48 times the maximum saturated adsorption capacity of BC, respectively. Compared with FBC, the phosphate ions in FBCP also co-precipitated with Cr(III), further improving the removal efficiency of pollutants.
1. Introduction
The heavy metal, chromium, is a widespread environmental pollutant, which mainly comes from the discharge of industrial wastewater from electroplating, leather, wood preservatives, the dye industry, paint, papermaking, and petroleum refining (Citation1). The emissions of chromium pollutants in the chemical pollution industry in the United States are 0.16 million t/a (Citation2), the emissions of chromium pollutants in the leather industry in Italy are 12 million t/a, the emissions of chromium pollutants in India are 15 million t/a, and the emissions of chromium pollutants in China are 450,000 t/a (Citation3). Chromium predominantly exists in the form of hexavalent chromium [Cr(VI)] or trivalent chromium [Cr(III)] in water. According to the National Toxicology Project of the United States, the toxicity of Cr(VI) is 500 times that of Cr(III) (Citation4), and Cr(VI) is carcinogenic and mutagenic (Citation5, Citation6). The methods for treatment of chromium-containing wastewater include chemical reduction (Citation7), physical adsorption (Citation8), chemical precipitation (Citation9), and ion exchange (Citation10). These traditional chemical and physical methods can have disadvantages, such as high costs, unstable effects, and secondary pollution. Among them, the adsorption method has the advantages of a high removal rate, low cost, simple operation, and use of a renewable adsorbent. Consequently, the adsorption method for wastewater treatment has become a focus of research on Cr(VI) pollution control. There is a need to identify an affordable, efficient, and environmentally friendly adsorbent.
Biochar is a very useful renewable carbonaceous resource, which is usually obtained by pyrolysis of plant waste and animal waste under oxygen-limited conditions (Citation11, Citation12). Biochar is widely used in wastewater treatment, water purification, and soil improvement because of its porosity, high specific surface area, high aromaticity, and richness in active functional groups (Citation13). The main components of biochar are carbon, volatile compounds, and minerals (ash) (Citation14). Biochar comes from a wide range of sources, including plant waste and animal waste. Biocharification of these wastes can not only effectively reduce environmental pollution, but also convert these wastes into useful resources and improve their economic value. However, biochar has some shortcomings, such as loss of functional groups, uneven pore structure, and limited adsorption capacity for some heavy metals. Therefore, improving the reduction–complexation adsorption of Cr(VI) in water by biochar is key to the efficient removal of heavy metal chromium by biochar.
Biochar can exhibit good adsorption performance for hexavalent chromium under strong acidic conditions. Therefore, previous studies on the adsorption performance of hexavalent chromium in aqueous solutions have focused on strong acidic conditions (pH = 2). In this study, sesame straw, an agricultural and forestry waste, was used as raw material to prepare biochar. The composite doping modification method was used to innovatively impregnate chloroapatite and ferric chloride onto biochar. The modification process was simple, which realized the resource utilization of waste, solved the disadvantages of single application of each material, and improved the adsorption efficiency of biochar to hexavalent chromium under weak acid (pH = 5) conditions. At the same time, the adsorption mechanism of Cr (VI) by FeBCP was studied by various characterization methods. This work will provide a low-cost, efficient and environmentally friendly adsorbent for the removal of Cr(VI) contaminated water.
2. Materials and methods
2.1. Materials
The raw material of biochar used in this study was sesame straw, which was cut and collected from Taikang Farm, Zhoukou City. After washing, drying, crushing, and passing through a 20-mesh sieve, the biochar was stored for later use. The main chemical reagents used in the preparatory steps described below were anhydrous calcium chloride (CaCl2), diammonium phosphate [(NH4)2HPO4], and ferric chloride hexahydrate (FeCl3·6H2O). Deionized water was used in biochar preparation.
2.2. Preparation of Biochars
The biochar powder was placed in a ceramic crucible, compacted, sealed with aluminum foil, and placed in a muffle furnace. The temperature was increased to 550°C at 10°C/min, and oxygen-limited pyrolysis was carried out for 2 h. After natural cooling, the pyrolysis product was passed through a 100-mesh sieve to obtain sesame straw biochar (BC), which was stored in a self-sealing bag in the dark.
Impregnation of BC with ferric chloride solution was performed using a solid: liquid ratio of 1: 20. Ten grams of BC and 200 ml 0.26 M FeCl3·6H2O solution were placed in a beaker, stirred at room temperature for 4 h, filtered, washed with deionized water, dried, and passed through a 100-mesh sieve to obtain iron-modified biochar (FBC), which was then sealed and stored for later use.
Co-impregnation of BC with solutions of ferric chloride and chlorapatite (a phosphorus-containing material) was performed using a solid: liquid: liquid ratio of 1: 20: 20. Ten grams of BC was added to 100 ml 0.334 M CaCl2 solution and stirred vigorously for 30 min. Then, 100 ml of 0.2 M (NH4)2HPO4 solution was added one drop at a time, stirred for 24 h, filtered, washed with deionized water, and dried. Subsequently, 200 mL of 0.26 M FeCl3·6H2O solution was added, and the solution was stirred at room temperature for 4 h. After filtration, the substrate was washed with deionized water, dried, ground, and passed through a 100-mesh sieve to obtain iron-doped chlorapatite-modified biochar (FBCP), which was then sealed and stored for later use.
2.3. Characterization of Biochars
The surface morphology of the three biochars at different magnifications was observed by SEM (SIGMA HD, ZEISS, Germany). The infrared spectra of the three biochars, before and after reaction with Cr(Ⅵ), were assessed by FTIR spectrometry (Nicolet IS10, Thermo Scientific, USA), and the crystal structure of biochar materials was analyzed by XRD spectrometry (Smartlab, Rigaku, Japan).
2.4. Adsorption experiments
2.4.1. Adsorption kinetics experiments
Fifty milliliters of Cr(VI) contaminated solution with an initial concentration of 10 mg/L was added to each of three conical flasks. The pH of the solutions was adjusted to 5 ± 0.1, and 0.05 g of BC, FBC, or FBCP were added. The three biochar solutions were oscillated in a constant temperature oscillator at 120 r/min at 25°C. Samples were taken from each flask at 5, 15, 35, 70 min, 2, 4, 8, 12, 16, 20, 24, 36, and 48 h, and filtered through a 0.45 μm filter membrane. The concentration of residual Cr(VI) in the supernatant was measured, and the adsorption capacity of each biochar to Cr(VI) was calculated and a kinetic model was fitted to data (see below).
2.4.2. Isothermal adsorption experiment
Cr(VI) contaminated solutions with initial concentrations of 5, 10, 15, 20, 30, 40, and 50 mg/L were prepared. For each concentration and each of the three biochars, 50 mL of Cr(VI) contaminated solution was added to a conical flask. The pH of the solutions was adjusted to 5 ± 0.1, and 0.05 g of BC, FBC, or FBCP were added. All the experiments were conducted with shaking at 120 r·min−1 for 24 h at 25°C. After filtration using a 0.45 μm filter membrane, the residual Cr(VI) concentration in the supernatant was measured, the adsorption capacity of each biochar to Cr(VI) was calculated, and isothermal adsorption models were fitted to data (see below).
2.4.3. Adsorption thermodynamics experiment
Cr(VI) contaminated solutions with initial concentrations of 10, 20, 30, 40 mg/L, or 50 mg/L were prepared. For each concentration and each of the three biochars, 50 mL of Cr(VI) contaminated solution was placed in a conical flask. The pH of the solutions was adjusted to 5 ± 0.1, and 0.05 g of BC, FBC, or FBCP was added. For each concentration and each biochar, three replicate solutions were prepared and kept at 25°C, 35°C, and 45°C, respectively. Following shaking at 120 r·min−1 for 24 h, the concentration of residual Cr(VI) in the supernatant was determined by filtration with 0.45 μm filter membrane, the adsorption capacity of each biochar to Cr(VI) was calculated, and adsorption thermodynamic models were fitted to data (see below).
2.5. Data treatment
2.5.1. Adsorption capacity and removal efficiency
The adsorption capacity of the unit adsorbent and the removal efficiency of Cr(VI) in the solution are calculated as follows,
(1.1)
(1.1)
(1.2)
(1.2) where q is the adsorption capacity of the unit adsorbent (mg/g), η is the removal percentage of heavy metals, C0 is the initial concentration of heavy metals in the solution (mg/L), Ct is the residual heavy metal concentration in the solution at time t (mg/L), V is the volume of adsorption solution (L), and m is the mass of adsorbent (g).
2.5.2. Adsorption kinetics model
A pseudo-first-order model and pseudo-second-order model were fitted to data on Cr(VI) adsorption by the three biochars, using the following equations:
Pseudo-first-order Model
(1.3)
(1.3) Pseudo-secondary Model
(1.4)
(1.4) where Qt is the unit adsorbent adsorption capacity at time t (mg/g), Qe is the adsorption capacity of unit adsorbent at equilibrium (mg/g), k1 is a pseudo-first-order kinetic adsorption rate constant (min−1), and k2 is the pseudo-second-order kinetic adsorption rate constant (g/mg·min−1).
2.5.3. Adsorption isotherm model
A Langmuir model and Freundlich model were fitted to data on isothermal adsorption, using the isotherm equation as follows:
Langmuir Model
(1.5)
(1.5)
(1.6)
(1.6)
Freundlich Model
(1.7)
(1.7) where Qe is the adsorption capacity of the adsorbent at equilibrium (mg/g), Ce is the solution concentration at equilibrium (mg/L), Qmax is the theoretical monolayer saturated adsorption capacity (mg/g), KL is the Langmuir adsorption rate constant (L/mg), C0 is the initial concentration of adsorbate (mg/L), RL is the dimensionless equilibrium parameter of Langmuir model, KF is the Freundlich equilibrium constant, and n is a dimensionless parameter.
2.5.4. Thermodynamic model
The adsorption thermodynamic model shown in the formulas (1.8), (1.9), and (1.10) was fitted to data, and ΔHθ and ΔSθ were calculated as follows:
(1.8)
(1.8)
(1.9)
(1.9)
(1.10)
(1.10) where
is Gibbs free energy (J·mol−1),
is the entropy change [J·(mol·K)−1],
is enthalpy change (J·mol−1), R is a gas constant, T is the thermodynamic temperature, K, and Kc is the equilibrium constant.
3. Results and discussion
3.1. Characterization of biochars
3.1.1. Physicochemical properties
The physicochemical properties of the three biochars are shown in . According to the change of pH value of biochar, it can be seen that the modified biochar changed from alkaline (BC 10.36) to acidic (FBC 3.24, FBCP 2.78). The number ratio of different atoms can be calculated by the proportion of C, H, O and N elements. H/C can reflect the degree of aromatization of the material (Citation15), and O/C can reflect the number of oxygen-containing functional groups (Citation16). The smaller the H/C ratio is, the higher the degree of aromatization is. The H/C values of the three biochars are 0.033, 0.029, and 0.028, respectively. The degree of aromatization is: FBCP > FBC > BC, and the H/C values are all less than 0.6, indicating that the material has good stability in the environment. The results of specific surface area, pore volume and average pore size showed that the specific surface area and total porosity of modified biochar increased. This shows that iron chloride and iron-doped chloroapatite are loaded onto the surface of biochar by co-impregnation, which changes the pore structure of sesame straw biochar itself, thereby improving the adsorption performance of biochar.
Table 1. Basic physicochemical properties of BC, FBC and FBCP.
From , it can be seen that the pore size of BC is mostly in the range of 2∼5 nm, the pore size of FBC is mostly in the range of 2∼20 nm, and the pore size of FBCP is mostly in the range of 2∼40 nm, which indicates that the three biochars are a hierarchical porous structure material dominated by mesopores. It shows that when ferric chloride is added alone, it is mainly to increase the number of mesopores with smaller pore size. When chlorapatite and ferric chloride are impregnated with biochar, it is mainly to increase the number of mesopores with larger pore size. This may be due to the addition of chlorapatite and ferric chloride, which catalyzes the gasification of carbon in biochar, so that the mesopores or micropores with smaller pore size are dredged, and the larger mesopores or even macropores are formed after the pore wall is gasified and broken. The increase of mesopores is conducive to the diffusion and migration of pollutants and can improve the adsorption capacity (Citation17).
3.1.2. SEM analysis
The SEM images of BC, FBC, and FBCP are shown in , illustrating that the three biochar materials had a defined porous structure. It can be seen from (a) and (b) that the surface of BC is smoother, compared with FBC and FBCP, and the fiber and pore structures of straw were retained after high-temperature pyrolysis. Following impregnation with ferric chloride solution and iron-doped chlorapatite solution, the respective modified biochars, FBC ((c), (d)) and FBCP ((e), (f)), exhibited an interior that is mostly tubular, and the surface was uneven with many folds and obvious particle attachment. The particles attached to the surface and pores of FBC and FBCP were likely iron oxide and chlorapatite (Citation18), and because of the adhesion of these particles, the specific surface area of biochar was increased, which in turn provided more binding sites. (f) illustrates that small pores existed within the large pores, which shows that the internal pore structure of FBCP was more developed and should thus be more conducive to the adsorption of pollutants (Citation19, Citation20).
3.1.3. FTIR analysis
The FTIR spectra of BC, FBC, and FBCP, before and after adsorption of Cr(VI), are shown in . The BC, FBC, and FBCP biochars all showed stretching vibrations of the alcohol hydroxyl or phenolic hydroxyl (–OH) groups (3440 cm−1), aliphatic C–H groups (2923 cm−1), and carbonyl (C = O) groups in lactones or alkene (C = C) groups (1578 cm−1) in aromatic compounds. The peak at 3440 cm−1 was relatively wide, indicating that the three biochars contained a large number of –OH groups (Citation21, Citation22). The hydrophilicity of the carbon material was high, which was conducive to the adsorption of pollutants. In the vicinity of 1578 cm−1, the peak intensity of FBC and FBCP increased, indicating that the content of unsaturated functional groups increased after modification, the degree of aromatization increased (Citation23, Citation24), and the cation -π interaction was enhanced to provide active sites on the surface of biochar (Citation25). FBC had a weak absorption peak at 469 cm−1, which is the characteristic absorption peak of Fe–O vibrations, indicating that magnetite (Fe3O4) was loaded onto the surface of FBC (Citation26, Citation27). FBCP showed obvious P–O–P stretching vibrations at 1040 cm−1, indicating that P-containing groups were successfully introduced into FBCP. There were also characteristic peaks of diphosphate (P2O74-) around 1140 cm−1 in FBCP, which indicated that BC was modified by impregnation with iron-doped chlorapatite solution and loaded with pyrophosphate groups, iron, and oxygen-containing groups.
The absorption peaks of the main functional groups in the infrared spectra of BC showed no significant difference before and after reaction with Cr(VI), which was consistent with the finding that BC had no obvious adsorption effect on Cr(VI). Following adsorption of Cr(VI) by FBC and FBCP, the absorption peaks at 3440 cm−1 and 1578 cm−1 were significantly reduced, corresponding to the stretching vibrations of –OH and C = O/C = C groups, respectively. The absorption peak at 3440 cm−1 (–OH) was smaller, indicating that Cr(VI) and Cr(III) may have had a complexation reaction with them (Citation28). The reduction in height of the absorption peak near 1578 cm−1 indicated that the aromatic cation -π conjugated system provided -π electrons to Cr(VI) and reduced it to Cr(III), following which Cr(III) reacted with C = O/C = C under the action of pi electrons to form Cπ–Cr (Citation29). Based on the above analysis, it is concluded that the adsorption process of Cr(VI) pollutants by FBC and FBCP had redox and functional complexation effects.
3.1.4. XRD analysis
The XRD patterns of BC, FBC, and FBCP, before reaction with Cr(VI), are shown in . The three biochars retained a wide amorphous carbon peak at ∼26°, which may be caused by the microcrystalline structure and disordered graphite layer formed by the biochar during pyrolysis (Citation30). The diffraction peaks at 30.6°, 35.5°, 57.1°, and 62.6° in FBC corresponded to the (220), (311), (511), and (440) crystal planes of the Fe3O4 standard card (PDF No. 19-0629), indicating that the biochar was successfully loaded with Fe3O4 after impregnation with ferric chloride (Citation31). The diffraction peaks at 32.36° and 49.96° in FBCP corresponded to the standard card of chlorapatite [Ca5(PO4)3Cl; PDF No. 27-0074], indicating that the surface of FBCP was successfully loaded with chlorapatite (Citation32). The crystal planes of the Fe3O4 standard card (PDF No. 19-0629) were also visible in FBCP, indicating that the FBCP was successfully loaded with iron oxide as well as chlorapatite (Citation33), which was consistent with the results of FTIR analysis.
The XRD patterns of BC, FBC, and FBCP, following adsorption of Cr(VI), are shown in . After the adsorption of Cr(VI) by BC, the peak of potassium chloride (KCl) disappeared, and a peak corresponding to chromium hydroxide [Cr(OH)3] appeared. This could be caused by the reduction of Cr(VI) to Cr(III) by oxygen-containing functional groups on the surface of BC. Ion exchange between Cr(III) and potassium cations (K+) occurs, and Cr(III) can generate Cr(OH)3 under alkaline conditions, which proves the existence of ion exchange and co-precipitation mechanisms (Citation34, Citation35). After the adsorption of Cr(VI) by FBC, the peaks at (311) and (440) were more obvious, which was caused by the combination of Cr2O42- and Fe3O4 in FBC. It can be seen that reduction and complexation were the main mechanisms of Cr(VI) removal by FBC (Citation36, Citation37). Following adsorption of Cr(VI) by FBCP, the surface material composition was mainly composed of iron(II) chromite (FeCr2O4) and chromium(III) phosphate (CrPO4). Diffraction peaks were consistent with XRD standards as follows: 35.5° and 62.7° were consistent with FeCr2O4 (PDF No. 24–0512 card); 28.31° and 57.2° were consistent with CrPO4 (PDF No. 13–0425 card); 25.4° was consistent with CrPO4 (PDF No. 05–0662 card); and 41.5° was consistent with CrPO4 (PDF No. 05–0233 card). In addition, there were characteristic peaks of Cr(P2O7)3 and Cr(OH)3 at 22.8° and 37.1°. Based on the above results, it can be concluded that, in addition to reduction, FBCP also exhibited ion exchange and phosphate precipitation in the adsorption process of Cr(VI) (Citation38).
3.2. Adsorption effect
3.2.1. The influence of different pH on the adsorption effect
When the pH value is in the range of 2∼8, the removal rate of Cr(VI) is shown in . With the increase of pH value, the removal rate of Cr(VI) by three biochars showed a decreasing trend. It can be seen that the adsorption performance of biochars is strongly dependent on the pH value of Cr(VI) solution. Under the same pH conditions, the removal rate of Cr(VI) by the three biochars was: FBCP > FBC > BC, indicating that the active sites on the surface of the modified biochar increased and could be applied in a wider pH range.
With the increase of pH from 2 to 7, the removal rate of Cr(VI) by BC, FBC and FBCP decreased from 72.28%, 98.81% and 99.70% to 1.74%, 14.14% and 17.50%, respectively. This is because Cr(VI) mainly exists in the form of HCrO42- and Cr2O72- under acidic conditions, and mainly exists in the form of CrO42- under alkaline conditions (Citation39). Under acidic conditions, the functional groups on biochar can be protonated and attract negatively charged metal ions through electrostatic attraction (Citation40). At the same time, because HCrO42- has lower free adsorption energy than CrO42- and HCrO42- is easier to be reduced to low valence chromium, HCrO4- with higher charge is easier to be adsorbed. In summary, acidic conditions are more conducive to the adsorption of Cr(VI).
shows the zeta potential of BC, BCP and FeBCP at different pH values. The zero potential point (pHZPC) of BC, BCP and FeBCP were 4.78, 4.89 and 6.18, respectively. When the solution pH < pHZPC, the surface of the adsorbent is protonated and positively charged, so it has a more obvious electrostatic attraction with the Cr(VI) anion, which is also the reason for the high adsorption capacity of the adsorbent for Cr(VI) at low pH. When the solution pH > pHZPC, with the increase of pH value, the surface of the adsorbent is protonated and negatively charged, and the electrostatic repulsion reaction with the Cr(VI) anion occurs, which is not conducive to the adsorption of Cr(VI) by the adsorbent (Citation41).
3.2.2. Adsorption kinetics
The pseudo-first-order kinetic model and pseudo-second-order kinetic model were fitted to the experimental data to examine the mechanism of adsorption of Cr(VI) in water by biochar before and after modification. The fitted results are shown in and .
Table 2. Fitting Parameters of Pseudo-first-order Model, Pseudo-second-order Model, Elovich Model and Double Constant Model for Cr(VI) Adsorption on BC, FBC and FBCP.
It can be seen from that, under identical conditions, the adsorption capacity for Cr(VI) was lowest for BC and highest for FBCP. The adsorption capacity for Cr(VI) of FBC and FBCP increased from 0–48 h, while there was no significant change in the adsorption capacity of BC over 0–48 h. In the early stages of rapid adsorption, the adsorption rate was lowest for BC and highest for FBCP. In the early stage, the adsorption rate of Cr(VI) by FBC and FBCP was higher, the adsorption curve was steeper and the slope was larger, compared with the later stages of adsorption. After 480 min, the adsorption rate decreased, and after 720 min the adsorption capacity Qe for Cr(VI) did not change significantly with increasing adsorption time and the curve became shallower. The high adsorption rate in the early stage was mainly caused by the large number of adsorption sites on the surfaces of FBC and FBCP and the high concentration of Cr(VI) in the contaminated solution resulting in a high mass transfer driving force, enabling Cr(VI) to easily enter the adsorption sites on the surface of biochars. As the adsorption time increased, the concentration of Cr(VI) in the solution decreased and there were fewer available adsorption sites on the surface of the biochars, and thus the adsorption capacity reached its maximum (Citation42).
It can be seen from that the adsorption capacity for Cr(VI) of modified biochar was higher than that of unmodified biochar. The adsorption capacities of FBC and FBCP were 8.33 and 9.95 times that of the unmodified biochar (BC), respectively. The adsorption effect of FBCP on Cr(VI) was higher than that of FBC. The pseudo-second-order model parameters (R2) of BC, FBC, and FBCP all exceeded 0.9, indicating that the adsorption of Cr(VI) by biochar, before and after modification, was consistent with the pseudo-second-order model, confirming that the adsorption process of Cr(VI) in water by biochar was mainly driven by chemical adsorption (Citation43, Citation44).
3.2.3. Adsorption isotherms
Freundlich and Langmuir models were fitted to adsorption data for BC, FBC, and FBCP in Cr(VI) contaminated solution with initial concentrations of 5 mg/L, 10, 20, 30, 40 mg/L, and 50 mg/L, a pH of 5, and a temperature of 25°C. The fitted curve and parameters are shown in and , respectively.
Table 3. Fitting parameters of adsorption isotherms of Cr(VI) on BC, FBC and FBCP.
It can be seen from the data in that the Langmuir isothermal adsorption model better explained the adsorption process of Cr(VI) by the biochars, indicating that the adsorption of Cr(VI) in water by biochars was caused by monolayer adsorption and the adsorption sites were evenly distributed on the surface of biochars (Citation45, Citation46).
The saturated adsorption capacities for Cr(VI) of BC, FBC, and FBCP were 1.948, 7.907, and 12.625 mg/g, respectively. The saturated adsorption capacities for Cr(VI) of FBC and FBCP were 4.06 and 6.48 times that of BC, respectively. The ratio of adsorption rate to desorption rate (KL) was 0.015, 0.509, and 0.603 for BC, FBC, and FBCP, respectively. A larger KL indicates a more spontaneous reaction, more stable product (Citation47, Citation48), and stronger adsorption capacity for Cr(VI).
The equilibrium adsorption capacity of modified biochar was improved to varying degrees. Combined with the above characterization analysis, it was demonstrated that the pore structure and specific surface area of FBC and FBCP were larger than those of BC and the extent of adsorption sites on the surface of biochar increased. This resulted in an increased contact area between biochar and pollutants in solution, thus increasing the saturated adsorption capacity of modified biochar for Cr(VI) in water, compared with unmodified biochar.
3.2.4. Adsorption thermodynamics
The thermodynamic fitted parameters of Cr(VI) adsorption by the three biochars at different temperatures are shown in . The ΔHθ values of the three biochars for Cr(VI) adsorption all exceeded zero, indicating that the adsorption process was an endothermic reaction (Citation49). The values of ΔSθ were also above zero, and FBCP (ΔSθ)> FBC(ΔSθ)> BC(ΔSθ), indicating that FBC and FBCP increased the system chaos during the adsorption process, so that the free movement of Cr(VI) in the solution was enhanced and the degree of freedom was increased (Citation50). The ΔGθ values of Cr(VI) adsorption by the three biochars were all below zero, indicating that the adsorption reaction was spontaneous; as the temperature increased, ΔGθ increased, demonstrating that elevated temperatures increased the adsorption effect (Citation51). At higher initial concentrations of Cr(VI), ΔGθ was lower compared with lower Cr(VI) concentrations at an equivalent temperature, indicating that the adsorption process was mainly controlled by diffusion, and the mass transfer driving force was not the main factor controlling the adsorption process (Citation52).
Table 4. Thermodynamic Fitting Parameters of Cr(VI) Adsorption on BC, FBC and FBCP.
4. Conclusions
In this study, iron-doped phosphorus-based modified sesame straw biochar was used to adsorb Cr(VI) in water. The adsorption kinetic analysis showed that the adsorption process of Cr(VI) in water by modified biochar was mainly chemical adsorption. The isothermal adsorption analysis showed that the Langmuir adsorption isotherm model best described the adsorption process of Cr(VI), and the adsorption process of Cr(VI) by modified biochar was multi-molecular layer adsorption. The saturated adsorption capacity for Cr(VI) of FBC, fitted by the Langmuir isothermal adsorption model, was 7.907 mg/g, and the saturated adsorption capacity for Cr(VI) of FBCP was 12.625 mg/g, which was about 4.06 and 6.48 times that of BC, respectively. The ratio between the adsorption and desorption rates (KL) was highest for FBCP (0.603), intermediate for FBC (0.509), and lowest for BC (0.015). The larger the KL, the more spontaneous the reaction, the more stable the product, and the stronger the adsorption capacity of modified biochar for Cr(VI). The adsorption of Cr(VI) by FBC and FBCP occurred by spontaneous endothermic reaction, and higher temperatures were more conducive to adsorption.
Disclosure statement
No potential conflict of interest was reported by the author(s).
References
- Zheng, J.; Lv, J., Liu, W., Dai, Z., Liao, H., Deng, H., and Lin, Z. Selective Recovery of Cr from Electroplating Nanosludge via Crystal Modification and Dilute Acid Leaching. Environ. Sci-Nano 2020, 7 (5), 1593–1601. doi: 10.1039/d0en00196a.
- Kaur, M.; Kumar, A., Mehra, R., and Kaur, I. Quantitative Assessment of Exposure of Heavy Metals in Groundwater and Soil on Human Health in Reasi District, Jammu and Kashmir. Environ. Geochem. Health 2020, 42 (1), 77–94. doi: 10.1007/s10653-019-00294-7.
- Chen, B.; Wang, M., Duan, M., Ma, X., Hong, J., Xie, F., Zhang, R., and Li, X. In Search of key: Protecting Human Health and the Ecosystem from Water Pollution in China. J. Cleaner Prod. 2019, 228, 101–111. doi: 10.1016/j.jclepro.2019.04.228.
- Wang, Z.; Yang, J., Li, Y., Zhuaang, Q., and Gu, J. In Situ Carbothermal Synthesis of Nanoscale Zero-Valent Iron Functionalized Porous Carbon from Metal-Organic Frameworks for Efficient Detoxification of Chromium(VI). Eur. J. Inorg. Chem. 2018, 1, 23–30. doi: 10.1002/ejic.201701089.
- Ramli, N. N.; Othman, A. R., Kurniawan, S. B., Abdullah, S. R. S., and Hasan, H. A. Metabolic Pathway of Cr(VI) Reduction by Bacteria: A Review. Microbiol. Res. 2023, 268. doi: 10.1016/j.micres.2022.127288.
- Singh, V.; Abhishek, K., Rai, S. N., Sing, S. K., Vamanu, E., and Kumar, A. Source of Cr(VI) in the Aquatic Ecosystem, its Genotoxic Effects and Microbial Removal from Contaminated Water. Green Chem. Letters Rev 2023, 16 (1). doi: 10.1080/17518253.2023.2267079.
- Mao, L.; Su, P., Huang, B., and Zhang, W. Y. Detoxification of Solid Waste Containing Cr(VI) with Phosphate by Thermal Treatment. Chem. Eng. J. 2017, 314, 114–122. doi: 10.1016/j.cej.2016.12.130.
- Wei, L.; Liu, P., Lin, K., Yi, J., Liou, C., Yang, G., Hsu, H., and Tsai, T. Selective Iron Sorption for on-Line Reclaim of Chromate Electroplating Solution at Highly Acidic Condition. Chem. Eng. J. 2015, 281, 434–443. doi: 10.1016/j.cej.2015.06.080.
- Li, W.; Zhang, C., Wei, X., Zhang H. L., Han, M. J., Sun, W., and Li, W. Z. Efficient Resource Treatment of Hexavalent Chromium Wastewater Based on Lead Carbonate (Cerussite)-Induced Precipitation Separation. Process Saf. Environ. Prot. 2022, 165, 475–486. doi: 10.1016/j.psep.2022.07.039.
- Rivera, G. L. D.; Hernandez, A. M., Cabello, A. F. P., Barragan, E. L. R., Montes, A. L., Escamilla, G. A. F., Rangel, L. S., Vazquez, S. I. S., and De, D. A. Removal of Chromate Anions and Immobilization Using Surfactant-Modified Zeolites. J. Water Process Eng. 2021, 39. doi: 10.1016/j.jwpe.2020.101717.
- Li, Z.; Yu, D., Wang, X., Liu, X., Xu, Z., and Wang, Y. A Novel Strategy of Tannery Sludge Disposalconverting Into Biochar and Reusing for Cr(VI) Removal from Tannery Wastewater. J. Environ. Sci. 2024, 138, 637–649. doi: 10.1016/j.jes.2023.04.014.
- Hadiya, V.; Popat, K., Vyas, S., Varjaani, S., Vithaanage, M., Gupta, V., Delgado, A. N., Zhou, Y., Show, P. L., Bilal, M., et al. Biochar Production with Amelioration of Microwave-Assisted Pyrolysis: Current Scenario, Drawbacks and Perspectives. Bioresour. Technol. 2022, 355. doi: 10.1016/j.biortech.2022.127303.
- Tomczyk, A.; Sokolowska, Z.; Boguta, P. Biochar Physicochemical Properties: Pyrolysis Temperature and Feedstock Kind Effects. Rev. Environ. Sci. Bio-Technol. 2020, 19 (1), 191–215. doi: 10.1007/s11157-020-09523-3.
- Zubiolo, C.; de Santana, H. E. P., Pereira, L. L., Ruzene, D., Silva, D. P., and Freitas, L. S. Bio-Oil Production and Characterization from Corn Cob and Sunflower Stem Pyrolysis. Ind. Eng. Chem. Res. 2023, 63 (1), 65–77. doi: 10.1021/acs.iecr.3c03337.
- Richa, L.; Colin, B., Petrissans, A., Wallace, C., Wolfgrm, J., Quirino, R., Chen, W., and Petrissans, M. Potassium Carbonate Impregnation and Torrefaction of Wood Block for Thermal Properties Improvement: Prediction of Torrefaction Performance Using Artificial Neural Network. Appl. Energy 2023, 351. doi: 10.1016/j.apenergy.2023.121894.
- Yuan, J.; Wang, C., Tang, Z., Chu, T., Zheng, C., Han, Q., Chen, H., and Tan, T. Biochar Derived from Traditional Chinese Medicine Residues: An Efficient Adsorbent for Heavy Metal Pb(II). Arabian Journal Of Chemistry 2024, 17 (3). doi: 10.1016/j.arabjc.2024.105606.
- Huang, Y.; Huang, F., Dong, B., Cao, X., Shang, Y., and Xu, X. Degradation of Ofloxacin by High-Efficiency Almond Shell-Based Biochar via Peroxymonosulfate Activation: A Study of the Effect of Pore Structure. Chem. Eng. J. 2023, 474. doi: 10.1016/j.cej.2023.145757.
- Zhang, L.; Hu, J., Li, C., Chen, Y., Zheng, L., Ding, D., and Shan, S. Synergistic Mechanism of Iron Manganese Supported Biochar for Arsenic Remediation and Enzyme Activity in Contaminated Soil. J. Environ. Manag. 2023, 347. doi: 10.1016/j.jenvman.2023.119127.
- Yi, Y.; Tu, G., Zhao, D., Tsang, P. E., and Fang, Z. Key Role of FeO in the Reduction of Cr(VI) by Magnetic Biochar Synthesised Using Steel Pickling Waste Liquor and Sugarcane Bagasse. J. Cleaner Prod. 2020, 245. doi: 10.1016/j.jclepro.2019.118886.
- Nguyen, T. T.; Chen, H. H., To, T. H., Chang, Y. C., Tsai, C. K., Chen, K. F., and Tsai, Y. P. Development of Biochars Derived from Water Bamboo (Zizania Latifolia) Shoot Husks Using Pyrolysis and Ultrasound-Assisted Pyrolysis for the Treatment of Reactive Black 5 (RB5) in Wastewater. Water 2021, 13 (12). doi: 10.3390/w13121615.
- Oh, S.; Kim, J. Degradation of Phenol by Perborate in the Presence of Iron-Bearing and Carbonaceous Materials. RSC Adv. 2023, 13 (46), 32833–32841. doi: 10.1039/d3ra06986a.
- Guo, M.; Ma, X., Han, X., Zhang, S., Yuan, M., Wang, B., and Li, M. Effects of Corn Stalks Biochar Amendment and Freezing-Thawing on the Cd Adsorption of Saline-Alkali Soil. Soil Sediment Contam. 2022, 31 (8), 925–940. doi: 10.1080/15320383.2021.2024141.
- Liu, Y.; Gao, C., Wang, Y., He, L., Lu, H., and Yang, S. Vermiculite Modification Increases Carbon Retention and Stability of Rice Straw Biochar at Different Carbonization Temperatures. J. Cleaner Prod. 2020, 254. doi: 10.1016/j.jclepro.2020.120111.
- Cao, Y.; Wang, L., Kang, X., Song, J., Guo, H., and Zhang, Q. Insight Into Atrazine Removal by Fallen Leaf Biochar Prepared at Different Pyrolysis Temperatures: Batch Experiments, Column Adsorption and DFT Calculations. Environ. Pollut. 2023, 317. doi: 10.1016/j.envpol.2022.120832.
- Dai, W.; Xu, M., Zhao, Z., Zheng, J., Huang, F., Wang, H., Liu, C., and Xiao, R. Characteristics and Quantification of Mechanisms of Cd2+ Adsorption by Biochars Derived from Three Different Plant-Based Biomass. Arab. J. Chem. 2021, 14 (5). doi: 10.1016/j.arabjc.2021.103119.
- Long, X.; Zhang, R., Rong, R., Wu, P., Chen, S., Ao, J., Fu, Y., and Xie, H. Adsorption Characteristics of Heavy Metals Pb2+ and Zn2+ by Magnetic Biochar Obtained from Modified AMD Sludge. Toxics 2023, 11 (7). doi: 10.3390/toxics11070590.
- Li, M.; Zhang, Z.S., Li, Z., and Wu, H. T. Removal of Nitrogen and Phosphorus Pollutants from Water by FeCl 3 -Impregnated Biochar. Ecol. Eng. 2020, 149. doi: 10.1016/j.ecoleng.2020.105792.
- Fang, L.; Yang, W., Hou, J., Zheng, K., Hussain, A., Zhang, Y., Hou, Z., and Wang, X. Tofukasu-derived Biochar with Interconnected and Hierarchical Pores for High Efficient Removal of Cr(VI). Biochar 2023, 5 (1). doi: 10.1007/s42773-023-00268-0.
- Yi, Y.; Wang, X., Ma, J., and Ning, P. Fe(III) Modified Egeria Najas Driven-Biochar for Highly Improved Reduction and Adsorption Performance of Cr(VI). Powder Technol. 2021, 388, 485–495. doi: 10.1016/j.powtec.2021.04.066.
- Gourai, M.; Nayak, A. K., Nial, P. S., Satpathy, B., Bhuyan, R., Singh, S., and Subudhi, U. Thermal Plasma Processing of Moringa Oleifera Biochars: Adsorbents for Fluoride Removal from Water. RSC Adv. 2023, 13 (7), 4340–4350. doi: 10.1039/d2ra07514h.
- Ding, H.; Liu, J., Li, Q., Liu, Z., Xia, K., Hu, L., Wu, X., and Yan, Q. Highly Effective Adsorption and Passivation of Cd from Wastewater and Soil by MgO- and Fe3O4 -Loaded Biochar Nanocomposites. Front. Environ. Sci. 2023, 11. doi: 10.3389/fenvs.2023.1239842.
- Deng, R.; Huang, D., Wan, J., Xue, W., Lei, L., Wen, X., Liu, X., Chen, S., Yang, Y., Li, Z., et al. Chloro-phosphate Impregnated Biochar Prepared by co-Precipitation for the Lead, Cadmium and Copper Synergic Scavenging from Aqueous Solution. Bioresour. Technol. 2019, 293. doi: 10.1016/j.biortech.2019.122102.
- Tai, S.; Li, Y., Yang, L., Zhao, Y., Wang, S., Xia, J., and Li, H. Magnetic-Transition-Metal Oxides Modified Pollen-Derived Porous Carbon for Enhanced Absorption Performance. Int. J. Environ. Res. Public Health 2022, 19 (24). doi: 10.3390/ijerph192416740.
- Li, J. Adsorption of Cd(II) and Pb(II) by Mg-Modified Straw Biochar. Desalin. Water Treat. 2023, 292, 131–140. doi: 10.3390/app12094725.
- Jin, X.; Liu, R., Wang, H., Han, L., Qiu, M., and Hu, B. Functionalized Porous Nanoscale Fe 3 O 4 Particles Supported Biochar from Peanut Shell for Pb(II) Ions Removal from Landscape Wastewater. Environ. Sci. Pollut. Res. 2022, 29 (25), 37159–37169. doi: 10.1007/s11356-021-18432-z.
- Zhao, Y.; Gao, J., Liang, T., Chen, T., Han, X., Hu, G., and Li, B. Efficient Removal of Cr(VI) by Protonated Amino-Bamboo Char Prepared via Radiation Grafting: Behavior and Mechanism. Sustainability 2023, 15 (18). doi: 10.3390/su151813560.
- Usmani, W.; Inam, M. A., Iftikhar, R., Irfan, I., Adnan, R., Niazi, M. B. K., Khan, R., and Hassan, M. Efficient Removal of Hexavalent Chromium Cr (VI) Using Magnesium-Iron Layered Double Hydroxide Supported on Orange Peel (Mg-Fe LDH@OPP): A Synthetic Experimental and Mechanism Studies. J. Water Process Eng. 2023, 55. doi: 10.1016/j.jwpe.2023.104233.
- Qiu, S.; Zhao, D., Feng, Y. Y., Li, M. M., Liang, X. F., Zhang, L. S., Luo, Y., Zhang, K. Q., and Wang, F. Adsorption Performance and Mechanism of Ca-Al-LDHs Prepared by Oyster Shell and pop Can for Phosphate from Aqueous Solutions. J. Environ. Manag. 2022, 303. doi: 10.1016/j.jenvman.2021.114235.
- Liu, N.; Zhang, Y., Xu, C., Liu, P., Lv, J., Liu, Y., and Wang, Q. Removal Mechanisms of Aqueous Cr(VI) Using Apple Wood Biochar: A Spectroscopic Study. J. Hazard. Mater. 2020, 384. doi: 10.1016/j.jhazmat.2019.121371.
- Yang, W.; Lei, G., Quan, S., Zhang, L., Wang, B., Hu, H., Li, L., Ma, H., Yin, C., Feng, F., et al. The Removal of Cr(VI) from Aqueous Solutions with Corn Stalk Biochar. Int. J. Environ. Res. Public Health 2022, 19 (21). doi: 10.3390/ijerph192114188.
- Zhang, H.; Wu, Z., Shi, Q., Khan, A., Rad, S., Shahab, A., Ullah, H., Ali, E., Arafat, A. A., Zeng, H., et al. Fabrication and Characterization of Magnetic Eucalyptus Carbon for Efficient Cr(VI) Removal in Aqueous Solution and its Mechanisms. Arab. J. Chem. 2023, 16 (9). doi: 10.1016/j.arabjc.2023.105047.
- Singh, S.; Naik, T. S. S. K., Thamaraiselvan, C., Behera, S. K., Pavithra, N., Nath, B., Dwivedi, P., Singh, J., and Ramamurthy, P. C. Applicability of new Sustainable and Efficient Green Metal-Based Nanoparticles for Removal of Cr(VI): Adsorption Anti-Microbial, and DFT Studies. Environ. Pollut. 2023, 320. doi: 10.1016/j.envpol.2023.121105.
- Wu, J.; Yang, J., Feng, P., Wen, L., Huang, G., Xu, C., and Lin, B. Highly Efficient and Ultra-Rapid Adsorption of Malachite Green by Recyclable Crab Shell Biochar. J. Ind. Eng. Chem. 2022, 113, 206–214. doi: 10.1016/j.jiec.2022.05.047.
- Liu, L.; Liu, X., Wang, D., Lin, H., and Huang, L. Removal and Reduction of Cr(VI) in Simulated Wastewater Using Magnetic Biochar Prepared by co-Pyrolysis of Nano-Zero-Valent Iron and Sewage Sludge. J. Cleaner Prod. 2020, 257. doi: 10.1016/j.jclepro.2020.120562.
- Zeghioud, H.; Mouhamadou, S. Easy Recovered Magnetic Bark Biochar for Methylene Blue Removal: Preparation Characterization and Adsorption Parameters Study. Chem Sel 2023, 8 (42). doi: 10.1002/slct.202302788.
- Ma, S.; Ji, J., Mou, Y., Shen, X., and Xu, S. Enhanced Adsorption for Trivalent Antimony by Nano-Zero-Valent Iron-Loaded Biochar: Performance, Mechanism, and Sustainability. Environ. Sci. Pollut. Res. 2023. doi: 10.1007/s11356-023-30299-w.
- Yang, Y.; Zhong, Z., Li, J., Du, H., and Li, Z. Efficient with low-Cost Removal and Adsorption Mechanisms of Norfloxacin, Ciprofloxacin and Ofloxacin on Modified Thermal Kaolin: Experimental and Theoretical Studies. J. Hazard. Mater. 2022, 430. doi: 10.1016/j.jhazmat.2022.128500.
- Ergan, B.T.; Aydin, E.S.; Gengec, E. The Effect of Various Thermochemical Methods on the Production of Biochar and Removal of 2-Naphthol Orange from Wastewater. J. Chem. Technol. Biotechnol. 2023. doi: 10.1002/jctb.7513.
- Panchu, S. E.; Sekar, S., Kolanthai, E., Gandhi, M. B., Sridharan, M. B., and Subbaraya, N. K. Physical Modification of Hydroxyapatite: The Drastic Enhancement of Both Cation (Cd2+) and Anion (F-) Adsorption and Recycling Efficiency. Environ. Sci-Nano 2023, 10 (10), 2701–2719. doi: 10.1039/d3en00297g.
- Qin, H.; Shao, X., Shaghaleh, H., Gao, W., and Hamoud, Y. A. Adsorption of Pb2+ and Cd2+ in Agricultural Water by Potassium Permanganate and Nitric Acid-Modified Coconut Shell Biochar. Agronomy-Basel 2023, 13 (7). doi: 10.3390/agronomy13071813.
- Batool, F.; Qadir, R., Adeeb, F., Kanwal, S., Abdelraahman, E. A., Noreen, S., Albaalawi, B. F. A., Mustaqeem, M., Imtiaz, M., Ditta, A., et al. Biosorption Potential of Arachis Hypogaea -Derived Biochar for Cd and Ni, as Evidenced Through Kinetic, Isothermal, and Thermodynamics Modeling. Acs Omega 2023, 8 (43), 40128–40139. doi: 10.1021/acsomega.3c02986.
- Pathak, S.; Pant, K.K.; Kaushal, P. Analysis of Naphthalene Adsorption from Wastewater Using Activated and non-Activated Biochar Produced from Bagasse. Biomass Convers. Biorefinery 2023. doi: 10.1007/s13399-023-04070-7.