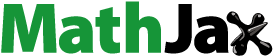
ABSTRACT
Prenatal stress disrupts reproductive function in females and males. These alterations have primarily been related to maternal corticosteroid fetal programming due to the stress response, affecting the fetus and causing long-lasting effects. The aim of this study was to investigate the influence of prenatal stress on male and female fertility. Dams were exposed to stress by immersion in cold water twice a day for the last week of gestation (days 15–21). In the adulthood, sperm quality, mature follicles, sexual hormones and fertility were assessed in female and male progeny. The results in prenatally stressed females showed lower body weight, longer estrous cycles, lower estradiol and progesterone, and lower number of pups. In prenatally stressed males, lower body weight, increased testicular cell death, as well as decreased testosterone levels, sperm quality, and fertility were observed. Aside from these effects, corticosterone levels in prenatally stressed males and females increased. These results show that prenatal stress can markedly influence infertility in adult female and male progeny.
Abbreviations: 3β-HSD: 3β hydroxysteroid dehydrogenase; CRH: corticotropin releasing hormone; DEX: dexamethasone; ERα: estrogen receptor alpha; H-E: hematoxylin-eosine; HPA: hypothalamus-pituitary-adrenal; KISS: Kisspeptin; ORW: ovarian relative weight; PBS: phosphates; PS: prenatally stressed; PRW: prostatic relative weight; ROS: reactive oxygen species; SRW: seminal relative weight; TdT: terminal deoxynucleotidyl transferase; TUNEL: terminal deoxynucleotidyl transferase dUTP Nick-end labelling; TRW: testicular relative weight; URW: uterine relative weight
Introduction
The exposure of pregnant female rats to stressors during the critical period of brain sexual differentiation (gestation days 14–21) can cause intense and long-term postnatal effects in progeny reproduction (Segarra et al. Citation1991). This is known as prenatal programming of the neuroendocrine system regulating reproduction (Evans et al. Citation2016). In males, demasculinization and feminization of sexual behavior has been observed (Ward Citation1972; Weinstock Citation2001; Gerardin et al. Citation2005). The demasculinization of sexual behavior in prenatally stressed males is related to the attenuation of the testosterone peak at gestational days 18 and 19 (Ward et al. Citation2003). Prenatal stress is also correlated with reduced anogenital distance, a decrease in plasma LH, FSH and testosterone levels, as well as delayed testicular descent (Pallarés et al. Citation2013). Also, an increase in the percentage of TUNEL- and active caspase-3 positive cells (apoptotic index) in testis has been observed (Chen et al., Citation2013). Whether the alterations in testicular cells affect sperm quality and male fertility remain to be assessed in prenatally stressed males.
Regarding prenatally stressed females, the number of studies is scarce. They show a decrease in the lordosis quotient and in the intensity of lordosis, as well as fewer solicitation behaviors in standard or paced mating (Frye and Orecki Citation2002). Also, longer estrous cycles (Politch and Herrenkohl Citation1984), failure to become pregnant, spontaneous abortion, and longer pregnancies (Herrenkohl Citation1979) have been reported (for review, see Braastad Citation1998). However, neither sexual hormones nor the number of offspring has been assessed. Therefore, the aim of this study was to evaluate the influence of prenatal stress by immersion in cold water on the sexual hormones, sperm quality, the number of ovarian antral follicles, and fertility in rats. Body and glandular weights were also assessed.
Results and discussion
The experimental design is shown in . Briefly, pregnant females were exposed to stress by immersion in cold water the last week of pregnancy, from day 15 to day 21. Dams were weighed during the pregnancy and corticosterone was assessed on day 18 of pregnancy. Youngs were weighed and sexed at birth; at postnatal day 21, the offspring were weaned, weighed, and separated males from females. Estrous cyclicity was monitored in adult female descendants. Afterward, females in proestrus or diestrus from each group were euthanized; serum corticosterone, estradiol and progesterone were assessed; the number of Graaf follicles were counted in ovaries; uterine epithelial height was measured.
Figure 1. Experimental design. GD: gestational day; ICW: immersion in cold water; PND: postnatal day; C: control group; PS: prenatal stress group; E2: estradiol; P4: progesterone.

In adult male descendants, serum corticosterone and testosterone concentrations, as well as testicular cell death and sperm quality were assessed. Finally, other males and females from CON and PS groups copulated; pregnancy duration and number of descendants were evaluated.
Body weight gain in dams
During the last week of pregnancy, when pregnant females were exposed to stress, body weight gain was lower (3.59 ± 0.27 g/day) compared with control dams, which had an average body weight gain of 5.43 ± 0.23 g/day (t15 = 5.065, p = 0.0002) (). Even though the quantity of food intake was not assessed, body weight gain was reduced in stressed dams compared with control dams. The correlation coefficient (r2) for control curve was 0.9842, and ANOVA for prediction was 13.16, p = 0.008. The correlation coefficient for PS curve was 0.9435, and ANOVA for prediction was 64.92, p = 0.0001. Low body weight gain can be explained by Hypothalamus-Pituitary-Adrenal (HPA) axis activation by stress, interfering with body weight gain despite having free access to food. Corticotropin Releasing Hormone (CRH) has been proposed to be responsible for this effect, since it is also a major anorexigenic peptide, suppressing appetite and feeding behavior in stressed subjects (Charmandari et al. Citation2005).
Figure 2. Daily body weight gain in control and stressed dams. Stressed dams gained less body weight from gestational day 15 to delivery (day 23). The regression lines of daily body weight for control and stressed dams are shown. Correlation coefficient for control curve: 0.9842; ANOVA for prediction: 13.16, p = 0.008. Correlation coefficient for PS curve: 0.9435; ANOVA for prediction: 64.92, p = 0.0001. The slopes indicate the average increase in body weight per day, and that of the stressed dams were significantly lower than that of control dams (p = 0.01), which. N = 10 per group.

Corticosterone levels in dams
Stressed dams showed significantly higher serum levels of corticosterone (555.18 ± 72.36 ng/mL) than control dams (214.4 ± 11.68 ng/mL) at day 18 of pregnancy [t8 = 10.39, p = 0.003]. These results corroborate the increase of maternal glucocorticoid during stress, as has been reported previously, with a slight increase in corticosterone of pregnant stressed mothers (Ward and Weisz Citation1984). In this study, corticosterone levels in stressed dams were almost three orders of magnitude higher than those of control dams. The differences with the study of Ward and Weisz could be explained by the intensity of the stressor used: restraint plus illumination vs immersion in cold water in this study, which is a stressor that elicits stronger HPA axis response (Retana-Márquez et al. Citation2003).
Number of offspring
The number of female pups from control dams (48/95; 50%) was similar to the number of female pups from stressed (53/97; 55%) dams. In the same way, there were no differences between the number of male pups from control (47/95; 49%) and male pups from stressed (46/97; 47%) dams.
Offspring body weight
PS females showed significant lower body weight than control females at all ages evaluated: birth (PS = 5.44 ± 0.25 vs CON = 6.26 ± 0.43), weaning (PS = 29.18 ± 1.52 vs CON = 32.07 ± 1.06) and adulthood (PS = 217.95 ± 15.52 vs CON = 249.78 ± 19.37), with the most marked differences at adulthood (p = 0.01). Similarly, body weight in PS males was lower at birth (PS = 5.85 ± 0.36 vs CON = 6.92 ± 0.92), weaning (PS = 33.69 ± 1.03 vs CON = 35.14 ± 1.26), and adulthood (PS = 341.90 ± 16.82 vs CON = 369.47 ± 21.54) compared to control males (p = 0.01). These results are different from other studies in which no effect of prenatal stress on birth weight was reported (Weinstock Citation2008; Pallarés et al. Citation2013). Only with severe prenatal stress, reduced birth weight has been reported (Kinsley and Svare Citation1986). Therefore, the stressor used in this work is severe enough to cause lower body weight in the offspring during life. Low birth weight has been explained by decreased maternal food intake in response to gestational stress and increased CRH. This might lead to intrauterine growth retardation due to decreased nutrient transport from mother to fetus, which has been attributed to high glucocorticoid levels in dams. Glucocorticoids cause a decrease in placental glucose transporter GLUT1 expression, leading to decreased glucose transfer to the fetus (Viltart and Vanbesien-Mailliot Citation2007). Lower body weight at weaning and adult ages in PS subjects may indicate offspring’s lower food intake, possibly due to alterations in the hormones controlling food intake, such as CRH, leptin and others. This hypothesis is supported by the finding that the administration of dexamethasone (DEX) in the last third of pregnancy causes hyperleptinemia in adult descendants, and it is well known that leptin inhibits food intake in adults (Sugden et al. Citation2001; Viltart and Vanbesien-Mailliot Citation2007).
Female descendant
Estrous cyclicity and female hormones
The evaluation of vaginal smears showed treatment-dependent changes in estrous cycles. Control rats showed normal estrous cycles, presenting all stages in normal progression. Prenatal stress caused irregular estrous cycles in adult female progeny, with an increase in the number of days in estrus and proestrus, thus enlarging estrous cycles, or causing incomplete cycles. Representative estrous cycles of females from the two groups are shown in . The percentage of females with abnormal estrous cycles in control females was 20%, while in PS females the percentage of females with abnormal cycles was 90% (p = 0.0001). The most common abnormalities observed were continuous proestrus or estrus, or even no diestrus, for several consecutive days in most of PS females. These results coincide with those reported by other authors (Herrenkohl and Politch Citation1978; Politch and Herrenkohl Citation1984). Regarding sexual hormones, serum levels of estradiol in PS females during proestrus and diestrus were lower than those observed in control females in the same stage (p = 0.01) (Supplementary Figure 1A). The alterations in estrous cyclicity may be explained by decreased but continuous estradiol observed in these animals, considering that the vaginal epithelium is responsive to this sex steroid. Decreased estradiol levels during proestrus can lead to lack of positive feedback at hypothalamic level, causing alterations in the endocrine events involved in ovulation induction. This remains to be confirmed by evaluating GnRH and Kisspeptin (KISS). Even though LH was not evaluated in this study, higher levels of this gonadotropin, associated to decreased estradiol levels, have been reported in prenatally stressed females, which indicates that prenatal stress causes hypoestrogenism leading to severe neuroendocrine dysfunctions (Ordyan et al. Citation2013).
Figure 3. Representative estrous cycles of control and prenatally stressed (PS) females during adulthood for three consecutive weeks. PS caused enlarged cycles with absence of diestrus periods, as well as an increase of days in estrus or proestrus. Estrus (E), Metestrus (M), Diestrus (D), Proestrus (P).

Low serum progesterone during proestrus and diestrus was also observed in PS females compared with control females (p = 0.003) (Supplementary Figure 1B). This can be due to low levels of gonadotropin causing fewer mature follicles as well as low number of ovulated oocytes and, therefore, corpora lutea. Low levels of progesterone can be explained by low number of corpora lutea, which might indicate low ovulation rates.
Female reproductive organs
ORW in PS females was lower than in control females in proestrus (p = 0.0007), but not in diestrus. Also, ORW during proestrus was different than during diestrus in control females, this was not observed in PS females. The number of preovulatory antral follicles in ovaries from PS females in proestrus was significantly lower than the number of preovulatory antral follicles in the ovaries from control females () in the same stage (p = 0.04). The number of corpora lutea was also smaller in ovaries from PS females during diestrus compared with control females (p = 0.02) (). The lower number of mature follicles in PS females’ ovaries could be related with the lower levels in ovarian hormones at this stage of estrous cycle, as discussed above. Also, lower levels of progesterone during diestrus might reflect a low ovulation rate with decreased function in corpora lutea. In this work, the number of primordial, primary and secondary follicles were not assessed. This study focused on the antral preovulatory follicles, in order to analyze female fertility. Despite this, the results of this work agree with a previous study in which a lower number of healthy primordial, primary, secondary, and antral preovulatory follicles were found in the ovaries of prenatally stressed females at puberty, as well as higher number of apoptotic follicles. Those results were attributed to lower response of follicles to FSH, reflected in lower cAMP (Barra et al. Citation2014).
Figure 4. Photomicrographs of ovaries from control (A) and prenatally stressed females (B). Ovaries from prenatal stressed females (PS) showed few mature follicles compared with control ovaries. Uterine epithelia from control (C) and PS females (D). Epithelial height was lower in PS compared with control uteri. Magnification: 40× for ovaries and 1000× for uteri. (e) Ovarian relative weight (ORW) and uterine relative weight (URW). Number of mature follicles and corpora lutea observed in ovaries of control and PS female rats in proestrus and diestrus stages. Data shown as Means ± SDM. Different letters indicate significant differences with the respective control, p = 0.05.

Regarding uterine relative weight (URW), it was lower in PS females compared with controls during proestrus (p = 0.045). No differences were observed in URW between groups during diestrus. URW in proestrus differed from diestrus in control but not in PS females (). Additionally, uterine epithelium in control female in proestrus was higher than in PS females during proestrus (), but not in diestrus (p = 0.02). These effects on uterine epithelium during proestrus are related with the low estradiol levels observed in this stage in PS females. It is known that uterine epithelial growth depends on estradiol through estrogen receptor alpha (ERα) (O´Brien et al., Citation2006; Winuthayanon et al. Citation2014). More studies are needed in order to evaluate content and expression of ER and PR in uterus from PS females. All of these effects can also be explained by the high levels of corticosterone (stress hormone) observed in PS females compared with control females (p = 0.0005) (Supplementary Figure 1C). This glucocorticoid can decrease gonadotropin release in the pituitary through reduce Kisspeptin releasing (Luo et al. Citation2016), leading to decreased synthesis of ovarian estradiol (Michael et al. Citation1993; Whirledge and Cidlowski Citation2010). The high levels of corticosterone observed in adult PS females coincide with earlier studies (Maccari et al. Citation2003) and it has been shown that the high levels of maternal glucocorticoid during stress are passed on to the fetus due to reduced expression and activity in placental 11β-HSD2, leading to a decrease in hippocampal GR and MR, which causes a deficit in negative feedback by glucocorticoids, both in basal (hyper-activity) and stress-induced (hyper-reactivity) conditions (Weinstock et al. Citation1992; Maccari et al. Citation2003; Viltart and Vanbesien-Mailliot Citation2007).
Male progeny
Reproductive organs and TUNEL-positive testicular cells
Testicular relative weight (TRW) was significantly lower in adult PS males (41.35 ± 4.03) compared with controls (51.23 ± 4.76, p = 0.004). No significant differences were observed in the prostatic relative weight (PRW, PS = 18.17 ± 4.73 vs CON = 18.09 ± 3.55; p = 0.8) and seminal relative weight (SRW, PS = 45.73 ± 7.04 vs CON = 42.20 ± 7.32; p = 0.1). The low testicular weight observed in PS males could be explained by decreased spermatogenesis due to a higher apoptosis index in germinal cells observed in this study (). The percentage of seminiferous tubules with TUNEL-positive germ cell was significantly higher in testes from PS males when compared with control group (p = 0.001). Also, the number of TUNEL-positive germ cells per seminiferous tubule was higher than in the testes from control males (p = 0.05) (). Primary spermatocytes were the main type of TUNEL-positive germ cells (). These results coincide with those reported in other studies in which an increased apoptotic index has been found in adult PS males (Chen Cárdenas et al. Citation2013). Decreased spermatogenesis in PS males, with severe damage to the seminiferous epithelium has also been reported (Chehreie et al. Citation2013). Interestingly, the number of TUNEL-positive Leydig cells was higher in testes from PS males (p = 0.05), (). This finding is in agreement with the reports from other authors who found a low number of Leydig cells in the testes of PS males (Pallarés et al. Citation2013). The increased germinal cell death in the testes of PS males can be attributed to decreased levels of testosterone, which in turn is explained by decreased number of Leydig cells observed in these males. Serum testosterone from adult PS males was significantly lower (1.35 ± 0.53 ng/mL) than in control males (3.64 ± 1.13 ng/mL; p = 0.0001). Our results are similar to those reported by other authors who described inhibition of the testicular axis due to PS, with lower testicular weights, testosterone and LH levels (Harvey and Chevins Citation1984; Kinsley et al. Citation1992; Shono and Suita Citation2003; Rodríguez et al. Citation2007; Chen Cárdenas et al. Citation2013; Pallarés et al. Citation2013). Lower levels of testosterone can be due to the high number of TUNEL-positive Leydig cells. Death in Leydig cells can be explained by the high levels of corticosterone observed in PS males (329.03 ± 82.91 ng/mL) compared with controls (183.45 ± 50.55 ng/mL; p = 0.0001). It has been reported that glucocorticoids such as corticosterone are capable of reducing the release of LH from the pituitary (Almeida et al. Citation1998) and steroidogenesis in Leydig cells, by inhibiting the transcription of genes encoding steroidogenic enzymes (Orr et al. Citation1994). This has been demonstrated by the prenatal administration of the synthetic glucocorticoid dexamethasone, which causes decreases steroidogenic enzyme 3β hydroxysteroid dehydrogenase (3β-HSD) reducing testosterone in embryonic testis (Yun et al. Citation2016). Furthermore, high levels of corticosterone can also initiate apoptosis in rat Leydig cells (Hardy et al. Citation2005).
Figure 5. TUNEL-positive cells in testicular sections from control and prenatally stressed (PS) males. (A) and (C): control testes; (B) and (D): seminiferous tubules from a PS male. The number of TUNEL-positive cells (arrows) observed in seminiferous epithelium were bigger in PS than in control males. (A) and (B): confocal microscopy; (C) and (D): light microscopy microphotographs showing TUNEL-positive cells, mainly Spermatocytes (arrows). Magnification in panels (A) and (B): 100×; magnification in (C) and (D): 1000×. (E) Percentage of seminiferous tubules with TUNEL-positive testicular germ and Leydig cells in control and prenatally stressed males. Data shown as Means ± SDM. Chi-square test for percentages; student’s t test for numbers. Different letters indicate differences from control group, × = 0.05.

Sperm analysis
Epididymal sperm parameter quality was significantly lower in PS males compared with control males. Total motility in PS males was: 58.59% ± 5.12 vs CON = 81.65% ± 5.50 (p = 0.05); viability in PS = 62.22% ± 4.69 vs CON = 84.86% ± 5.45 (p = 0.05) and total sperm count in PS = 101.48 × 106 ± 15.25 vs CON = 141.82 × 106 ± 23.46 (p = 0.003) Aside from these values, the percentage of abnormal sperm was higher in PS subjects (15.63 ± 8.36) when compared with controls (2.95 ± 1.77; p = 0.01). Abnormalities consisted of different patterns of abnormal heads and abnormal tails (Supplementary Figure 2). To our knowledge, this is the first report on sperm quality in PS males, which is critical for male fertility. Epididymis is an organ in which sperm maturation occurs, consisting of changes in motility, metabolism, morphology, biochemical properties and the development of the ability to fertilize oocyte. This process is androgen dependent (Robaire et al. Citation2006); therefore, the decrease in testosterone observed in this work may explain low sperm motility and viability, since in the androgen-deprived state, spermatozoa become immotile, lose the ability to fertilize, and expire (Dyson and Orgebin-Crist Citation1973). The low sperm count can be due to increased testicular germ cell death, as observed in this work, possibly caused by decreased testosterone, since this androgen is essential for spermatogenesis. High corticosterone levels observed in PS males can also be involved in low sperm quality, altering the epididymal microenvironment by inducing reactive oxygen species (ROS) overproduction and lipid peroxidation (Dhanabalan et al. Citation2010). Lipid peroxidation can cause a defect in the mid piece decreasing motility due to axoneme defects and ATP depletion (Agarwal et al. Citation2014), leading to low sperm viability and, consequently, low fertility.
Fertility
Mating between control females and control males was successful in 100%, as control males impregnated all the control females. Up to 70% of the control females that mated with PS males were impregnated. The percentage of PS females copulating with control males reached about 67% with only 50% of PS females that mated with PS males achieving pregnancy (p = 0.001) (). The average number from control females/control male offspring was 11. The number of pups from the other mating groups was significantly lower than controls, 7 in average (p = 0.0024) (). All the reproductive alterations observed in PS females might explain their lower fertility, as indicated by the lower percentage of pregnant PS females when they copulated with PS males, as well as the lower number of pups at birth. These results may be due to decreased number of mature follicles and ovulation rate in PS females. However, this remains to be demonstrated. The results of this work differ from other reports in which no differences in litter size were observed (Herrenkohl Citation1979). This difference may be due to the type of stressor used in this study, which is more intense than heat, restraint and bright light used in that study. In addition to decreasing the number of mature follicles, lower uterine epithelial height could also contribute to low embryo implantation and low number of offspring in PS females. The lower fertility observed in PS males when copulating with control and PS females might be explained by low sperm quality. However, more studies are necessary to evaluate possible alterations in sperm acrosome and fertilizing potential.
Figure 6. Percentages of pregnant control (C) and prenatally stressed (PS) females (A). Lower percentages of pregnancy were obtained in control and PS females after copulating with PS males. X2 followed by Fisher exact probability test. (B): the number of offspring born from control or PS mothers copulating with PS males was significantly lower. Two-way ANOVA followed by Newman-Keuls. Data shown as Mean ± S.D.M. *p = 0.05 compared with C-C group.

There is evidence that prenatal exposure to some disturbing events may affect fertility in humans (Lumey Citation1998). Recently, it has been reported that maternal stress due to bereavement during the first trimester is an infertility risk factor in daughters (Plana-Ripoll et al. Citation2016) and congenital anomalies of genital organs (hypospadias, cryptorchidism, and testicular cancer) in sons (Plana-Ripoll et al. Citation2017). However, the evidence of the effects of prenatal stress in humans is limited, and the study of long-term effects of prenatal stress is difficult. Despite the stress in humans is far different than the stress in animals, the results of this work suggest possible effects of prenatal stress on human reproduction, considering the increase of medically assisted reproduction in couples experiencing infertility in several countries.
The results of this work show that prenatal stress disrupts female and male progeny, decreasing sex hormones, the number of Graaf follicles in females, as well as testicular cell death and low sperm quality in males. All lead to infertility and decreased number of offspring. These alterations are likely related to hyperactivity of the adrenal axis.
Material and methods
Ethical declaration
Animal management and experiments were carried out in accordance with Mexican official regulations (NOM-062-ZOO-1999), and the domestic and laboratory animal regulation published in the Ethical Conduct Guidelines for Research, Teaching and Outreach of the Health and Biological Sciences Division, Universidad Autónoma Metropolitana (2010). This study was approved by Ethics Committee of Health and Biological Sciences, Universidad Autónoma Metropolitana.
Animals
Three-month old female Wistar rats, weighing 250 g (n = 20) were obtained from the vivarium of the Universidad Autónoma Metropolitana Iztapalapa. Animals were maintained under a reversed light-dark cycle (12:12, lights off at 09:00), room temperature (23 ± 1°C), and access to food and water ad libitum.
Adult females copulated with sexually experienced males, who were allowed to ejaculate twice in order to impregnate females. The presence of sperm in vagina was confirmed, and that day was considered as day 0 of pregnancy. Pregnant females were housed in Plexiglass individual cages throughout gestation and were assigned to one of two groups: Control (CON, n = 10) and maternal stress (PS, n = 10).
The experimental design is shown in .
Prenatal stress procedure
Pregnant females of the stress group were transferred to another room, where they were exposed to stress by immersion in cold water during the last gestational week (days 15–21). The order of cages being taken to the stressor area was random day to day. Rats were placed individually in a covered tank with cold water (depth = 15.5 cm; temperature = 15°C). Rats remained in an upright position and keeping their head above water level (Retana-Márquez et al. Citation2003). The stressor was applied twice a day, at 9:00 and 15:00 h, for 15 min each time. At the end of this time, rats were picked up from the tank and towel dried. In order to evaluate daily body weight gain, dams were weighed every day during pregnancy. Additionally, five control dams and five stressed dams were euthanized at day 18 of pregnancy in order to examine fetal resorption and blood samples were obtained for corticosterone evaluation.
Dams of control group were kept undisturbed in their cages
After delivery, the offspring were sexed, weighed and the number of young in the litters was homogenized within their respective groups. Pups remained with their mother until weaning (day 21 of postnatal life), when they were weighed, sex confirmed and separated males from females. Two animals from each litter were included in the experimental groups. At 3 months of age, reproductive variables were assessed in female and male descendants (control groups n = 20 females, n = 20 males; PS n = 20 females, n = 20 males).
Reproductive measures in female descendants
Vaginal cytology and estrous cyclicity
Estrous cycles in female descendants (n = 10, each group) were monitored by daily vaginal smears, which were taken every day for 3 weeks, stained with hematoxylin-eosin and evaluated with a light microscope (Olympus, model CX41RF) at 400× magnification. Vaginal smears were obtained one hour before the onset of the dark period. Estrous cycles were considered normal when full estrus, metestrus, diestrus, and proestrus periods were observed during 4-day or 5-day cycles. Estrous cycles were considered irregular when (a) shortened condensed or absent diestrus period (3-day cycle); (b) two or more consecutive days of proestrus or estrus periods were observed (Henry and Witt Citation2002).
Biological samples
After the three weeks of estrous cycle monitoring, control and PS females (n = 10, each group) were rapidly euthanized by decapitation at PND 111 and trunk blood was collected (2–3 mL). Blood samples and sexual organs were obtained from females in proestrus (n = 5) and diestrus (n = 5), in order to obtain data from both stages of the estrous cycle.
Ovarian and uterine weights
Ovaries and uteri were excised; organs were weighed after connective tissue and fat were removed. Ovarian and uterine relative weights (ORW and URW, respectively) were calculated according to the following formulations:
Morphologic analysis
Ovaries and uteri from females of each group were excised and embedded in 4% formaldehyde with 0.1 M buffer of phosphates (PBS), at pH 7.4 for 24 h. Tissues were then dehydrated and embedded in paraffin. Serial sections (5 μm thick, each) were obtained from whole ovaries. Also, transverse serial sections from the upper, middle and lower portion of uteri were obtained from each animal.
The sections were mounted and stained with hematoxylin-eosine (H-E) and observed with an optic microscope (Olympus, model CX41RF) at a 40x magnification. Photomicrographs were taken on a Leica d-lux3 camera (10.9 Megapixel), 1px = 0.75pt, for morphometric analyses. Morphological and morphometric analyses were done using AmScope 3.7.7934 software, Version ×64 for Windows 10 (AmScope, Irvine CA, USA), calibration 4× for ovaries and 40× for uteri. Ten photographs were taken per organ.
Morphological analysis of ovary sections
According to the study of Myers et al. (Citation2004), follicular stages were identified as primordial follicles (an oocyte surrounded by a layer of flattened granulosa cells); primary follicles (an oocyte surrounded by cuboidal granulosa cells); secondary follicles (oocyte surrounded by more than one layer of cuboidal granulosa cells, without antrum); early antral follicles (with emerging antral spaces); antral follicles (with a defined antral space); preovulatory follicles (the largest follicles, with a defined cumulus granulosa cell layer). In the proestrus, follicles develop rapidly until ovulation at the end of proestrus (Sato et al. Citation2016). Therefore, the number of large preovulatory follicles were observed and counted in ovarian sections in proestrus.
During diestrus stage, ovary presents large corpora lutea newly formed from the previous ovulation with maximal size, which is the best marker for diestrus. Degenerate corpora luteum are also present. At the end of the cycle, corpora lutea with fibrous tissue are present (Westwood Citation2008). Only large, newly formed corpora lutea were counted, corpora lutea with fibrous tissue were not counted, because regressive corpora lutea persist for 12–14 days; therefore, ovary rodents tend to present many corpora lutea (Sato et al. Citation2016).
During proestrus, uterine epithelium is characterized by tall columnar cells (Westwood Citation2008), and these changes are induced by estradiol (Sato et al. Citation2016). During diestrus, the uterus is small, with no activity and with a low cuboidal epithelium (Westwood Citation2008). According to this, epithelial height of endometrium was measured from each female in proestrus and diestrus, from the upper, middle and lower portion of uteri. Uterine epithelial cell heights were measured by drawing lines from the basement membrane to the apical end of cells. Ten points per uterine section per animal were measured, according to Winuthayanon et al. (Citation2014).
Hormonal analysis
In order to minimize pre-analytical variability upon the results of immunoassay, the procedures of blood collection, coagulation, centrifugation, storage, and analysis after sampling were looked after (Bielohuby et al. Citation2012) Blood samples were allowed to coagulate for 30 min at room temperature, and then centrifuged at 2000 × g, at 4°C, for 15 min to separate the serum. Serum samples were stored at −20°C for three days until estradiol, progesterone, testosterone, and corticosterone determination by ELISA. All analyses were done the same day, in order to avoid several freeze-thawing cycles. Commercial kits (DRG Instruments GmbH, Germany) were used for each hormone determination according to the manufacturer’s specifications: Corticosterone (EIA-1887), Estradiol (EIA-2693), Progesterone (EIA-1561), and Testosterone (EIA-1559). Each run included a standard curve. The absorbance of each well was assessed at 420 nm with a microtiter plate reader (Model EPOCH ELx50, BioTek Instruments, Winoosky Vermont, USA). Tihe limit of detection for estradiol was 0.10 pg/mL, for progesterone 0.1 ng/mL, for testosterone 0.49 ng/mL, and for corticosterone 5.13 ng/mL. Method validation included intra- and interday precision. C.V.s for intra- and interday precision for testosterone were 1.93% and 2.12%, respectively. C.V.s. for intra- and interassay for estradiol were 2.36% and 2.67%, respectively. C.V.s. for intra- and interassay for progesterone were 1.2% and 1.59%, respectively. C.V.s for intra- and inter-day precision for corticosterone were 1.23% and 1.93%, respectively.
Reproductive measures in male descendants
Body and glandular weights
At the end of sexual testing and recording body weights, 10 males from each group were rapidly euthanized by decapitation. Blood was collected, allowed to coagulate for 30 min at room temperature, and then centrifuged at 2000 × g, at 4°C, for 15 min to separate the serum. Testes, prostate, and seminal glands of every male were excised and weighed.
Testes, prostate and seminal glands were excised and weighed after connective tissue and fat were removed. Testicular relative weight (TRW), prostatic relative weight (TRW), and seminal relative weight (SRW) were calculated according to the following formulations:
Testicular tissue sampling
Testes were dissected and weighed without the epididymis, washed with 0.9% saline solution. Paraformaldehyde (4%, Sigma-Aldrich, USA, purity 95%) was perfused (volume 0.5 mL, flux 0.1 mL/s) through testicular artery. Testes were processed and paraffin embedded (Paraplast-plus, McCormick Scientific, USA). Transverse non-serial sections (5 μm) from the middle region of testes of each animal were cut from blocks using a microtome (Leica, Vienna, Austria) and mounted on poly-L-lysine hydrobromide (high purity grade, Sigma Aldrich, USA) treated slides. Four sections per animal were obtained.
Terminal deoxynucleotidyl transferase (TdT) dUTP Nick-end labeling (TUNEL) assay
This assay detects cells undergoing extensive DNA degradation in late stages of cell death. Blunt ends of double-stranded DNA breaks are labeled with TdT without a template (Kyrylkova et al. Citation2012). The In-Situ Cell Death Detection kit (POD kit, Cat. No. 11684817910 Roche, Germany) was used according to manufacturer’s instructions. Testis tissue sections were dewaxed in xylene and rehydrated in progressive ethanol dilutions: 100, 96, 80 and 70%, washed and maintained in PBS (137 mM NaCl, 2.68 mM KCl, 8.03 mM Na2HPO4, 1.47 mMK2HPO4, pH 7.4). Tissue sections were treated with Proteinase K (Proteinase K recombinant PCR Grade, Cat. No. 03 115 887 001 Roche, Germany, 20 μg/ml) for 30 min at 37°C in a dark humidity chamber. Permeabilization was achieved with 0.1% Triton X-100 in 0.1% sodium citrate for 5 min on ice (4°C); sections were incubated with the TUNEL reaction mixture TdT (25 μl), and fluorescein-dUTP for 60 min at 37°C in a dark humidity chamber. At least 100 seminiferous tubules were randomly analyzed per slide in different fields. TUNEL-positive germinal cells (showing intense green nuclear fluorescence) were first identified and counted in an epifluorescence microscope (Axiostar Plus, HBO 50/Ac, Carl Zeiss). The percentage of tubules with TUNEL-positive germ cells and the number of TUNEL-positive cells contained in 100 tubules randomly selected were counted from five subjects of each group. The total number of TUNEL-positive Leydig cells (showing green epifluorescence) among seminiferous tubules were identified and counted per slide in different fields using an epifluorescence microscope.
After counting the number of TUNEL-positive cells with an epifluorescence. microscope, tissues were covered with 50 µl of converter (Converter POD- HRP) and were incubated at 37°C during 30 min into a dark humidified chamber. Then, washed twice with PBS. Afterward, tissues were treated with 50 µl of DAB (3–3´Diaminobenzidine tetrahydrochloride) at 0.05%, during 1 min at room temperature. Subsequently, slides were washed three times with PBS. Then, tissues were counter-stained with hematoxilin and covered with a solution of glicerol/PBS (1:1), a slide was put and let dry at room temperature. Seminiferous tubules were analyzed with a light microscope (Olympus Light Microscope CX 41), at magnification 400x in order to identify germ cells TUNEL-positive, which presented an intense brown stain (Yazawa y cols., Citation1999). This procedure allowed to identify what kind of testicular cells were TUNEL-positive. The number of TUNEL-positive Leydig cells were identified and counted. The average field size of measurement was 1.0 mm2 for each section.
Sperm analysis
The right cauda epididymis was deposited in 1 ml of PBS at 37°C. Cauda epididymis was cut using fine tip dissection scissors to release the sperm stored.
Sperm viability
Sperm viability was assessed with one-step eosin–nigrosine (5% nigrosin, 1% eosin and sodium citrate dissolved in distilled water) staining technique (Juárez-Rojas et al. Citation2015). Ten microliters of sperm suspension were mixed with the colorant solution (10 μl) and analyzed on a pre-warmed slide. Slides were observed under an optic microscope (Olympus Light Microscope CX 41) at 400 x magnification. Two hundred sperm were counted in each slide. Unstained sperm were considered as viable whereas stained sperm were considered as dead. Different fields were analyzed randomly until two hundred sperm were counted. Sperm viability was reported as the viable spermatozoa percentage out of the total count.
Sperm motility
Ten microliters of sperm suspension were analyzed on a pre-warmed slide. Two hundred sperm were counted in each slide. Total sperm motility (progressive and non-progressive motility) was assessed from 200 sperm per slide and expressed as percentage of motile sperm of the total sperm counted. Sperm were observed under an optic microscope (Olympus Light Microscope CX 41) at 400× magnification.
Sperm count
Epididymal sperm count was performed using a Neubauer chamber. A sample of sperm suspension (25 μl) was diluted in distilled water to a final volume of 500 μl. Diluted sperm suspension (10 μl) was placed on the Neubauer chamber and counted on eight different spots. Count discrimination criteria were set using the spermatozoa head position inside the chamber squares that were being analyzed. Sperm concentration was obtained according to the following formula: sperm count × # chamber squares (8) × dilution factor (21) × 10,000/2. Final sperm count was expressed in millions per milliliter.
Sperm with morphological abnormalities in head and flagellum were counted and expressed as the percentage of a total of 500 sperm counted.
Fertility
Other males (n = 10) and females (n = 10) from CON and PS groups copulated each other, as shown in . Males were allowed to ejaculate twice in order to impregnate females. Sperm was identified in vagina and that day was considered as day 0 of pregnancy. Pregnancy duration and offspring was evaluated.
Statistical analyses
Corticosterone in control and stressed dams at pregnancy day 18, the number of follicles, uterine epithelial height at proestrus, and male relative organ weight were analyzed by Student’s t Test. Body weight at different ages was analyzed by repeated measures two-way ANOVA, with condition and ages as factors, followed by Newman-Keuls post hoc test; Female relative organ weight and hormone concentrations in females during proestrus and diestrus were analyzed by two-way ANOVA, with condition and stage as factors, followed by Newman-Keuls post hoc test. Percentage of females with abnormal estrous cycles, tubules with apoptotic cells, sperm parameters and pregnant females were analyzed by Chi-square. The number of young was analyzed by one-way ANOVA. Differences were considered significant when p < 0.05. All analyses were performed with GB-STATTM for Windows (Dynamic Microsystems, Inc., Silver Spring, MD, USA).
Supplemental Material
Download Zip (123.4 KB)Acknowledgments
The authors want to express their gratitude to Mrs. EdithMonroy for her advice in English language, and Dr. Mario García Lorenzana for his support in the processing of biological samples for histologic analysis
Disclosure statement
No potential conflict of interest was reported by the authors.
Supplementary material
Supplemental data for this article can be accessed here.
Additional information
Notes on contributors
Socorro Retana-Márquez
Carried out estrous cycle assessment, histology, TUNEL and drafted the manuscript: GVD; Carried out the sperm analysis, TUNEL and participated in the critical revision of the manuscript: JRL; Carried out hormonal evaluation and participated in the critical revision of the manuscript: RMS; Conceived, designed and coordinated the study, statistical analysis, revised and corrected manuscript, participated in the critical revision of the manuscript: R-MS. All authors read and approved the final version of the manuscript.
References
- Agarwal A, Virk G, Ong C, du Plessis SS. 2014. Effect of oxidative stress on male reproduction. World J Mens Health. 32:1–17.
- Almeida SA, Petenusci SO, Anselmo-Franci JA, Rosa-e-Silva AA, Lamano- Carvalho TL. 1998. Decreased espermatogenesis and androgenic testicular functions in adult submitted to immobilization-induced stress from puberty. J Med Biol Res. 31:1443–1448.
- Barra R, Cruz G, Mayerhofer A, Paredes A, Lara HE. 2014. Maternal sympathetic stress impairs follicular development and puberty of the offspring. Reproduction. 148:137–145.
- Bielohuby M, Popp S, Bidlingmaier M. 2012. A guide for measurement of circulating metabolic hormones in rodents: pitfalls during the pre-analytical phase. Mol Metab. 1:47–60.
- Braastad BO. 1998. Effects of prenatal stress on behaviour of offspring of laboratory and farmed mammals. Appl Anim Behav Sci. 61:159–180.
- Charmandari E, Tsigos C, Chrousos G. 2005. Endocrinology of the stress response. Annu Rev Physiol. 67:259–284.
- Chehreie S, Rabzia A, Farhadi-Mesterkhani. M. 2013. Maternal water deprivation affects the spermatogenesis of the offspring rats. Int J Morphol. 31:156–161.
- Chen Cárdenas SM, Mayer N, Romanini MC, Rolando AN, Liaudat AC, Brun N, Vivas A, Gauna HF, Rodríguez N. 2013. Reproductive response in offspring male rats exposed to prenatal stress and to early postnatal stimulation. Int J Morphol. 31:754–764.
- Dhanabalan S, Jubendradass R, Latha P, Mathur PP. 2010. Effect of restraint stress on 2,3,7,8 tetrachlorodibenzo-p-dioxin induced testicular and epididymal toxicity in rats. Hum Exp Toxicol. 30:567–578.
- Dyson ALMB, Orgebin-Crist M-C. 1973. Effect of hypophysectomy, castration and androgen replacement upon the fertilizing ability of rat epididymal spermatozoa. Endocrinology. 93:391–402.
- Evans NP, Bellingham M, Robinson JE. 2016. Prenatal programming of neuroendocrine reproductive function. Theriogenology. 86:340–348.
- Frye CA, Orecki SA. 2002. Prenatal stress alters reproductive responses of rats in behavioral estrus and paced mating of hormone-primed rats. Horm Behav. 42:472–483.
- Gerardin DCC, Pereira OCM, Kempinas WG, Florioc JC, Moreira EG, Bernardi MM. 2005. Sexual behavior, neuroendocrine, and neurochemical aspects in male rats exposed prenatally to stress. Physiol Behav. 84:97–104.
- Hardy MP, Gao H-B, Dong Q, Ge R, Wang Q, Chai WR, Feng X, Sottas C. 2005. Stress hormones and male reproductive function. Cell Tissue Res. 322:147–153.
- Harvey PW, Chevins PFD. 1984. Crowding or ACTH treatment of pregnant mice affects adult copulatory behavior of male offspring. Horm Behav. 18:101–110.
- Henry LA, Witt DM. 2002. Resveratrol: phytoestrogen effects on reproductive physiology and behavior in female rats. Horm Behav. 41:220–228.
- Herrenkohl LR. 1979. Prenatal stress reduces fertility and fecundity in female offspring. Science. 206:1097–1099.
- Herrenkohl LR, Politch JA. 1978. Effects of prenatal stress on the estrous cycle of female offspring as adults. Experientia. 34:1240–1241.
- Juárez-Rojas AL, García-Lorenzana M, Aragón-Martínez A, Gómez-Quiroz LE, Retana-Márquez S. 2015. Intrinsic and extrinsic apoptotic pathways are involved in rat testis by cold water immersion-induced acute and chronic stress. Syst Biol Reprod Med. 61:211–221.
- Kinsley C, Svare B. 1986. Prenatal stress effects: are they mediated by reductions in maternal food and water intake and body weight gain? Physiol Behav. 37:191–193.
- Kinsley CH, Mann FE, Bridges RS. 1992. Diminished luteinizing hormone release in prenatally stressed male rats after exposure to sexually receptive females. Physiol Behav. 52:925–928.
- Kyrylkova K, Kyryachenko S, Leid M, Kioussi C. 2012. Detection of apoptosis by TUNEL assay. Methods Mol Biol. 887:41–47.
- Lumey LH. 1998. Reproductive outcomes in women prenatally exposed to undernutrition: a review of findings from the Dutch famine birth cohort. Proc Nutr Soc. 57:129–135.
- Luo E, Stephens SBZ, Chaing S, Munaganuru N, Kauffman AS, Breen KM. 2016. Corticosterone blocks ovarian cyclicity and the LH surge via decreased kisspeptin neuron activation in female mice. Endocrinology. 157:1187–1199.
- Maccari S, Darnaudery M, Morley-Fletcher S, Zuena AR, Cinque C, Van RO. 2003. Prenatal stress and long-term consequences: implications of glucocorticoid hormones. Neurosci Biobehav Rev. 27:119–127.
- Michael AE, Pester LA, Curtis P, Shaw RW, Edwards CR, Cooke BA. 1993. Direct inhibition of ovarian steroidogenesis by cortisol and the modulatory role of 11 beta-hydroxysteroid dehydrogenase. Clin Endocrinol. 38:641–644.
- Myers M, Britt KL, Wreford NGM, Ebling FGP, Kerr JB. 2004. Methods for quantifying follicular numbers within the mouse ovary. Reproduction. 127:569–580.
- O’Brien JE, Peterson TJ, Tong MH, Lee E-J, Pfaff LE, Hewitt SC, Korach KS, Weiss J, Jameson JL. 2006. Estrogen-induced proliferation of uterine epithelial cells is independent of estrogen receptor α binding to classical estrogen response elements. J Biol Chem. 281:26683–26692.
- Ordyan NE, Fedotova YO, Pivina SG. 2013. Effects of prenatal stress on the activity of the pituitary-ovarian system in female rats. Bull Exp Biol Med. 155:433–435.
- Orr TE, Taylor MF, Bhattacharyya AK, Collins DC, Mann DR. 1994. Acute immobilization stress disrupts testicular steroidogenesis in adult male rats by inhibiting the activities of 17α-hydroxylase and 17,20-lyase without affecting the binding of LH/hCG receptors. J Androl. 15:302–308.
- Pallarés ME, Adrover E, Baier CJ, Bourguignon NS, Monteleone MC, Brocco MA, González-Calvar SI, Antonelli MC. 2013. Prenatal maternal restraint stress exposure alters the reproductive hormone profile and testis development of the rat male offspring. Stress. 16:429–440.
- Plana-Ripoll O, Li J, Kesmodel US, Olsen J, Parner E, Basso O. 2016. Maternal stress before and during pregnancy and subsequent infertility in daughters: a nationwide population-based cohort study. Hum Rep. 31:454–462.
- Plana-Ripoll O, Li J, Kesmodel US, Parner E, Olsen J, Basso O. 2017. Reproductive function in the sons of women who experienced stress due to bereavement before and during pregnancy: a nationwide population-based cohort study. Fertil Steril. 107:189–197.
- Politch JA, Herrenkohl LR. 1984. Effects of prenatal stress on reproduction in male and female mice. Physiol Behav. 32:95–99.
- Retana-Márquez S, Bonilla-Jaime H, Vázquez-Palacios G, Domínguez-Salazar E, Martínez-García R, Velázquez-Moctezuma J. 2003. Body weight gain and diurnal differences of corticosterone changes in response to acute and chronic stress in rats. Psychoneuroendocrinology. 28:207–227.
- Robaire B, Hinton BT, Orgebin-Crist MC. 2006. The epididymis. In: Neill JD, Plant MT, Donald WP, Challis JGC, De Kretser DM, Richards JS, et al., editors. Knobil and Neill’s physiology of reproduction. St. Louis (MO): Academic Press; p. 1081–1092.
- Rodríguez N, Mayer N, Gauna HF. 2007. Effects of prenatal stress on male offspring sexual maturity. Biocell. 31:67–74.
- Sato J, Nasu M, Tsuchitani M. 2016. Comparative histopathology of the estrous or menstrual cycle in laboratory animals. Toxicol Pathol. 29:155–162.
- Segarra AC, Luine VN, Strand FL. 1991. Sexual behavior of male rats is differentially affected by timing of perinatal ACTH administration. Physiol Behav. 50:689–697.
- Shono T, Suita S. 2003. Disturbed pituitary-testicular axis inhibits testicular descent in the prenatal rat. BJU Int. 92:641–643.
- Sugden MC, Langdown ML, Munns MJ, Holness MJ. 2001. Maternal glucocorticoid treatment modulates placental leptin and leptin receptor expression and materno-fetal leptin physiology during late pregnancy, and elicits hypertension associated with hyperleptinaemia in the early-growth-retarded adult offspring. Eur J Endocrinol. 145:529–539.
- Viltart O, Vanbesien-Mailliot CCA. 2007. Impact of prenatal stress on neuroendocrine programming. Sci World J. 7:1493–1537.
- Ward IL. 1972. Prenatal stress feminizes and demasculinizes the behavior of males. Science. 175:82–84.
- Ward IL, Ward OB, Affuso JD, Long WD III, French JA, Hendricks SB. 2003. Fetal testosterone surge: specific modulations induced in male rats by maternal stress and/or alcohol consumption. Horm Behav. 43:531–539.
- Ward IL, Weisz J. 1984. Differential effects of maternal stress on circulating levels of corticosterone, progesterone, and testosterone in male and female rat fetuses and their mothers. Endocrinology. 114:1635–1644.
- Weinstock M. 2001. Effects of maternal stress on development and behaviour in rat offspring. Stress. 4:157–167.
- Weinstock M. 2008. The long-term behavioural consequences of prenatal stress. Neurosci Biobehav Rev. 32:1073–1086.
- Weinstock M, Matlina E, Maor GI, Rosen H, McEwen B. 1992. Prenatal stress selectively alters the reactivity of thehypothalamic-pituitary adrenal system in the female rat. Brain Res. 595:195–200.
- Westwood RF. 2008. The female rat reproductive cycle: a practical histological guide to staging. Toxicol Pathol. 36:375–384.
- Whirledge S, Cidlowski JA. 2010. Glucocorticoids, stress, and fertility. Minerva Endocrinol. 35:109–125.
- Winuthayanon W, Hewitt SC, Korach KS. 2014. Uterine epithelial cell estrogen receptor alpha-dependent and -independent genomic profiles that underlie estrogen responses in mice. Biol Reprod. 91:1–10.
- Yazawa H, Sasagawa I, Ishigooka M, Nakada T. 1999. Effect of immobilization stress on testicular germ cell apoptosis in rats. Human Reprod. 14:1917–1920.
- Yun HJ, Lee J-Y, Kim MH. 2016. Prenatal exposure to dexamethasone disturbs sex-determining gene expression and fetal testosterone production in male embryos. Biochem Biophys Res Comm. 471:149–155.