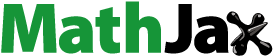
Abstract
A successful crop rotation choice is key to the profitability and sustainability of farm management and may simultaneously have an impact on soil organic carbon (SOC) content. In this study, we estimated how changes in crop rotations affected SOC balance in Finland between 2009 and 2018, using geospatial data and Bayesian modeling. The area designated for perennial-dominated and diverse cereal rotations increased over the study period. Perennial grassland rotation was found to have a positive impact on SOC balance, while rotations dominated by annual crops did not differ in their impacts on SOC content. At the national scale, changes in Finnish crop rotations resulted in an estimated annual mitigation of the loss of SOC content by 1336 Mg C year−1 in mineral soils and reduced the carbon dioxide emissions of organic soils by 10,475 Mg C year−1. The combined effect of these two contributions is 11,811 Mg C year−1, with an 80% probability interval of (−6600; 30,300) Mg C year−1. While the overall impact of changes in crop rotations on SOC is relatively small, a continued change to more diverse and perennial-dominated crop rotations may have other agronomic and environmental benefits, e.g. on resilience and biodiversity.
Introduction
Soil stores a significant amount of carbon as soil organic matter [Citation1,Citation2]. Given the enormous absolute size of this carbon stock, even relatively minor changes in soil organic carbon (SOC) content can have a significant effect on the CO2 level of the atmosphere. It has been suggested that with proper management, soils could store significantly more carbon than presently, and carbon sequestration in soils could therefore be utilized to counteract anthropogenic greenhouse gas (GHG) emissions [Citation3,Citation4]. It has even been proposed that improved soil management strategies could lead to an accrual of SOC stock at a rate that would substantially compensate for the CO2 emissions from the consumption of fossil fuels [Citation5,Citation6], although some other researchers regard such an objective unattainable [Citation7–10]. An additional driving force for SOC accrual in arable topsoil is the role of SOC content in maintaining and improving important soil physical functions [Citation11,Citation12]. However, despite the calls to increase SOC stocks, several European studies indicate that SOC content is systematically decreasing in agricultural soils [Citation13–18].
In general, soil management practices may significantly influence soil carbon sequestration [Citation6,Citation19], and diverse crop rotations, including perennial grasses, promote SOC accrual [Citation18]. Long-term modeling of SOC dynamics has indicated that the inclusion of grasslands within crop rotation and extending their duration are effective means of increasing SOC stocks compared to many other management practices [Citation20,Citation21]. Grassland establishment leads to high inputs of organic matter and reduces soil disturbance, which slows the degradation of organic matter. In young grasslands, this leads to the accumulation of SOC, which can persist over long periods [Citation22]. However, tillage of grasslands or even a single plowing event in a grass-to-grass renovation have been found to significantly reduce carbon stocks [Citation23,Citation24]. In crop rotations including perennial grasslands, these counteracting processes lead to a new SOC equilibrium, and the increase in the proportion of temporary grasslands in the rotation may have an incremental effect on the SOC accrual [Citation25].
After a change in soil management, SOC stocks evolve toward a new steady state, which may mean either a higher or a lower SOC content, depending on how the management practices are altered. Typically, the time needed to reach a new equilibrium is long, which is why it can be difficult to determine whether a soil has reached a stable level of SOC at a given time [Citation26]. After deploying new management practices, the SOC change typically occurs on a decadal time scale [Citation27] but may in some cases take more than a century [Citation22,Citation28]. To further complicate the issue, SOC loss related to the conversion of cropland to grassland may happen on a shorter time scale than the restoration of SOC in the reverse process [Citation29]. It is thus likely that most soils are in a transient state between two equilibria, and their SOC content does not fully reflect the SOC stock corresponding to the current SOC inputs and degradation.
Changes in SOC content following the deployment of novel soil management practices have been determined in long-term experiments [Citation27,Citation30–32]. Oscillations in SOC have been observed during crop rotation phases where certain phases accumulate, and others diminish, SOC [Citation33]. It has also been found that equilibrium SOC values may change over time due to the continuously changing climate [Citation26].
In Finnish arable soils, the SOC content has been found to decrease almost linearly in the last four decades [Citation16]. Changes in SOC stock have been associated with land-use history, management practices, and climate change [Citation18]. This study aims to explore the recent changes in crop rotations in Finland and to quantify their impact on the nationwide SOC content by combining several geospatial datasets with the results of the national soil monitoring network. We aim to quantify the overall effect of the ongoing short-term changes in crop rotations on SOC balance and to put this into perspective by comparing it with Finland’s total annual GHG emissions.
Materials and methods
The cultivated areas for each crop rotation in Finland were calculated based on two geospatial datasets: (1) the database of management and cultivated crop plants by the Finnish Food Authority; and (2) the Finnish Soil Database [Citation34]. The Soil Database was used to identify whether the soil type of a field parcel should be classified as a mineral or an organic soil. Changes in SOC content in mineral soils were estimated by combining the results of the national soil monitoring network with the European observational (E-OBS) climate data grid [Citation35] and the database of the Finnish Food Authority. Emissions of organic soils were estimated by using default emissions factors by the Intergovernmental panel on climate change (IPCC) [Citation36]. A flowchart of the analysis is presented in .
Figure 1. Schematic illustration of the workflow and the geospatial datasets used in the study. Notes: SOC refers to soil organic carbon, E-OBS is the European observational climate grid, and IPCC is the Intergovernmental Panel on Climate Change.

National soil monitoring network
Finland’s arable soil monitoring network was established in 1974, and plots were resampled in 1987, 1998, 2009, and 2018. However, coordinates of all sampling plots have been determined using Global positioning system (GPS) from 2009 onward, resulting in much less scatter in the data due to the inaccuracy of the sampling plot location compared with earlier sampling campaigns (see [Citation16,Citation18]). This study was therefore based on the SOC content of the mineral soil samples collected in 2009 and 2018. In 2018, the soil monitoring network had a total of 620 sampling plots. For this study, the number of sampling plots was 323, as organic soils (as defined by organic matter > 20%), plots that had not been sampled in both 2009 and 2018, and plots for which crop rotation could not be classified were omitted. presents the location of the plots.
Figure 2. Location of parcel-scale sampling plots of the Finnish national soil monitoring network utilized in this study (n = 323).

Soil samples were collected from 10 m × 10 m areas using a soil corer with a diameter of 2 cm. Samples were taken from the topmost 15 cm soil layer. The samples were air-dried, ground, and passed through a 2 mm sieve. The soil organic C content of the samples was determined by the dry combustion method using a LECO CN-2000 analyzer (LECO). Soil texture was determined using the sieve-pipette method [Citation37].
Classification of crop rotations
The crop rotations were classified as in earlier studies [Citation38]. The classification is based on the occurrence of different crop species in a five-year rotation. Rotations were classified for two periods: from 2009 to 2013, and from 2014 to 2018, based on the database of the Finnish Food Authority. The above periods were selected because they coincide with the sampling campaigns of the national soil monitoring network.
The crop rotations were classified as: (1) cereal species monoculture rotation, in which the same cereal species was grown in a field for at least four years (usually barley (Hordeum vulgare L.) or oats (Avena sativa L.) only); (2) cereal monoculture rotation, in which two or more cereal species were included in the rotation (often spring or winter wheat (Triticum aestivum L.) and winter rye (Secale cereale L.)); (3) a rotation with a break-crop, in which a crop species other than cereals (often a grain legume or rapeseed) appeared once in the cereal-dominated crop rotation; (4) diverse crop rotation, in which spring and winter cereals appeared for one or two years, and at least two other crops were included in the rotation; (5) perennial grassland rotation, in which nonpermanent grass was produced for at least three years (the fields used for grazing and ones with harvested hay or silage could not be differentiated); and (6) green fallow rotation, in which green fallow, nature-managed fields, or diverse game fields were grown for at least three years. Fields in the green fallow rotation encompass a range of extensively managed fields sown with perennial or annual grasses, meadow plants or forage plants with the purpose of increasing biodiversity and providing feed and shelter for wild game animals (moose, dear, fowl). These fields may also be used for grazing and need to be mown to prevent growth of trees and bushes.
Farmers often divide field parcels into several agricultural parcels in which different crop species are grown. Locations of agricultural parcels cannot be positioned exactly within the field parcels, and only those field parcels for which the largest agricultural parcel covered ≥70% of the total field parcel area were therefore included in this study.
Effect of crop rotation on SOC
For mineral soils, the statistical analysis is based on a Bayesian framework [Citation18]. The model captures the high and non-trivial spatial variation in SOC content with a mixing model where the changes in SOC levels follow a mix of two normal distributions, one narrower and one wider. Details, justifications, model performance, and extensive discussion of the model and the necessity of each part of it were reported earlier [Citation18]. For this study, the main interest is the effect of crop rotations. In addition, the model was controlled for changes in precipitation and temperature, as well as the organic-carbon-to-fine-soil ratio, which also affects the SOC levels.
The basic idea behind the model is that after controlling for climate, management practices, a random effect associated with the measurement group, and soil properties, the unexplained residual variation in SOC change follows a distribution, which is a mix of two normal distributions (instead of the standard one normal distribution in a basic linear model). The two normal distributions have the same mean, but one is wide, and one is narrow. When the two measurements, which were carried out almost a decade apart, have been done very close to each other, the variation is relatively small, and the observation is from the narrow distribution. However, if the measurements differ in location even by some meters, the variation can be much larger due to the huge spatial variation of SOC, and thus the observation is from the wider distribution. As we cannot know for which plots the re-location has been successful (as it depends on the local variation in SOC), each measurement has some non-zero probability of belonging to either one of the distributions.
The formulation of the model may appear somewhat complicated, but regarding the research questions of this study, the majority of the parameters are nuisance parameters, i.e. parameters which are not of interest, but which are needed to properly capture the structure of the data. Formally, the model can be written as
where for plot
is the change in SOC,
is an intercept term corresponding to cereal species monoculture rotation with no changes in temperature or precipitation, and an organic-carbon-to-fine-soil-ratio of 1,
is an indicator variable which has the value of 1 if the plot
is farmed using the rotation
and 0 otherwise.
and
denote changes in total rainfall and annual average temperature, respectively.
is the organic-carbon-to-fine-soil-ratio,
s are the regression coefficients to be fitted,
is a measurement-group-specific random effect, and the
s form the covariance structure, with
being the standard deviation of the narrow distribution,
that of the wide distribution, and
that of the random effect, with
being the probability that the observation is from the narrow distribution, and
the probability that the observation is from the wide distribution.
refers to a normal distribution and
to a categorical distribution. We use uninformative priors and sufficient efficient sample sizes as specified by Heikkinen et al. [Citation18]. The fit was done using JAGS software (Just Another Gibbs Sampler [Citation39]), and the statistical software R [Citation40].
For the purposes of this study, we are interested in the parameters which describe the difference in annual SOC sequestration/loss between a given rotation
and the cereal species monoculture rotation. With the Bayesian approach, by specifying a model and fitting it with our data we get a probability distribution
known as a posterior distribution, from which we can infer a likely value for the parameter
and its probability distribution. A natural point estimate for the parameter is the expected value of the distribution
). For hypothesis testing, the probabilities
and
are analogous to frequentist one-sided p-values, as they describe the probability that the parameter has a given sign. Probabilities above 95% are considered here as solid evidence of a difference between the rotations. A range of likely values is described by a probability interval (i.e. the posterior interval or Bayes interval) which is analogous to the frequentist confidence interval, but the interpretation is more straightforward, as it directly produces a probability that the value of the parameter is contained within the interval. In Bayesian statistics, the traditional choice is to use an 80% probability interval (80% PI), which we also adopt for this work. For further information about Bayesian statistical analysis, see Gelman et al. [Citation41].
Emissions of organic soils were estimated using the default emissions factors from the IPCC [36], which are 7900 kg C ha−1 year−1 (95% confidence intervals, ranging from 6500 to 9400 kg C ha−1 year−1) for annual crops and 5700 kg C ha−1 year−1 (2900–8600 kg C ha−1 year−1) for perennial grasses. Organic soils were identified using the Finnish Soil Database [Citation34] by extracting the soil type based on the coordinates of the center point of the field parcel.
Results
Changes in crop rotation area
In mineral soils, the cultivated area of cereal species monoculture and rotation with break-crops decreased between 2009–2013 and 2014–2018 (). A decrease by 52,800 ha in cereal species monoculture and 47,100 ha in rotation with break-crops was observed. On the other hand, the greatest increase in cultivated area was found for cereal monoculture rotation (53,100 ha), followed by perennial grassland (28,400 ha) and green fallow rotation (22,100 ha). The cultivation area designated for diverse rotations was found to have increased by 10,000 ha. In mineral soils, the crop rotation class changed in 52% of the cultivated area between the periods 2009–2013 and 2014–2018.
Figure 3. Changes in cultivated area under different crop rotations in the entire country of Finland between 2009–2013 and 2014–2018 in hectars (ha) for (a) mineral and (b) organic soils.

In organic soils, the changes were qualitatively similar to those in mineral soils, with the area occupied by cereal monoculture, diverse rotation, green fallow rotation, and perennial grass rotation increasing, while the total area for other rotations decreased (). However, in organic soils, the greatest area increases were those of green fallow and perennial grassland rotations, with increases by 5100 and 4800 ha, respectively. According to the present data, the share of organic soils is 10.6% of the total agricultural area. In organic soils, the proportion of fields where the rotation changed was 45% between the classification periods 2009–2013 and 2014–2018.
Effect on SOC content
Mineral soils
presents the results from the Bayesian model fit for mineral soils. Perennial grassland rotation stood out as the most beneficial cropping system with respect to SOC accumulation between 2009 and 2018. As indicated by 80% PIs, the perennial grassland rotation has a clear indication of deviating from cereal species monoculture with respect to its effect on SOC concentration. For green fallow rotation the point estimate was also positive, but the size of the effect was smaller – only about 10% of that of the perennial grass rotation – and the 80% PI includes both negative and positive values. The point estimates for monotonous cereal species sequencing, rotation with break-crop, and diverse rotation are negative, meaning that based on this dataset, it is possible that these rotations are counterproductive with respect to SOC accrual compared to cereal species monoculture.
Table 1. Effect of crop rotations, climate change, and SOC-to-fine-fraction ratio on soil organic carbon (SOC) content change ) using E-OBS climate data, 80% (equally tailed) probability interval, and probability of positive effect (P(
> 0)).
Other variables included in the model show that changes in SOC concentration were strongly associated with the initial SOC-to-fine-fraction ratio, indicating that soils with a large amount of SOC or coarse texture tend to lose more carbon than soils with a higher amount of fine fraction particles relative to the SOC concentration. The results also indicate that an increase in both temperature (May–September) and precipitation led to SOC losses. These findings are in line with the earlier study [Citation18] but are beyond the scope of this study, as the variables were included in the analysis only to control for effects unrelated to crop rotations.
The overall national-level impact of crop rotation changes was calculated by combining the estimated changes in SOC content per hectare with the cultivated areas of each conversion class combination (). By summing up the effects of each converted crop rotation class combination, the nationwide accumulation of SOC can be estimated to be approximately 1336 Mg C year−1 in mineral soils. However, the 80% PI is extremely wide, varying from an order of magnitude higher SOC loss to an order of magnitude higher SOC accumulation.
Table 2. Cultivated area of each crop rotation class combination in 2009–2013 and 2014–2018, corresponding emission factors (kg C ha year−1), and overall effect on soil organic carbon (SOC) stock (Mg C year−1). The effects (EF) have been given per hectare (ha).
Organic soils
The area of perennial grassland rotation in organic soils increased by 4800 ha between the classification periods of 2009–2013 and 2014–2018 (). Using the IPCC default emission factors, the increase in area of perennial grassland rotation corresponds to an annual emissions reduction of approximately 10,475 Mg, with the 80% PI varying from an emissions reduction of 476 Mg to 20,476 Mg. Regionally, the emissions of organic soils decreased in the regions where perennial-dominated crop rotations increased in area (). As the uncertainties related to mineral and organic soils can reasonably be assumed to be independent given that the numbers originate in different models and datasets, the combined effect of mineral and organic soils on SOC is 11,811 Mg C year−1, with an 80% PI of (−6600; 30,300) Mg C year−1.
Discussion
The diversification of agricultural land use has been identified [Citation42] as one of the key measures for improving the sustainability and resilience of agriculture. In Finland, cereal species monocultural rotations (usually either barley or oats) have given way to more diverse cereal-dominated rotations and to some extent to diverse rotations. On the other hand, the area designated for a single break-crop during a five-year rotation has been reduced, which is probably attributable to a reduction in the cultivation of turnip (Brassica rapa L.) driven by increased uncertainties and yield losses experienced by farmers [Citation43,Citation44]. Changes in land use to higher diversity in Finland [Citation45] have primarily been driven by systematic increases in farm size, the warming of the climate, and the opening of domestic markets for diversifying special crops [Citation38,Citation45,Citation46]. On the other hand, agricultural policies have increasingly pressed for a transition to the use of environmental grasslands (i.e. green fallow rotations, ), though this has taken place in parcels with a low production capacity and structural, functional, and logistic weaknesses [Citation47].
Among the six crop rotations considered, perennial grassland rotation, which was defined as three or more grass years in a five-year period, was discerned as the most efficient for SOC sequestration. The conversion of cropland to grassland is known to induce SOC accumulation [Citation28,Citation48]. Temporary grass cultivation, i.e. rotational leys, has also been identified as an option for soil carbon management, though potentially not as efficient as the use of grasslands [Citation49,Citation50]. The tendency of perennial vegetation to accumulate SOC is attributed to high root-derived C input, which is more persistent in soils than carbon originating in residues of aboveground biomass [Citation51–53]. In addition, a reduction in the frequency and intensity of disturbance through tillage in rotations containing temporary leys contributes to enhancing SOC storage [Citation54]. The observed rates of gains (or, in some cases, losses) of SOC in grasslands vary depending on soil and environmental conditions (e.g. soil C sink capacity, the age of the ley, temperature, and precipitation) and management practices [Citation55]. Improvements in management, such as the fertilization and sowing of new plant varieties, tend to increase SOC [Citation56,Citation57]. Consequently, in the current data, extensive management may at least partly explain the lack of a positive effect of the green fallow rotation, including nature-managed fields, on SOC content change. Furthermore, in this crop rotation class, fields of inherently low biomass production potential are known to be common [Citation47,Citation58].
An increase in the diversity of plant species within annual rotations did not promote SOC buildup or maintenance according to the current data. Similar results are common in other published crop-rotational studies [Citation59]. However, in a previous study using a different classification for the rotations using Finnish data [Citation18], diverse cropping systems (Shannon index > 0.8) exhibited a positive SOC effect compared with annual less diverse (Shannon index < 0.8) systems. This was thought to be potentially associated with an increased C input, as rotation phases with perennials were also possibly included. Another reason suggested was changes in the chemical composition of plant residues favoring SOC accumulation, changes in soil microbial community, or cultivation operations associated with the diverse rotations [Citation59–61]. In this study, we identified crop rotations based on the five preceding years for both study periods, which may be too short a period to record any large-scale impacts on SOC, even though many diversifying crops cause immediate yield benefits for the following crops in rotation. This was evident according to the large-scale studies on farmers’ fields that used satellite data to identify changes in biomass production capacities depending on pre- and following crop combinations used in crop sequencing [Citation62]. The apparent discrepancy between the two studies may stem from their difference in the definition of perenniality. Moreover, the higher number of rotational classes in the present study decreases the number of observations per class and the statistical power of the comparisons.
Based on European agroecosystem modeling, an annual SOC increase of 400–800 kg C ha−1 year−1 was found after the conversion of arable land to grassland [Citation20]. In turn, a review of 115 studies from 17 different countries [Citation57] showed a corresponding organic carbon increase rate of 540 kg C ha−1 year−1. Another study [Citation50] reported a SOC increase rate of 490 kg C ha−1 year−1 based on a large French soil database [Citation63]. These values are in line with the results of the present study, showing that a change from annual to perennial grassland rotation leads to SOC restoration at a rate of 193–510 kg C ha−1 year−1, depending on the preceding annual rotation. However, our study showed that changes in SOC content depend greatly on the initial SOC-to-fine-fraction ratio. Thus, even though conversion from annual to grassland rotation has a beneficial impact on SOC balance, it does not necessarily result in SOC sequestration (i.e. an absolute increase in SOC content). Soils with a high level of SOC especially can lose carbon despite the cultivation of perennial grasses, but the rate of decrease is lower than in the case of annual rotations.
According to the national GHG inventory, the total emissions in Finland without the land use, land-use change, and forestry sector were 47.8 Mt CO2 equivalent in 2020. The estimated climatic impact of the crop rotation changes in mineral and organic soils amount to 11,811 Mg C year−1 (80% PI (−6600; 30,300) Mg C year−1), corresponding to approximately 0.1% of total GHG emissions in Finland, with an 80% PI of (−0.05%, +0.23%). In the national GHG inventory, the CO2 emission from cultivated soils has been reported to be 7.7 Mt CO2 equivalent, of which 6.6 and 1.1 Mt results from organic and mineral soils, respectively. In comparison, the contribution of crop rotation changes is about 0.6%. Despite the fact that we had a decade-long high-quality data set, and used state-of-the-art modeling, the uncertainties regarding the effects of individual crop rotations are still large. These uncertainties should be carefully considered when making strategic decisions regarding climate and environmental policy. In particular, basing policy on point estimates from individual studies should be discouraged, and a sufficiently large range of probable values (e.g. an 80% PI from a Bayesian analysis, or the widely used 95% confidence interval from a frequentist analysis) should be considered in the decision-making process. On the other hand, methodological developments are needed to improve the accuracy of the estimates, which would make the decision-making process more straightforward.
The present study focused only on the effect of crop rotations on SOC balance. The study did not consider the climatic impact of possible changes in dietary or livestock feeding, which are closely associated with the choice of cultivated crop plants. Quantification of overall climatic impact would require comprehensive life-cycle assessment, including e.g. emissions of ruminants, transportations, and food processing. Furthermore, it should be noted that changes in crop rotation are only one possible mitigation measure and, particularly in organic soils, raising the water table level (controlled subsurface drainage, paludiculture, or peatland restoration) has proved to be a far more efficient way to reduce the emissions [Citation36,Citation64].
Despite the rather limited contribution of crop-rotation-induced changes on SOC and, further, on national C balance, the diversification of cropping systems may generate many substantial agronomic and environmental benefits, e.g. improved resilience in variable weather conditions, increased biodiversity, higher yields, improved soil water holding capacity, a reduction in pests and diseases, and enhanced water quality [Citation65]. A crop-rotation-induced boost in belowground microbial diversity and activity would support soil functioning by enhancing nutrient cycling and strengthening soil structure formation and structural stability [Citation66]. Furthermore, creating spatial crop diversity via versatile temporal rotations serves to promote aboveground arthropod diversity and density, thus supporting ecosystem resilience [Citation67]. As with water quality, crop rotation diversification can help in reducing nutrient leaching and soil erosion [Citation68], and diverse crop rotations have been shown to reduce nitrogen leaching [Citation69]. Regarding soil erosion, for fixed SOC content, the inclusion of grass in crop rotation has been observed to have only a minor effect on soil aggregate stability compared with cereal monoculture [Citation69]. However, as SOC content has a positive effect on aggregate stability, crop rotations can have a positive influence through an increased SOC level [Citation70].
Conclusions
During the last decade, increased farm size, the warming of the climate, and increased demand for special crops has resulted in a diversification of crop rotations and the adoption of perennial-dominated arable systems in Finland. An increase in the cultivation area designated for perennial grasses was found to have a positive climatic impact through the accumulation of SOC into cultivated mineral soils, and by decreasing the emissions of organic soils. However, a nationwide estimate of the effect of crop rotation on SOC balance proved to be highly uncertain in both mineral and organic soils, drawing attention to the need for methodological development to assess the national-scale climatic impacts. Compared with the total GHG emissions at the national level, the climatic impacts of changes in crop rotations were ultimately relatively small, accounting for approximately 0.1% (80% PI (−0.05%, +0.23%)) of total Finnish GHG emissions. Considering that the large-scale changes in crop rotations which have occurred nationwide have had at most a minute impact on the climate, policy measures aimed solely at increasing carbon in the soil do not appear to be very effective. Instead of large-scale policies focused on soil carbon alone, policies that facilitate targeted site-specific measures with multiple simultaneous goals, including soil productivity, biodiversity, and water body impacts in addition to soil carbon sequestration, might be more justifiable.
Acknowledgements
We thank the Finnish Food Authority for providing data on crop rotation. We also acknowledge the E-OBS dataset from the EU-FP6 project UERRA (http://www.uerra.eu) and the Copernicus Climate Change Service, and the data providers in the ECA&D project (https://www.ecad.eu). We thank the numerous members of Luke’s technical and laboratory staff involved in the soil monitoring network for their contribution to the study.
Disclosure statement
The authors report that there are no competing interests to declare.
Data availability statement
The data that support the findings of this study are available on request from the corresponding author. The data are not publicly available due to privacy or ethical restrictions. The official register of cropping system and farm type used in the sampling site classification can be requested from the Finnish Food Authority: https://www.ruokavirasto.fi/tietoameista/avointieto/tiedonluovutukset.
Additional information
Funding
References
- Köchy M, Hiederer R, Freibauer A. Global distribution of soil organic carbon – part 1: masses and frequency distributions of SOC stocks for the tropics, permafrost regions, wetlands, and the world. SOIL. 2015;1(1):351–365. doi:10.5194/soil-1-351-2015.
- Crowther TW, van den Hoogen J, Wan J, et al. The global soil community and its influence on biogeochemistry. Science. 2019;365(6455):eaav0550. doi:10.1126/science.aav0550.
- Paustian K, Andrén O, Janzen HH, et al. Agricultural soils as a sink to mitigate CO2 emissions. Soil Use Manag. 1997;13(s4):230–244. doi:10.1111/j.1475-2743.1997.tb00594.x.
- Lal R, Bruce JP. The potential of world cropland soils to sequester C and mitigate the greenhouse effect. Environ Sci Policy. 1999;2(2):177–185. doi:10.1016/S1462-9011(99)00012-X.
- Chabbi A, Lehmann J, Ciais P, et al. Aligning agriculture and climate policy. Nat Clim Change. 2017;7(5):307–309. doi:10.1038/nclimate3286.
- Minasny B, Malone BP, McBratney AB, et al. Soil carbon 4 per mille. Geoderma. 2017;292:59–86. doi:10.1016/j.geoderma.2017.01.002.
- de Vries W. Soil carbon 4 per mille: a good initiative but let’s manage not only the soil but also the expectations: comment on Minasny et al. (2017) Geoderma 292: 59–86. Geoderma. 2018;309:111–112. doi:10.1016/j.geoderma.2017.05.023.
- Poulton P, Johnston J, Macdonald A, et al. Major limitations to achieving “4 per 1000” increases in soil organic carbon stock in temperate regions: evidence from long-term experiments at Rothamsted Research, United Kingdom. Glob Chang Biol. 2018;24(6):2563–2584. doi:10.1111/gcb.14066.
- Wiesmeier M, Mayer S, Burmeister J, et al. Feasibility of the 4 per 1000 initiative in Bavaria: a reality check of agricultural soil management and carbon sequestration scenarios. Geoderma. 2020;369:114333. doi:10.1016/j.geoderma.2020.114333.
- Rumpel C, Amiraslani F, Chenu C, et al. The 4p1000 initiative: opportunities, limitations and challenges for implementing soil organic carbon sequestration as a sustainable development strategy. Ambio. 2020;49(1):350–360. doi:10.1007/s13280-019-01165-2.
- Lal R. Soil organic matter and water retention. Agron J. 2020;112(5):3265–3277. doi:10.1002/agj2.20282.
- Baveye PC, Schnee LS, Boivin P, et al. Soil organic matter research and climate change: merely re-storing carbon versus restoring soil functions. Front Environ Sci. 2020;8:579904. doi:10.3389/fenvs.2020.579904.
- Bellamy PH, Loveland PJ, Bradley RI, et al. Carbon losses from all soils across England and Wales 1978–2003. Nature. 2005;437(7056):245–248. doi:10.1038/nature04038.
- Saby NPA, Arrouays D, Antoni V, et al. Changes in soil organic carbon in a mountainous French region, 1990–2004. Soil Use Manag. 2008;24(3):254–262. doi:10.1111/j.1475-2743.2008.00159.x.
- Meersmans J, van Wesemael B, de Ridder F, et al. Changes in organic carbon distribution with depth in agricultural soils in Northern Belgium, 1960–2006. Global Change Biol. 2009;15(11):2739–2750. doi:10.1111/j.1365-2486.2009.01855.x.
- Heikkinen J, Ketoja E, Nuutinen V, et al. Declining trend of carbon in Finnish cropland soils in 1974-2009. Glob Chang Biol. 2013;19(5):1456–1469. doi:10.1111/gcb.12137.
- Taghizadeh-Toosi A, Olesen JE, Kristensen K, et al. Changes in carbon stocks of Danish agricultural mineral soils between 1986 and 2009. Eur J Soil Sci. 2014;65(5):730–740. doi:10.1111/ejss.12169.
- Heikkinen J, Keskinen R, Kostensalo J, et al. Climate change induces carbon loss of arable mineral soils in boreal conditions. Glob Chang Biol. 2022;28(12):3960–3973. doi:10.1111/gcb.16164.
- Merante P, Dibari C, Ferrise R, et al. Adopting soil organic carbon management practices in soils of varying quality: implications and perspectives in Europe. Soil Tillage Res. 2017;165:95–106. doi:10.1016/j.still.2016.08.001.
- Lugato E, Bampa F, Panagos P, et al. Potential carbon sequestration of European arable soils estimated by modelling a comprehensive set of management practices. Glob Chang Biol. 2014;20(11):3557–3567. doi:10.1111/gcb.12551.
- Launay C, Constantin J, Chlebowski F, et al. Estimating the carbon storage potential and greenhouse gas emissions of French arable cropland using high-Resolution modeling. Global Change Biol. 2021;27(8):1645–1661. doi:10.1111/gcb.15512.
- Vertes F, Hatch D, Velthof G, et al. Short-term and cumulative effects of grassland cultivation on nitrogen and carbon cycling in ley-arable rotations In Proceedings of the 14th Symposium of the European Grassland Federation, Ghent, Belgium; 2007. p. 227–246.
- Linsler D, Taube F, Geisseler D, et al. Temporal variations of the distribution of water-stable aggregates, microbial biomass and ergosterol in temperate grassland soils with different cultivation histories. Geoderma. 2015;241-242:221–229. doi:10.1016/j.geoderma.2014.11.013.
- Reinsch T, Loges R, Kluß C, et al. Effect of grassland ploughing and reseeding on CO2 emissions and soil carbon stocks. Agric Ecosyst Environ. 2018;265:374–383. doi:10.1016/j.agee.2018.06.020.
- Guillaume T, Makowski D, Libohova Z, et al. Carbon storage in agricultural topsoils and subsoils is promoted by including temporary grasslands into the crop rotation. Geoderma. 2022;422:115937. doi:10.1016/j.geoderma.2022.115937.
- Chenu C, Angers DA, Barré P, et al. Increasing organic stocks in agricultural soils: knowledge gaps and potential innovations. Soil Till Res. 2019;188:41–52. doi:10.1016/j.still.2018.04.011.
- Johnston AE, Poulton PR, Coleman K, et al. Changes in soil organic matter over 70 years in continuous arable and ley–arable rotations on a sandy loam soil in England. Eur J Soil Sci. 2017;68(3):305–316. doi:10.1111/ejss.12415.
- Poeplau C, Don A, Vesterdal L, et al. Temporal dynamics of soil organic carbon after land-use change in the temperate zone – carbon response functions as a model approach. Global Change Biol. 2011;17(7):2415–2427. doi:10.1111/j.1365-2486.2011.02408.x.
- Or D, Keller T, Schlesinger WH. Natural and managed soil structure: on the fragile scaffolding for soil functioning. Soil Till Res. 2021;208:104912. doi:10.1016/j.still.2020.104912.
- Bolinder MA, Kätterer T, Andrén O, et al. Long-Term soil organic carbon and nitrogen dynamics in forage-based crop rotations in Northern Sweden (63–64°N). Agric Ecosyst Environ. 2010;138(3-4):335–342. doi:10.1016/j.agee.2010.06.009.
- Chan KY, Conyers MK, Li GD, et al. Soil carbon dynamics under different cropping and pasture management in temperate Australia: results of three long-term experiments. Soil Res. 2011;49(4):320–328. doi:10.1071/SR10185.
- Laamrani A, Voroney PR, Berg AA, et al. Temporal change of soil carbon on a long-term experimental site with variable crop rotations and tillage systems. Agronomy. 2020;10(6):840. doi:10.3390/agronomy10060840.
- Franzluebbers AJ, Sawchik J, Taboada MA. Agronomic and environmental impacts of pasture–crop rotations in temperate North and South America. Agric Ecosyst Environ. 2014;190:18–26. doi:10.1016/j.agee.2013.09.017.
- Lilja H, Uusitalo R, Yli-Halla M, et al. Suomen maannostietokanta: Käyttöopas versio 1.1 (Finnish Soil Database: manual, version 1.1). Luonnonvara- ja biotalouden tutkimus; 6. Vol. 114. Helsinki, Finland: Natural Resources Institute Finland; 2017.
- Cornes RC, van der Schrier G, van den Besselaar EJM, et al. An ensemble version of the E-OBS temperature and precipitation data sets. JGR Atmos. 2018;123(17):9391–9409. doi:10.1029/2017JD028200.
- IPCC. 2013 supplement to the 2006 IPCC guidelines for national greenhouse gas inventories: wetlands (Hiraishi T, Krug T, Tanabe K, Srivastava N, Baasansuren J, Fukuda M, Troxler TG, editors.Geneva (Switzerland): IPCC; 2014.
- Elonen P. Particle-size analysis of soil. Acta Agric Fenn. 1971;122:1–122.
- Peltonen-Sainio P, Jauhiainen L, Sorvali J. Diversity of high-Latitude agricultural landscapes and crop rotations: increased, decreased or back and forth? Agric Syst. 2017;154:25–33. doi:10.1016/j.agsy.2017.02.011.
- Plummer M. JAGS: a program for analysis of Bayesian graphical models using Gibbs sampling In Proceedings of the 3rd International Workshop on Distributed Statistical Computing, Vienna, Austria, 2013.
- R Core Team. R: a language and environment for statistical computing. Vienna, Austria: R Foundation for Statistical Computing; 2023.
- Gelman A, Carling JB, Stern HS, et al. Bayesian data analysis. 3rd ed. Boca Raton, FL: Chapman and Hall/CRC; 2013.
- EASAC. Regenerative agriculture in Europe. A critical analysis of contributions to european union farm to fork and biodiversity strategies. EASAC Policy Report 44. 2022. p. 58. Available from: www.easac.eu
- Peltonen-Sainio P, Jauhiainen L, Hannukkala A. Declining rapeseed yields in Finland: how, why and what next? J Agric Sci. 2007;145(6):587–598. doi:10.1017/S0021859607007381.
- Peltonen-Sainio P, Jauhiainen L, Laitinen P, et al. Identifying difficulties in rapeseed root penetration in farmers’ fields in Northern European conditions. Soil Use Manag. 2011;27(2):229–237. doi:10.1111/j.1475-2743.2011.00331.x.
- Peltonen-Sainio P, Jauhiainen L. Large zonal and temporal shifts in crops and cultivars coincide with warmer growing seasons in Finland. Reg Environ Change. 2020;20(3):89. doi:10.1007/s10113-020-01682-x.
- Peltonen-Sainio P, Jauhiainen L. Unexploited potential to diversify monotonous crop sequencing at high latitudes. Agric Syst. 2019;174:73–82. doi:10.1016/j.agsy.2019.04.011.
- Peltonen-Sainio, P.; Jauhiainen, L. Risk of low productivity is dependent on farm characteristics: how to turn poor performance into an advantage. Sustainability. 2019;11(19):5504. doi:10.3390/su11195504.
- McLauchlan KK, Hobbie SE, Post WM. Conversion from agriculture to grassland builds soil organic matter on decadal timescales. Ecol Appl. 2006;16(1):143–153. doi:10.1890/04-1650.
- Persson T, Bergkvist G, Kätterer T. Long-Term effects of crop rotations with and without perennial leys on soil carbon stocks and grain yields of winter wheat. Nutr Cycl Agroecosyst. 2008;81(2):193–202. doi:10.1007/s10705-007-9144-0.
- Soussana J-F, Loiseau P, Vuichard N, et al. Carbon cycling and sequestration opportunities in temperate grasslands. Soil Use Manag. 2004;20(2):219–230. doi:10.1111/j.1475-2743.2004.tb00362.x.
- Anderson-Teixeira KJ, Masters MD, Black CK, et al. Altered belowground carbon cycling following land-use change to perennial bioenergy crops. Ecosystems. 2013;16(3):508–520. doi:10.1007/s10021-012-9628-x.
- Kong AYY, Six J. Tracing root vs. residue carbon into soils from conventional and alternative cropping systems. Soil Sci Soc Am J. 2010;74(4):1201–1210. doi:10.2136/sssaj2009.0346.
- Rasse DP, Rumpel C, Dignac M-F. Is soil carbon mostly root carbon? Mechanisms for a specific stabilisation. Plant Soil. 2005;269(1-2):341–356. doi:10.1007/s11104-004-0907-y.
- Deiss L, Sall A, Demyan MS, et al. Does crop rotation affect soil organic matter stratification in tillage systems? Soil Till Res. 2021;209:104932. doi:10.1016/j.still.2021.104932.
- Post WM, Kwon KC. Soil carbon sequestration and land-use change: processes and potential. Global Change Biol. 2000;6(3):317–327. doi:10.1046/j.1365-2486.2000.00308.x.
- Conant RT, Cerri CEP, Osborne BB, et al. Grassland management impacts on soil carbon stocks: a new synthesis. Ecol Appl. 2017;27(2):662–668. doi:10.1002/eap.1473.
- Conant RT, Paustian K, Elliott ET. Grassland management and conversion into grassland: effects on soil carbon. Ecol Appl. 2001;11(2):343–355. doi:10.1890/1051-0761(2001)011[0343:GMACIG]2.0.CO;2.
- Peltonen-Sainio P, Jauhiainen L, Laurila H, et al. Land use optimization tool for sustainable intensification of high-Latitude agricultural systems. Land Use Policy. 2019;88:104104. doi:10.1016/j.landusepol.2019.104104.
- King AE, Blesh J. Crop rotations for increased soil carbon. Ecol Appl. 2018;28(1):249–261. doi:10.1002/eap.1648.
- Cotrufo MF, Wallenstein MD, Boot CM, et al. The microbial efficiency-matrix stabilization (MEMS) framework integrates plant litter decomposition with soil organic matter stabilization: do labile plant inputs form stable soil organic matter? Glob Chang Biol. 2013;19(4):988–995. doi:10.1111/gcb.12113.
- Kallenbach CM, Grandy AS, Frey SD, et al. Microbial physiology and necromass regulate agricultural soil carbon accumulation. Soil Biol Biochem. 2015;91:279–290. doi:10.1016/j.soilbio.2015.09.005.
- Peltonen-Sainio P, Jauhiainen L, Honkavaara E, et al. Pre-crop values from satellite images for various previous and subsequent crop combinations. Front Plant Sci. 2019;10:462. doi:10.3389/fpls.2019.00462.
- Arrouays D, Deslais W, Badeau V. The carbon content of topsoil and its geographical distribution in France. Soil Use Manag. 2001;17(1):7–11. doi:10.1111/j.1475-2743.2001.tb00002.x.
- Bianchi A, Larmola T, Kekkonen H, et al. Review of greenhouse gas emissions from rewetted agricultural soils. Wetlands. 2021;41(8):1–7. doi:10.1007/s13157-021-01507-5.
- Beillouin D, Ben-Ari T, Malézieux E, et al. Positive but variable effects of crop diversification on biodiversity and ecosystem services. Glob Chang Biol. 2021;27(19):4697–4710. doi:10.1111/gcb.15747.
- McDaniel MD, Grandy AS, Tiemann LK, et al. Crop rotation complexity regulates the decomposition of high and low quality residues. Soil Biol Biochem. 2014;78:243–254. doi:10.1016/j.soilbio.2014.07.027.
- Meyer M, Ott D, Götze P, et al. Crop identity and memory effects on aboveground arthropods in a long-term crop rotation experiment. Ecol Evol. 2019;9(12):7307–7323. doi:10.1002/ece3.5302.
- Hunt ND, Hill JD, Liebman M. Cropping system diversity effects on nutrient discharge, soil erosion, and agronomic performance. Environ Sci Technol. 2019;53(3):1344–1352. doi:10.1021/acs.est.8b02193.
- Beillouin D, Pelzer E, Baranger E, et al. Diversifying cropping sequence reduces nitrogen leaching risks. Field Crops Res. 2021;272:108268. doi:10.1016/j.fcr.2021.108268.
- Soinne H, Hyväluoma J, Ketoja E, et al. Relative importance of organic carbon, land use and moisture conditions for the aggregate stability of post-glacial clay soils. Soil till Res. 2016;158:1–9. doi:10.1016/j.still.2015.10.014.