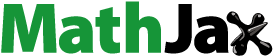
ABSTRACT
The immobilization of enzymes is an important strategy to improve their stability and reusability. However, it often comes with a trade off of reduced biological activity and catalytic efficiency. In this study, a composite hydrogel based on corn stalk carboxymethyl cellulose, acrylic acid, and mesoporous silica SBA-15 was developed as a support for the immobilization of lipase from Pseudomonas S.P. (PSL). The surface morphology and functional properties of the composite hydrogels were investigated by scanning electron microscopy, Fourier transform infrared, X-ray diffraction and simultaneous thermal analyzer, respectively. The composite hydrogel materials had a large number of mesopores and macropores were present in the wall of the hydrogel sheet. The hydrogel had a network structure that reduced the diffusion resistance of the substrate and improved the accessibility of the enzyme. The immobilized PSL exhibited high enantioselectivity (E value: 88.2) and enzyme activity (316.8 µmol/g/min) in the preparation of (S)-ibuprofen by enzymatic hydrolytic resolution of racemic ibuprofen ethyl ester. This prepared novel composite hydrogel is a promising supporting matrix for the immobilization of enzymes.
1. Introduction
Lipases (triacylglycerol acyl hydrolases, EC 3.1.1.3) are widely used in industrial chemistry because of their high specificity and catalytic activity under organic solvents and chemical conditions, and are efficient and environmentally friendly catalysts (Citation1). Lipases are one of the biocatalysts that have attracted much attention in recent years because of their regioselectivity in trans-esterification, hydrolysis and esterification reactions (Citation2). The enzymatic resolution of racemic compounds is a mild method that has emerged as an efficient and industrially productive method (Citation3–5). Ibuprofen is a nonsteroidal anti-inflammatory drug that is commonly used to treat conditions associated with inflammation and pain such as arthritis and neuritis. It has proven to be effective in managing these conditions with few side effects and is generally well tolerated by patients (Citation6). In addition, due to the high enantioselectivity of lipases, lipase-catalyzed resolution of racemic drugs is increasingly used (Citation7). However, their industrial application is still hampered by difficulties in isolation and reuse of free enzymes as well as their low stability at high temperatures, extreme pH and organic solvents (Citation8). Enzyme immobilization on insoluble carriers is an effective optimization strategy for their operational performance in large-scale industrial applications (Citation9,Citation10).
In recent years, the main methods of enzyme immobilization in various carrier materials are physical adsorption, covalent bonding, ion exchange, microencapsulation, and matrix-embedding techniques are widely used (Citation11,Citation12). Among the existing support materials, hydrogels are recognized as an excellent material due to its high hydrophilicity, biocompatibility and biodegradability (Citation13). Hydrogels are hydrophilic polymer with a three-dimensional network structure obtain through physical or chemical cross-linking (Citation14). They are insoluble in water, but can absorb a large amount of water swelling (Citation15). It is because of such structural characteristics that hydrogels are used in a large number of applications in cell separation and culture, controlled release of drugs, immobilization of enzymes and other fields (Citation16). So far, hydrogels as enzyme immobilization materials, generally made of natural or synthetic polymers such as cellulose, chitosan, alginate, gelatin, carrageenan, and poly (vinyl alcohol) (PVA) have attracted great interest as unique carriers (Citation17–20).
Stalk, the stem and leaf fractions remaining after the ripening and threshing of cereal crops such as rice, wheat, and corn (Citation21). The utilization of straw is mainly focused on other low value-added products such as fertilizers and feed, while the development and utilization of high value-added functional materials based on crop straw is still in its early stage (Citation22). The burning of corn straw as agricultural waste not only pollutes the environment, but also causes a waste of biological resources. Corn straw contains 30%–40% of cellulose, hemicellulose and lignin, which are natural long-chain polymers (Citation23). Compared with carbon fiber and glass fiber, straw has the advantages of wide source, low price, renewable, low density, natural environmental protection, etc. It can solve the problems of high cost of composite materials and serious environmental pollution, etc. With people's attention to environmental protection, the preparation of straw-based functional materials with good performance has become a research hotspot of the researchers in this field (Citation24).
Natural cellulose is abundant in nature and it is one of the most widely distributed and abundant polysaccharides. Cellulose is a linear homopolymer that is structurally characterized as a β-anhydroglucoside polysaccharide with a 1,4-β-glycosidic bond. Cellulose is difficult to dissolve in water and organic solvents, making it difficult to react with other compounds and limiting its general application (Citation25). The current modification technology of cellulose mainly focuses on etherification and esterification, and carboxymethylation reaction is one kind of etherification technology (Citation26). Cellulose is carboxymethylated to obtain carboxymethyl cellulose (CMC), which changes its solubility in water and organic solvents, and the aqueous solution of CMC has the functions of thickening, film-forming, bonding, water-retaining, emulsifying and suspending (Citation27). CMC is one of the most important cellulose ethers, which is widely used in industry and has great potential for application in the fields of petroleum, food, pharmaceutical, textile and paper making (Citation28).
In recent years, mesoporous silica materials have attracted extensive attention from researchers in this field due to their structural features such as large specific surface area, large pore volume, tunability of pore structure size, and abundant silanol groups on the surface (Citation29). SBA-15 is a mesoporous molecular sieve with a two-dimensional hexagonal through-hole structure, and the silica on the SBA-15 backbone is generally amorphous (Citation30). The synthesis of SBA-15 is an important chemical technology emerging in recent years, and its high hydrothermal stability has opened up new research areas with a wide range of applications in the disciplines of catalysis, adsorption and separation, biology, nanomaterials and advanced inorganic materials (Citation31,Citation32). In addition, many studies have shown that SBA-15, a mesoporous material with large surface area, high pore volume and abundant surface silanol groups, can make the firmness of the active conformation of the enzyme molecule mechanically stronger and more stable, making it a suitable carrier for enzyme immobilization (Citation33). Enzymes immobilized on pure mesoporous silica materials usually lose their activity rapidly due to leaching of the enzyme (Citation34–36). The mesoporous silica materials and hydrogels effectively adsorb the enzyme, and the hydrogels prevent the enzyme from leaking during operation due to its unique structure (Citation37). Therefore, it is important to immobilize the enzyme on mesoporous silica hydrogel composites (Citation38). However, until now, little information reported on hydrogel composites based on mesoporous silica materials and their application in enzyme immobilization. Therefore, the immobilization of enzyme is important for the study of mesoporous silica hydrogel composites (Citation39–43).
In this paper, acrylic acid (AA) was grafted onto the carboxymethyl cellulose chains of corn straw by free radical polymerization, and mesoporous silica (SBA-15) was successfully embedded into the composite hydrogel. The schematic illustration of CMC-g-PAA/SBA-15 composite hydrogels formation and immobilization PSL enantioselective hydrolysis racemic ibuprofen ethyl ester mechanism can be illustrated in . The structure and morphology of the composite hydrogels were characterized by Fourier transform infrared spectroscopy ATR (FTIR-ATR), X-ray diffraction (XRD), and scanning electron microscopy (SEM) and simultaneous thermal analyzer (TG - DSC). A preliminary study on lipase from Pseudomonas S.P. (PSL) immobilization with the prepared hydrogel composites as carriers was performed. Then, the immobilized PSL was utilized in the resolution of racemic ibuprofen ethyl ester by enantioselective hydrolysis.
2. Materials and methods
2.1. Materials and reagents
Acrylic acid (AA, A.R.), Ammonium persulfate (APS, A.R.), N, N-methylene bisacrylamide (MBA, C.R.) and Lipase from Pseudomonas S.P. (PSL) were purchased from Aladdin Reagent Co., Ltd (Shanghai, China). Ibuprofen ethyl ester of analytical grade was purchased from Sigma-Aldrich Chemical Co., Ltd (Shanghai, China). Hydrochloric acid (HCl, A.R.), sodium hydroxide (NaOH, A.R.) and ethanol were obtained by J&K Scientific Co., Ltd (Beijing China). Other reagents of reagent grade and desiccants were purchased from Shanghai Chemical Reagent Co., Ltd (Shanghai, China). AA was distilled under reduced pressure before use. All other reagents were used as received without further purification and all solutions were prepared with distilled water. All the reagents used in this study were of reagent grade. Corn stalks from autumn fields in Changchun, Jilin province.
2.2. Instruments and characterization
The synthesis of corn stalk carboxymethyl cellulose and composite hydrogels were characterized comprehensively by a various range of techniques. The characterization methods were as follows.
2.2.1. Fourier transform infrared spectroscopy ATR (FTIR-ATR)
Fourier transform infrared spectra ATR (FTIR-ATR) were collected by PerkinElmer FTIR Spectrometer Spectrum Two (PerkinElmer Company, America). FTIR spectrum was collected in the scanning range of 4000–400 cm−1 with the resolution of 4 cm−1. The advantage of this instrument's ATR method is that both powder and liquid samples can be tested directly without the need for testing by mixing and pressing potassium bromide powder. SBA-15, CMC, PSL, immobilized PSL and cellulose extracted from straw could be used directly for ATR, while composite hydrogel samples need to be freeze-dried before they could be tested by ATR.
2.2.2. X-ray diffraction (XRD)
Powder X-ray diffraction (XRD) characterizations were made using a Rigaku Smart Lab X-ray diffractometer (Rigaku Company, Japan), with an operating voltage of 40 kV and a current of 40 mA using Cu Ka radiation. SBA-15, cellulose extracted from straw, and CMC were mounted directly on the sample holders, while the composite hydrogel samples had to be freeze-dried before being mounted on the sample holders, and all tests were scanned in the range of 10–80° in 0.01° increments.
2.2.3. Scanning electron microscopy (SEM)
Scanning electron microscopy (SEM) images were collected on a JEOL-JSM-6700F electron microscope (JEOL Company, Japan). The surface morphologies of SBA-15, cellulose extracted from straw, CMC, and composite hydrogel samples were imaged in ultra-high vacuum mode at an accelerating voltage of 5 kV using a SEM. The samples were placed on monocrystalline silicon wafers and, after freezing in liquid nitrogen, were rapidly transferred to a freeze dryer for 12 h. The dried samples were carefully placed on a conductive adhesive and then coated with gold vapor to make them conductive, followed by SEM examination.
2.2.4. Thermal analysis
The thermogravimetric analysis measurements were performed on a HITACHI STA200 simultaneous thermal analyzer (Hitachi Company, Japan), with a heating rate of 10°C/min from room temperature to 500°C at (N2, Air, Ar) atmosphere.
2.2.4. Proton nuclear magnetic resonance
Proton nuclear magnetic resonance (1H NMR) spectra were recorded on the Bruker AVANCEIII 500. The samples were placed in NMR tubes and dissolved with Chloroform-d for testing.
2.3. Preparation of corn stalk carboxymethyl cellulose
Using corn stalks as raw material, cellulose is extracted using the alkali method, and then cellulose is alkalized and etherified to prepare carboxymethyl cellulose (Citation44). Washed and dried corn stalks and then crushed, through a 60-mesh sieve, the straw powders and 5% NaOH according to the material-liquid ratio of 1:20 mixed, placed in 80°C water bath stirring treatment for 2 h, to remove hemicellulose. Subsequently, the precipitate was filtered and washed, and 0.25 mol/L sodium chlorite was added to the precipitate according to the material-liquid ratio of 1:10, and 10% hydrochloric acid was used to adjust the pH in the range of 6–7, and the lignin was removed by stirring at 75°C for 40 min, and then a large amount of distilled water was used to wash the precipitate, so as to obtain the corn stalk cellulose.
Carboxymethyl cellulose (CMC) was prepared by solvent method. The extracted corn stalk cellulose was mixed with 80% ethanol solution, stirred magnetically at 65°C for 30 min, and then added NaOH and stirred for 2 h. After stirring for 2 h, chloroacetic acid was added and stirred for 1.5 h, and then adjusted the pH of the solution with glacial acetic acid to 6. After the reaction, the extracted corn stalk cellulose was filtered, and then washed with 80% ethanol solution for a number of times, and then dried at 50°C to a constant weight to obtain the carboxymethyl cellulose.
2.4. Acrylic acid neutralization
Pure AA monomer (50 mL) was added to a 200 mL beaker immersed in an ice-water bath, and approximately 35 mL of a 20% NaOH solution was added dropwise with constant stirring to give 70% neutralized acrylic acid (AA). The reaction was exothermic and the neutralization of AA should be carried out at a low temperature to avoid self-polymerization.
2.5. Preparation of the CMC-g-PAA/SBA-15
For hydrogel composites preparation, 1.8 g CMC powder and 50 mL distilled water were placed in a 250 mL four-necked flask. Equipped with mechanical stirrer, thermometer, reflux condenser and nitrogen tube. The mixture was stirred for 30 min and the temperature was slowly increased to 60°C to form a clear viscous solution. After being purged with N2 flushing to remove dissolved oxygen, 5 mL of saturated APS aqueous solution was added for 10 min at 60°C under continuous stirring to generate free radicals. AA (7.2 g) with a neutralization level of 70% were dissolved in 100 ml of water and slowly added to the 250 ml flask under nitrogen blowing. After 5 min, suitable MBA cross-linker and SBA-15 powder were dissolved in 20 mL of deionized water and sonicated for 2 h until the mixture formed a homogeneous solution. The temperature was cooled to 50°C and then slowly pushed into the flask where the CMC radicals were generated using a syringe under a N2 atmosphere. The temperature was then raised to 70°C and kept under N2 atmosphere for 3 h to complete the polymerization reaction. After the obtained hydrogel product was cooled, it was washed several times with distilled water to remove unreacted species and added to a petri dish and dried completely in an oven at 70°C for 2 h. The hydrogel composites were cut into the size of 0.2×0.2×0.2 cm for the next study.
2.6. Immobilization of PSL on CMC-g-PAA/SBA-15
Immobilized PSL was obtained by cross-linking PSL and hydrogel composites through physical adsorption and cross-linking using glutaraldehyde as a bridging ligand. The 10 g of enzyme (PSL) powder was added to 1 L of deionized water, stirred at 4°C for 2 h, and centrifuged at 8000 rpm. The insoluble matter in water was removed and the enzyme solution was lyophilized to obtain a preliminary purified enzyme powder. Dissolve 1 g of lyophilized enzyme powder in 100 mL, pH 7.0 phosphate buffer (10 mM) to make 10 mg/mL of enzyme solution.
The hydrogel composites (0.2×0.2×0.2 cm) were then immersed in 4.5 mL of enzyme solution (10 mg/mL) at 4°C for 24 h. Then, the hydrogel composites were rinsed three times with phosphate buffer solution, and then 4 mL of glutaraldehyde (0.25%–1.25%) at different concentrations were added and reacted for (0.5–2.5 h) at 4°C to achieve complete cross-linking. After washing the excess unbound PSL with deionized water, immobilized PSL was obtained and freeze-dried overnight under vacuum. We measured additional values for the activity of the wash fractions and the protein content of the wash fraction. These values can be used to calculate the immobilization yield, which is crucial in determining the amount of active enzyme that was heterogenized (Citation45,Citation46). The amount of PSL immobilized on the support (320 mg/g) was measured by the Lowry method with BSA as a standard for protein concentration (Citation47).
2.7. Enzymatic hydrolytic resolution of racemic ibuprofen ethyl ester
The standard reaction was carried out in a 20 ml round-bottom flask containing immobilized PSL, 1 mmol racemic ibuprofen ethyl ester, and 10 mL of 50 mM sodium acetate buffer (pH 7.0). The resulting mixture was shaken at 65°C (180 rpm). The above conditions were used for all experiments, unless otherwise stated. The effects of reaction temperature, pH, and reaction time on the synthesis of (S)-ibuprofen were investigated.
2.8. High performance liquid chromatography (HPLC) analysis
HPLC analysis was performed according to the method described by R. Canaparo et al (Citation48). with minor modifications. Briefly, the reaction mixture analysis was performed on a chiral HPLC (chiral OJ-H column, Diacel) at 1 mL/min and monitored by UV (254 nm). The mobile phase was a mixture of 2-propanol: acetic acid (90:10:0.5, v/v/v). UV detection at 254 nm was used for quantification at ambient temperature and (R)-fenoprofen was used as an internal standard (retention time was 9.8 min). We took samples at 30-min intervals. The reaction was quenched to ensure no further product formation after taking the sample. The sample (20 μL) was injected and the system mobile phase was kept in equilibrium for at least five minutes before the next injection of the specimen. The retention times of (S)-ibuprofen and (R)-ibuprofen ethyl ester were respectively 13.1 and 4.8 min. The enantiomeric excess value (ee) was calculated from the two enantiomeric contents. The reaction's conversion (C) was calculated based on the hydrolysis of ibuprofen ethyl ester. The enzyme activity was calculated by controlling the conversion rate within 15–30%, testing 5–15 points and performing a linear regression. The enzyme activity (µmol/g/min) was defined as the amount (in micromoles) of the produced (S)-ibuprofen per minute per gram of protein content. The enantiomeric ratio (E value) was calculated as follows (Citation49):
(1)
(1)
(2)
(2) where S and R represent the concentrations of the (S, R)-diastereomer and (R, R)-diastereomer, respectively.
2.9. Michaelis–Menten kinetics analysis
The rate study and Michaelis–Menten kinetics are as follows:
(3)
(3) where v (μmol/(L
min)) represents the initial reaction speed,
(μmol/(L
min)) represents the maximum reaction speed,
(μmol/L) represents the substrate concentration,
(μmol/L) represents the Michaelis constant. The reciprocal treatment of the above equation is as follows:
(4)
(4)
Let's draw a straight line from to
, the slope is
, the cross-intercept is
°C we know the value of
.
13 mg of free PSL was weighed and mixed with the enzyme containing different concentrations of racemic ibuprofen ethyl acetate (10、15、25、30、35 μmol/L) and 5 mL of 50 mM sodium acetate buffer (pH 7.0). The reaction was carried out and the initial speed of the reaction was determined. 40 mg of PSL-CMC-g-PAA/SBA-15 was weighed and mixed with the enzyme containing different concentrations of racemic ibuprofen ethyl acetate (10、15、25、30、35 μmol/L) and 5 mL of 50 mM sodium acetate buffer (pH 7.0). The reaction was carried out and the initial speed of the reaction was determined.
3. Results and discussion
3.1. Characterization
3.1.1. FTIR results
The compositions and structures of the substances were determined and analyzed using the changes in infrared absorption spectra. The FTIR spectra of the prepared hydrogel composites and their components were shown in . For cellulose extracted from corn stalks, the stretching absorption peaks of 3464, 1051 and 2909 cm−1 were the O-H, C–H and C–O–C bond stretching vibration, respectively. The stretching absorption peaks of 1423 and 1322 cm−1 were resulted from the bending vibrations of C–H and O-H, respectively. For synthesized carboxymethyl cellulose, the stretching absorption peaks at 1589 cm−1, attributed to COO- stretching vibration. The spectrum of SBA-15 showed characteristic peak absorption bands of Si-O-Si stretching vibrations at 1078 and 801 cm−1. The band at 957 cm−1 was due to the stretching vibrations of Si-OH.
Figure 2. The FT-IR spectra of (a) SBA-15, (b) Cellulose extracted from corn stalks, (c) CMC, (d) CMC-g-PAA/SBA-15(12 wt% CMC, 0.6 wt% SBA-15, and 0.6 wt% MBA), (e) CMC-g-PAA/SBA-15(12 wt% CMC, 0.6 wt% SBA-15, and 0.5 wt% MBA) and (f) CMC-g-PAA/SBA-15(12 wt% CMC, 0.6 wt% SBA-15, and 0.4 wt% MBA)
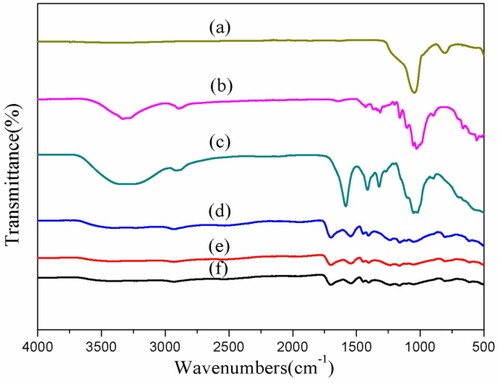
Compared with CMC, SBA-15, there were some new bands appeared on the spectrum of the prepared hydrogel composites ((d–f)). These hydrogel composites were named according to their preparation parameters. It could be seen that the stretching vibration peaks of C-OH of CMC (1061, 1115 and 1159 cm−1) were significantly weakened after copolymerization. The spectrum of CMC-g-PAA/SBA-15 hydrogel composites showed two new bands, one of which was the -COOH stretching vibration peak at 1739cm−1 and the other was the -COO- asymmetric stretch at 1556 cm−1. -COOH and -COO- stretching vibration peaks all originated from PAA. An asymmetric stretching vibrational absorption band of the C = O group on CMC at 1625 cm−1 can be observed, which overlaps with the characteristic absorption peak of the -COO- group of grafted PAA at 1556 cm−1 to form a broad band. It could be observed that the bands corresponding to O-H and Si-OH stretching vibrations shifted slightly from 1051 for CMC and 957 for SBA-15 to around 1100 cm−1 (hydrogel composites), while the shifted bands became wider, suggesting that extensive hydrogen bonding was formed between SBA-15 and CMC and PAA. All these phenomena suggested that SBA-15 was involved in the formation process of composite hydrogels through its reactive groups.
3.1.2. SEM results
To examine the morphology of the aerogels, the samples were analyzed by SEM. Scanning electron microscopy studies were carried out to characterize the surface of the prepared hydrogels. SEM images of SBA-15, cellulose and carboxymethyl cellulose (CMC) and composite hydrogels was observed and shown in . As shown in (a), On the surface of these SBA-15 particles a half-channel stripe structure could be observed, which consisted of unenclosed pore walls and grooves. These stripes were a structural feature of SBA-15 and could be attributed to the uniform arrangement of two-dimensional hexagonal through-holes. As can be seen in (b and c), the cellulose extracted from corn stalks had a fibrous structure, which was chemically modified to carboxymethyl cellulose and then had a rod-like structure. The physical properties changed from insoluble in water to easily soluble in water, which was more favorable for free radical reaction polymerization.
Figure 3. SEM images of (a) SBA-15, (b) Cellulose extracted from corn stalks, (c) Carboxymethyl cellulose, (d) CMC-g-PAA, (e) CMC-g-PAA/SBA-15(12 wt% CMC, 0.6 wt% SBA-15, and 0.5 wt% MBA), (f) CMC-g-PAA/SBA-15 (12 wt% CMC, 0.6 wt% SBA-15, and 0.6 wt% MBA) and (g) CMC-g-PAA/SBA-15(12 wt% CMC, 0.6 wt% SBA-15, and 0.4 wt% MBA).
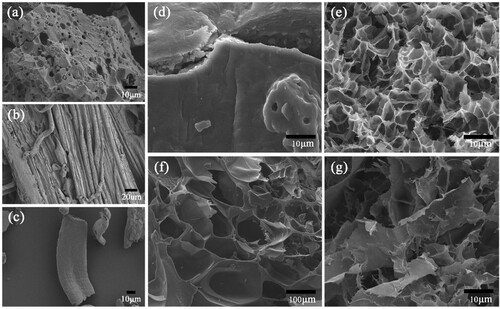
It can be seen that the morphology of the pure hydrogel ((d)) was significantly different from that of the composite hydrogels ((e–g)). The surface of pure CMC-g-PAA ((d)) hydrogel was dense and almost devoid of pore structure. And the surface of the composite hydrogels containing SBA-15 showed the structural characteristics of laminated and porous. Compared to the initial compositions, hydrogel composites with lamellar and porous structures were formed during free radical polymerization and hydrogen bonding cross-linking as shown in (e–g). SBA-15 is an ordered mesoporous silica material with pore-forming properties and rich pore structure on the inner and outer surfaces. The composite hydrogel formed by high cross-linking has thick pore walls, large pores and fewer pore channels ((f)). Composite hydrogels with a low degree of cross-linking had a loose pore structure and had fewer pore structures ((g)). After the addition of suitable amounts of SBA-15 and MBA, the surface morphology of the non-homogeneous composite hydrogel changed significantly, and the surface showed a relatively uniform three-dimensional reticulated pore structure, which was conducive to the immobilization of the enzyme. Therefore, we chose a composite hydrogel with moderate cross-linking degree as the immobilization carrier for the PSL (e).
3.1.3. XRD results
XRD analysis was employed to investigate the crystalline nature of the samples. The XRD patterns of cellulose extracted from corn stalks, SBA-15, carboxymethyl cellulose (CMC) and the prepared hydrogel composites were shown in . Compared with the diffraction peaks of cellulose extracted from corn stalks at 2θ = 15.3°, 21.8° and 34.6°, there was only a broad and weak diffraction peak at 2θ = 22.1°, suggesting that the crystallinity of CMC was low and the crystalline structure might be shifted from cellulose I to cellulose II, which could be due to the alkali treatment. For SBA-15, a broad and weak diffraction peak was observed only at 2θ = 22.9° over the diffraction angle range of 5° to 80°. However, in the synthesized hydrogel composites ((d–f)), the characteristic peak of SBA-15 disappeared and only one characteristic peak was observed at 2θ = 21.2°. This result might be attributed to the grafting reaction as well as hydrogen bond formation and interaction between CMC, PAA and SBA-15 during free radical polymerization.
Figure 4. X-ray diffraction patterns of (a) Cellulose extracted from corn stalks, (b) CMC, (c) SBA-15, (d) CMC-g-PAA/SBA-15(12 wt% CMC, 0.6 wt% SBA-15, and 0.6 wt% MBA), (e) CMC-g-PAA/SBA-15(12 wt% CMC, 0.6 wt% SBA-15, and 0.5 wt% MBA) and (f) CMC-g-PAA/SBA-15(12 wt% CMC, 0.6 wt% SBA-15, and 0.4 wt% MBA)
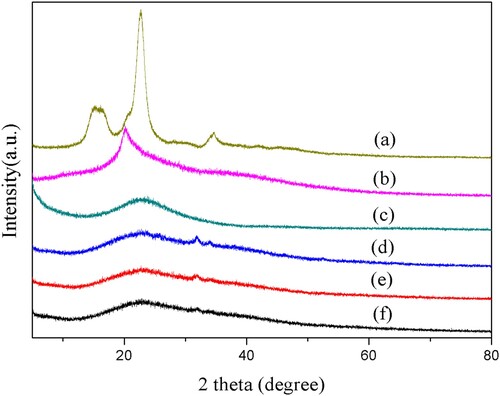
3.1.4. Thermal analysis
The thermal properties of cellulose extracted from corn stalks, PCMC and prepared hydrogel composites were comparatively studied by TG and DSC. As can be seen from (a-e), the TG curves of all experimental samples could be divided into two phases. The first stage at about 100°C was attributed to the evaporation of water associated with the polymer, and the second stage weight loss from 200°C to 380°C was due to degradation of the base polymer. In contrast to the TG curves of cellulose in (a), CMC (b) showed that the presence of carboxymethylated cellulose was significantly different from its natural due to decomposition at higher temperature. Although the modified CMC began to decompose at a lower temperature (about 261°C) than cellulose (289°C), it exhibited a significant weight loss effect with further heating, and it was found that cellulose and CMC retained 11% and 40% of their initial weights at 500°C, respectively. CMC had higher thermal stability, probably due to the lower number of hydroxyl groups after carboxymethylation. As shown in (c and d), after heating the prepared hydrogel composites to 500°C, the CMC-g-PAA/SBA-15 (12 wt% CMC, 0.6 wt% SBA-15, and 0.6 wt% MBA) and CMC-g-PAA/SBA-15 (10 wt% CMC, 0.6 wt% SBA-15, and 0.4 wt% MBA) showed that the contents of CMC and SBA-15 had a positive influence on the thermal stability. The initial decomposition temperatures of the hydrogel composites prepared there in reached 333°C and 315°C, respectively, with improved thermal stability compared to cellulose and carboxymethyl cellulose. The DSC curves of all samples showed an exothermic peak at around 100°C, corresponding to the first stage of weight loss on the TG curve. Further elevation of temperature (>200°C) CMC-g-PAA/SBA-15 (12 wt% CMC, 0.6 wt% SBA-15, and 0.6 wt% MBA) showed almost no peaks and a relatively smooth curve. For the DSC curve of CMC-g-PAA/SBA-15 (10 wt% CMC, 0.6 wt% SBA-15, and 0.4 wt% MBA), weak absorption peaks around 258, 320, 361, 402, and 458°C occured at further elevated temperatures (>200°C), indicating exothermic phenomena. Apparently, the suitable mass ratio of CMC, SBA-15 and MBA improved the thermal stability of the hydrogel composites, which might be a result of enhanced hydrogen bonding between CMC and SBA-15 in the polymer network.
3.2. Lipase-catalyzed enantioselective hydrolysis of ibuprofen ethyl ester
3.2.1. Immobilization of PSL
To confirm whether PSL was successfully immobilized on the PSL-CMC-g-PAA/SBA-15, we studied the FT-IR spectra of PSL and immobilized PSL. As shown in , the strong absorption band at 3270 cm−1 indicated that PSL had a large number of N-H groups. After immobilization by the PSL-CMC-g-PAA/SBA-15 composite hydrogel, the N-H groups formed hydrogen bonds with the hydrogen in the CMC, and the intensity band of this absorption decreased dramatically. In addition, stronger absorption bands associated with C–O stretching (around 1630 cm−1) and C–H deformation (around 1510 cm−1) could be observed in the PSL and PSL-CMC-g-PAA/SBA-15 spectra. These results demonstrated that PSL had been successfully immobilized on PSL-CMC-g-PAA/SBA-15 hydrogel composite.
3.2.2. Comparison of the catalytic properties of immobilized PSL with that of free PSL
In our study, free PSL and immobilized PSL were used for the enantioselective hydrolysis of ibuprofen ethyl ester. The mesoporous silica material and hydrogel can effectively adsorb the enzyme, and the hydrogel can prevent the enzyme from leaking during the operation due to its unique three-dimensional, swellable, and yet, insoluble properties. As shown in , the activity and enantioselectivity of immobilized PSL were improved.
Table 1. Comparison of the catalytic properties of free and immobilized PSL.
Reaction condition: the reaction was carried out in a 20 ml round-bottom flask containing free PSL 13 mg or immobilized PSL 40 mg (protein content: 13 mg), 1 mmol racemic ibuprofen ethyl ester, and 5 mL of 50 mM sodium acetate buffer (pH 7.0). The resulting mixture was shaken at 40°C (180 rpm).
3.2.3. Effect of reaction temperature
Enzyme stability in the environment is one of the most important factors affecting the practical application of biocatalyst. Temperature is a potential factor that may affect both enzyme activity and enantioselectivity. Temperature affects the rate of enzyme rate of the enzymatic reaction for two reasons: first, an increase in temperature increases the thermal energy of the substrate molecules, thereby accelerating the rate of the reaction; Secondly, an increase in temperature increases the thermal energy of the molecules that make up the protein structure of the enzyme molecule itself. This increases the chance of breaking multiple weak non-covalent bonds, which ultimately leads to enzyme inactivity. Therefore, we investigated the effects of temperature on the enzyme performance in this study. We chose a temperature range between 20°C and 60°C to examine the effect of temperature on the catalytic activity of the free and immobilized enzyme and the E value. As shown in (a and b), the enzyme activity increased with increasing temperature, and the catalytic activity of the enzyme reached its maximum when the temperature was 40°C. This might be due to the nature of PSL (its optimal temperature range was 30–40°C). Therefore, 40°C was chosen as the optimal temperature for this reaction.
Figure 7. Effect of temperature on free (a) and immobilized (b) the enzymatic hydrolysis of ibuprofen ethyl ester. The standard reaction was carried out in a 20 ml round-bottom flask containing free PSL 13 mg or immobilized PSL 40 mg (protein content: 13 mg), 1 mmol racemic ibuprofen ethyl ester, and 5 mL of 50 mM sodium acetate buffer (pH 7.0). The resulting mixture was shaken at different temperature (20-60°C) (180 rpm).
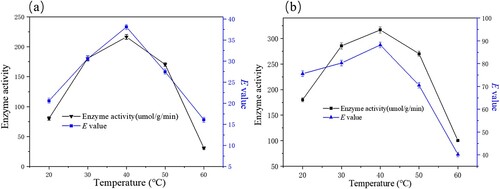
3.2.4. Effect of reaction pH
The effect of pH on the free and immobilized enzymatic hydrolysis of racemic ibuprofen ethyl ester was investigated at pH 5.0–8.0 at 40°C ( (a and b)). The enantioselectivity of the enzymatic racemic ibuprofen ethyl ester was highest when the pH of the buffer was 7.0. The results for free enzyme activity and E valuse were 216.5 μmol/g·min and 38.1, respectively (a). The results for immobilized enzyme activity and E valuse were 316.8 μmol/g·min and 88.2, respectively (b). In fact, alkali saponification occurred at higher pH values rather than by enzymatic hydrolysis to produce the racemate of ibuprofen. Therefore, the optimum pH of the buffer in the system was 7.0.
Figure 8. Effects of pH on the free (a) and immobilized (b) enzymatic hydrolysis of ibuprofen ethyl ester. The standard reaction was carried out in a 20 ml round-bottom flask containing free PSL 13 mg or immobilized PSL 40 mg (protein content: 13 mg), 1 mmol racemic ibuprofen ethyl ester, and 5 mL of 50 mM sodium acetate buffer (pH 5.0-8.0). The resulting mixture was shaken at 40°C (180 rpm).
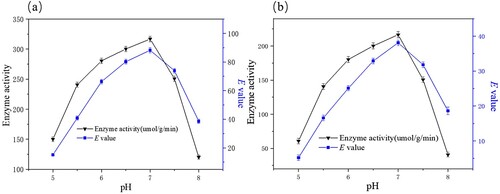
3.2.5. Effect of reaction time
The effect of reaction time (0–5 h) on reaction conversion and enantioselectivity (E value) in the catalytic production of (S)-ibuprofen by immobilized PSL was shown in . There was no significant increase in the yield of (S)-ibuprofen after 4 h of enzymatic reaction. Industrial process reaction times such as enantioselective crystallization and chemically asymmetric synthesis of (S)-ibuprofen were longer than the enzymatic resolution reaction time. In addition, almost all industrial methods involve complex syntheses, require demanding separation techniques, and were difficult to work with. The maximum ibuprofen ethyl ester conversion (47.5%) was obtained when the reaction time was 4 h. We could also observe from the figure that reaction time did not affect the E value.
Figure 9. Effect of time on the free (a) and immobilized (b) enzymatic hydrolysis of ibuprofen ethyl ester. The standard reaction was carried out in a 20 ml round-bottom flask containing free PSL 13 mg or immobilized PSL 40 mg (protein content: 13 mg), 1 mmol racemic ibuprofen ethyl ester, and 5 mL of 50 mM sodium acetate buffer (pH 7.0). The resulting mixture was shaken at 40°C (180 rpm) for (0-4 h).
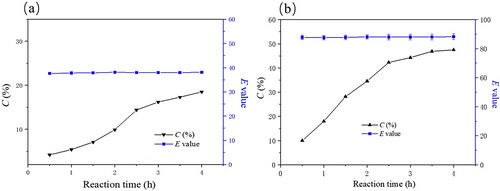
3.2.6. Reusability of enzyme
The recovery efficiency and durability of carrier-immobilized enzymes will be the most desirable features to consider for their practical industrial applications. Therefore, CMC-g-PAA/SBA-15 composite gel immobilization of PSL was investigated for its recoverability (). Notably the PSL-CMC-g-PAA/SBA-15 retained more than 80% of the catalytic activity of the enzyme after four consecutive applications. The composite hydrogel immobilized enzyme activity did not decrease significantly during the first 3 cycles, maintaining more than 90% of the activity. Even after 6 cycles, 47.5% of the initial enzyme activity was still maintained. Measurements in the wash solution did not show any significant activity, indicating that there was no PSL leakage in the newly developed composite hydrogel matrix. Thus, the observed activity was caused by PSL immobilized on hydrogels. However, the loss of enzyme activity in this process was usually mainly due to the leakage of some unimmobilized enzyme components or the accumulation of highly reactive free radicals capturing the catalytic site of the enzyme. It could be hypothesized that the PSL enzyme is effectively entangled in the hydrogel polymer network, thus ensuring that the active form of the enzyme remains remarkably active after several rounds of repeated use.
Figure 10. Reusability of immobilized PSL on the enzymatic hydrolysis of ibuprofen ethyl ester. The standard reaction was carried out in a 20 ml round-bottom flask containing 40 mg immobilized PSL, 1 mmol racemic ibuprofen ethyl ester, and 5 mL of 50 mM sodium acetate buffer (pH 7.0). The resulting mixture was shaken at 40°C (180 rpm).
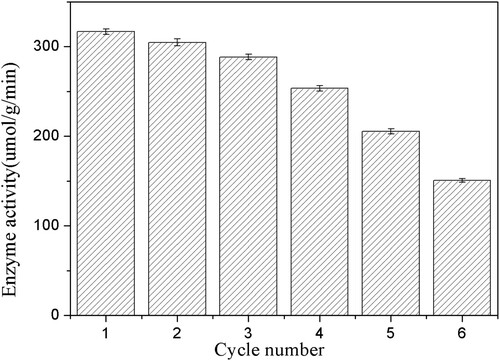
3.3 Structural characterization of (S)-ibuprofen
The immobilized PSL was hydrolyzed to split ibuprofen ethyl ester to synthesize (S)-ibuprofen, and the hydrogen spectrum results are shown in . 1H NMR (400 MHz, Chloroform-d) δ 7.26 (d, J = 8.0 Hz, 2H), 7.14 (d, J = 8.0 Hz, 2H), 3.75 (q, J = 7.2 Hz, 1H), 2.49 (d, J = 7.2 Hz, 2H), 1.88 (hept, J = 13.6, 6.6 Hz, 1H), 1.54 (d, J = 7.2 Hz, 3H), 0.94 (s, 3H), 0.93 (s, 3H).
3.4 Michaelis–Menten kinetics analysis
Michaelis–Menten kinetics were studied as shown in , which could provide valuable insights into the substrate affinity and reaction rate of immobilized PSL compared to free PSL. As shown in the Michaelis constant value of Km for immobilized PSL was 23.5294 (μmol/L), and the Michaelis constant value of Km for free PSL was 28.7852 (μmol/L). From the results, we observed that the immobilized enzyme had a small Km value and the smaller the Km the greater the affinity with the substrate. Therefore the immobilized PSL had better affinity with ibuprofen ethyl ester.
Table 2. Parameters obtained from fitting the Lineweaver- burk polt.
4. Conclusions
In this study, AA was grafted onto CMC by free radical polymerization and SBA-15 was used as filler to prepare eco-friendly CMC-g-PAA/SBA-15 hydrogel composites that could be used as an effective carrier for PSL immobilization. The characteristics of the hydrogel composites were analyzed by FTIR-ATR, XRD, SEM, TG and DSC. FTIR-ATR and XRD results confirmed that CMC was grafted with PAA to form a polymer and that there was a strong hydrogen interaction with SBA-15. SEM images showed that the hydrogel composites have a porous structure contributing to the effective adsorption of PSL. The TG and DSC curves verified that PCMC and SBA-15 had a positive effect on enhancing the thermal stability of hydrogel composites. Immobilized PSL was used for enantioselective hydrolysis for the preparation of (S)-ibuprofen and showed excellent biocatalytic properties (enzyme activity, 316.8 µmol/g/min; E value, 88.2). In conclusion, PSL immobilized by CMC-g-PAA/SBA-15 is a sustainable and promising catalyst for biocatalysis. In addition, this approach may be extended to several other industrially relevant enzymes with novel functions, particularly in the enantioselective hydrolysis of racemic drugs.
Disclosure statement
No potential conflict of interest was reported by the author(s).
Additional information
Funding
References
- Fernandez-Lafuente, R. Lipase from Thermomyces Lanuginosus: Uses and Prospects as an Industrial Biocatalyst. J. Mol. Catal. B Enzym. 2010, 62, 197–212.
- Gamayurova, V.S.; Zinov’eva, M.E.; Shnaider, K.L.; Davletshina, G.A. Lipases in Esterification Reactions: A Review. Catal. Ind. 2021, 13, 58–72.
- Rodrigues, R.C.; Ayub, M.A.Z. Effects of the Combined use of Thermomyces Lanuginosus and Rhizomucor Miehei Lipases for the Transesterification and Hydrolysis of Soybean oil. Process. Biochem. 2011, 46, 682–688.
- Robles-Medina, A.; González-Moreno, P.A.; Esteban-Cerdán, L.; Molina-Grima, E. Biocatalysis: Towards Ever Greener Biodiesel Production. Biotechnol. Adv. 2009, 27, 398–408.
- Fjerbaek, L.; Christensen, K.V.; Norddahl, B. A Review of the Current State of Biodiesel Production Using Enzymatic Transesterification. Biotechnol. Bioeng. 2009, 102, 1298–1315.
- Chávez-Flores, D.; Salvador, J.M. Commercially Viable Resolution of Ibuprofen. Biotechnol. J. 2009, 4, 1222–1224.
- Shanbhag, V.; Crider, R.; Gokhale, A.M.; Harpalani, R.; Dick, R.M. Ester and Amide Prodrugs of Ibuprofen and Naproxen: Synthesis, Anti-Inflammatory Activity, and Gastrointestinal Toxicity. J. Pharmaceut. Sci. 1992, 81, 149–154.
- Sánchez, A.; Valero, F.; Lafuente, J.; Solá, C. Highly Enantioselective Esterification of Racemic Ibuprofen in a Packed bed Reactor Using Immobilized Rhizomucor Miehei Lipase. Enzyme Microb. Technol. 2000, 27, 157–166.
- Yousefi, M.; Mohammadi, M.; Habibi, Z. Enantioselective Resolution of Racemic Ibuprofen Esters Using Different Lipases Immobilized on Octyl Sepharose. J. Mol. Catal. B Enzym. 2014, 104, 87–94.
- Garcia-Galan, C.; Berenguer-Murcia, Á; Fernandez-Lafuente, R.; Rodrigues, R.C. Potential of Different Enzyme Immobilization Strategies to Improve Enzyme Performance. Adv. Synth. Catal. 2011, 353, 2885–2904.
- Bolivar, J.M.; Woodley, J.M.; Fernandez-Lafuente, R. Is Enzyme Immobilization a Mature Discipline? Some Critical Considerations to Capitalize on the Benefits of Immobilization. Chem. Soc. Rev. 2022, 51, 6251–6291.
- Fernandez-Lafuente, R. Editorial for Special Issue: Enzyme Immobilization and Its Applications. Molecules 2019, 24, 4619–4623.
- Garcia-Galan, C.; Berenguer-Murcia, Á; Fernandez-Lafuente, R.; Rodrigues, R.C. Potential of Different Enzyme Immobilization Strategies to Improve Enzyme Performance. Adv. Synth. Catal. 2011, 353, 2885–2904.
- Bayramoglu, G.; Altintas, B.; Arica, M.Y. Immobilization of Glucoamylase Onto Polyaniline-Grafted Magnetic Hydrogel via Adsorption and Adsorption/Cross-Linking. Appl. Microbiol. Biot. 2013, 97, 1149–1159.
- Kim, M.H.; An, S.; Won, K.; Kim, H.J.; Lee, S.H. Entrapment of Enzymes Into Cellulose- Biopolymer Composite Hydrogel Beads Using Biocompatible Ionic Liquid. J. Mol. Catal. B Enzym. 2012, 75, 68–72.
- Gan, S.; Zakaria, S.; Chia, C.H.; Padzil, F.N.M.; Ng, P. Effect of Hydrothermal Pretreatment on Solubility and Formation of Kenaf Cellulose Membrane and Hydrogel. Cararbohyd. Polym. 2015, 115, 62–68.
- Wang, L.; Wang, M. Removal of Heavy Metal Ions by Poly (Vinyl Alcohol) and Carboxymethyl Cellulose Composite Hydrogels Prepared by a Freeze-Thaw Method. Acs. Sustain. Chem. Eng. 2016, 4, 2830–2837.
- Wu, R.; He, B.; Zhang, B.; Zhao, G.; Li, J.; Li, X. Preparation of Immobilized Pectinase on Regenerated Cellulose Beads for Removing Anionic Trash in Whitewater from Papermaking. J. Nanobiotechnol. 2014, 89, 1103–1109.
- Wang, S.; Zhang, C.; Qi, B.; Sui, X.; Jiang, L.; Li, Y.; Wang, Z.; Feng, H.; Wang, R.; Zhang, Q. Immobilized Alcalase Alkaline Protease on the Magnetic Chitosan Nanoparticles Used for soy Protein Isolate Hydrolysis. Eur. Food. Res. Technol. Echnol. 2014, 239, 1051–1059.
- Liu, S.; Luo, W.; Huang, H. Characterization and Behavior of Composite Hydrogel Prepared from Bamboo Shoot Cellulose and β-Cyclodextrin. Int. J. Biol. Macromol. 2016, 89, 527–534.
- Wu, X.M.; Hu, C.C. Greener Solution to Waste Corn Stalks and Shortage of Asphalt Resource: Hydrochar Produced by Hydrothermal Carbonization as a Novel Performance Enhancer for Asphalt Binder. Materials. (Basel) 2021, 14, 1427–1441.
- Wang, W.; Yang, D.; Mou, L.; Wu, M.; Wang, Y.; Tan, F.; Yang, F. Remodeling of Waste Corn Stalks Into Renewable, Compressible and Hydrophobic Biomass-Based Aerogel for Efficient and Selective oil/Organic Solvent Absorption. Colloid. Surface. A. 2022, 645, 128940–128940.
- Zou, Y.P.; Hu, J.H.; Zhang, S.Y.; Shi, K.X.; Liu, X.C.; Zhao, S.J.; Yang, H.J.; Jia, J.L. Carbonization Characteristics of co-Pyrolysis of Sewage Sludge and Corn Stalks and its Agricultural Benefits. J. Soil. Sediment. 2023, 23, 1674–1686.
- Xia, Y.D.; Klinger, J.; Bhattacharjee, T.; Thompson, V. The Elastoplastic Flexural Behaviour of Corn Stalks. Biosyst. Eng. 2022, 216, 218–228.
- Guo, Y.Z.; Nakajima, T.; Mredha, M.T.I.; Guo, H.L.; Cui, K.; Zheng, Y.; Cui, W.; Kurokawa, T.; Gong, J.P. Facile Preparation of Cellulose Hydrogel with Achilles Tendon-Like Super Strength Through Aligning Hierarchical Fibrous Structure. Chem. Eng. J. 2022, 428, 132040–132050.
- Ayucitra, A.; Angkawijaya, A.E.; Ju, Y.; Gunarto, C.; Ismadji, S. Graphene OxideヽArboxymethyl Cellulose Hydrogel Beads for Uptake and Release Study of Doxorubicin. Asia-pac. J. Chem. Eng. 2021, 16, 26461–264610.
- Li, H.L.; Zhang, H.R.; Xiong, L.; Chen, X.F.; Wang, C.; Huang, C.; Chen, X.D. Isolation of Cellulose from Wheat Straw and Its Utilization for the Preparation of Carboxymethyl Cellulose. Fiber. Polym. 2019, 20, 975–981.
- Casaburi, A.; Rojo, ÚM; Cerrutti, P.; Vázquez, A.; Foresti, M.L. Carboxymethyl Cellulose with Tailored Degree of Substitution Obtained from Bacterial Cellulose. Food. Hydrocolloids. 2018, 75, 147–156.
- Ghaedi, H.; Zhao, M. Review on Template Removal Techniques for Synthesis of Mesoporous Silica Materials. Energy Fuels. 2022, 36, 2424–2446.
- Mazzotta, E.; Santo, M.D.; Lombardo, D.; Leggio, A.; Pasqua, L. Mesoporous Silicas in Materials Engineering: Nanodevices for Bionanotechnologies. Materials Today Bio. 2022, 17, 100472–100489.
- Larki, A.; Saghanezhad, S.J.; Ghomi, M. Recent Advances of Functionalized SBA-15 in the Separation/Preconcentration of Various Analytes: A Review. Microchem. J. 2021, 169, 106601–106618.
- Marcucci, S.M.P.; Zanin, G.M.; Arroyo, P.A. Synthesis of SBA-15 and Pore-Expanded SBA-15 and Surface Modification with tin for Covalent Lipase Immobilization. Micropor Mesopor. Mat. 2022, 337, 111951–111968.
- Bhange, P.; Sridevi, N.; Bhange, D.S.; Prabhune, A.; Ramaswamy, V. Immobilization of Bile Salt Hydrolase Enzyme on Mesoporous SBA-15 for co-Precipitation of Cholesterol. Int. J. Biol. Macromol. 2014, 63, 218–224.
- Zhang, H.; Zhang, X.; Wang, B.; Zeng, X.; Guo, H.Z.; Ren, B.; Yang, X.D. Immobilization of Laccase Onto Functionalized Ionic Liquid-Modified Mesoporous Silica SBA-15. Biocatal. Biotransfo. 2021, 3, 1–11.
- Santis, P.D.; Meyer, L.k.; Kara, S. The Rise of Continuous Flow Biocatalysis –Fundamentals, Very Recent Developments and Future Perspectives. React. Chem. Eng. 2020, 5, 2155–2184.
- Meyer, J.; Meyer, L.E.; Kara, S. Enzyme Immobilization in Hydrogels: A Perfect Liaison for Efficient and Sustainable Biocatalysis. Eng. Life. Sci. 2021, 22, 165–177.
- Meyer, L.E.; Horváth, D.; Vaupel, S.; Meyer, J.; Alcalde, M.; Kara, S. A 3D Printable Synthetic Hydrogel as an Immobilization Matrix for Continuous Synthesis with Fungal Peroxygenases. React. Chem. Eng. 2023, 8, 984–988.
- Bat-Ozmatara, M.; Ünlü, A.; Gevrek, T.N. Preparation of Isocyanate-Containing Hydrogel Films as Antibacterial Enzyme Immobilization Matrices. React. Funct. Polym. 2023, 192, 105695–105710.
- Dai, H.J.; Ou, S.Y.; Liu, Z.J.; Huang, H.H. Pineapple Peel Carboxymethyl Cellulose/Polyvinyl Alcohol/Mesoporous Silica SBA-15 Hydrogel Composites for Papain Immobilization. Carbohyd. Polym. 2017, 169, 504–514.
- Solra, M.; Das, S.; Srivastava, A.; Sen, B.; Rana, S. Temporally Controlled Multienzyme Catalysis Using a Dissipative Supramolecular Nanozyme. ACS Appl. Mater. Interfaces. 2022, 14, 45096–45109.
- Srivastava, A.; Kaur, H.; Pahuja, H.; Rangarajan, T.M.; Varma, R.S.; Pasricha, S. Optimal Exploitation of Supported Heterogenized Pd Nanoparticles for C-C Cross-Coupling Reactions. Coord. Chem. Rev. 2024, 507, 215763–215802.
- Zhao H.W.; Han H. Synthesis and Characterization of Functionalized SBA-15 Silica Through Template Removal. 2020, 282, 121074–121089.
- Zandieh, M.; Liu, J.W. Nanozymes: Definition, Activity, and Mechanisms. Adv. Mater. 2023, 36, 2211041–2211048.
- Fu, T.K.; Li, J.H.; Wang, Q.H.; Huang, H.; Wei, X.Y.; Cui, L.H.; Wang, Y.H.; Wang, F. Structure and Properties of Natural Cellulose Extracted from Pineapple Leaf. A. M. R. 2013, 815, 379–385.
- Petermeier, P.; Bittner, J.P.; Müller, S.; Byström, E.; Kara, S. Design of a Green Chemoenzymatic Cascade for Scalable Synthesis of bio-Based Styrene Alternatives. Green. Chem. 2022, 24, 6889–6899.
- De Santis, P.; Petrovai, N.; Meyer, L.E.; Hobisch, M.; Kara, S. A Holistic Carrier-Boundimmobilization Approach Forunspecific Peroxygenase. Front. Chem. 2022, 10, 985997–986107.
- Yu, D.H.; Wang, Z.; Zhao, L.; Cheng, Y.M.; Cao, S.G. Resolution of 2-Octanol by SBA-15 Immobilized Pseudomon as s.p. Lipase. J. Mol. Catal. B Enzym. 2007, 48, 64–69.
- Canaparo, R.; Muntoni, E.; Zara, G.P.; Pepa, C.D.; Berno, E.; Costa, M.; Eandi, M. Determination of Ibuprofen in Human Plasma by High-Performance Liquid Chromatography: Validation and Application in Pharmacokinetic Study. Biomed. Chromatogr. 2000, 14, 219–226.
- Chen, C.S.; Fujimoto, Y.; Girdaukas, G.; Sih, C.J. Quantitative Analyses of Biochemical Kinetic Resolutions of Enantiomers. J. Am. Chem. Soc. 1982, 104, 7294–7299.