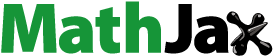
ABSTRACT
The protective capability of fringing reefs against tsunami hazards has been reported in numerous post-disaster surveys. It is believed that global warming is changing the water level over the reef flat and reef surface roughness by sea-level rise and coral bleaching. For a better understanding of the influence of climate change on tsunami hazards over fringing reefs, this study utilized a shock-capturing Boussinesq wave model, FUNWAVE-TVD, to simulate the tsunami-like solitary wave propagation and run-up over fringing reefs. Calibrated and validated by the newly obtained experimental data, the present model with shock-capturing scheme, in which only the ratio of wave height to water depth is used to trigger wave breaking, shows reasonable prediction of solitary wave transformation and run-up height over sharply varying reef bathymetry. Numerical experiments were then carried out to investigate the effects of sea-level rise and degrading of the reef surface roughness on the solitary wave inundation distance and fluid force distribution in the inundation zone. Numerical results clearly demonstrate how tsunami hazards change within the inundation zone in response to higher water levels and lower reef roughness and suggest climate change, especially sea-level rise, will significantly increase tsunami hazards in the low-lying areas of the reef-lined coasts. Presented results are discussed for the effects of sea-level rise and coral bleaching on the solitary wave process and implications to further improve the resilience under the threat of climate change.
1. Introduction
Submarine landslides, earthquakes, volcanic eruptions in open sea areas often trigger destructive shoreward tsunamis. Tsunami waves can run up over the coastal beach with large inundations and fast fluid velocities, devouring towns and villages as demonstrated in the 2004 Indian Ocean tsunami and the 2011 North-East Japan Tsunami. Fringing coral reefs are widely distributed along the coastlines in the tropical or sub-tropical regions, where many coastal areas are susceptible to tsunamis. A typical fringing reef is characterized by a seaward steep fore-reef face and an inshore shallow reef flat extending towards the back-reef beach (Yao et al. Citation2012). After the 2004 Indian Ocean Tsunami, the protective capability of fringing reefs against tsunami hazards for the reef-lined coasts has been reported in numerous post-disaster surveys. For example, in Sri Lank and the Maldives, coral reefs were found to have reduced the tsunami impact efficiently (e.g. Fernando et al. Citation2005; Goff et al. Citation2006; Okal et al. Citation2006) with wave heights and inundation distances 5–10 times lower than the plane natural beaches (Fernando et al. Citation2008). A substantial part of the incident energy can be dissipated by the strong wave breaking over the steep fore-reef face and by the bottom friction over the shallow and rough reef flat.
Global warming is a long-term rise in the average temperature of the Earth’s climate system. Ongoing and anticipated effects include rising sea levels, changing precipitation and expansion of deserts in the subtropics. Based on the Fourth Assessment Report of the Intergovernmental Panel on Climate Change (IPCC Citation2007), the global sea level will rise by up to 60 cm by the end of 21th century in response to ocean warming and glaciers melting. Several researches also revealed the possibility of future sea-level rise of up to 2 m by 2100 (e.g. Vermeer and Rahmstorf Citation2009; Grinsted et al. Citation2010). Moreover, it is believed that the above-average seawater temperatures caused by global warming are the leading cause of the coral bleaching (Michael Citation2016). These effects directly increase the water depth over the reef flat and degrade the reef surface roughness, which may have an impact on the protective capability of fringing reefs against tsunami hazards.
For a better understanding of the influence of climate change on tsunami hazards over fringing reefs, this study utilized a numerical model to simulate the tsunami-like solitary wave propagation and run-up over fringing reefs. Hydrodynamics associated with waves over sharply varying reef bathymetry is more complex than that on the plane beaches (Yao et al. Citation2012) and so far, Boussinesq-type models have been recognized as a both efficient and accurate method of reproducing reef hydrodynamics among various numerical models. The Boussinesq-type models employ a polynomial approximation to the vertical profile of velocity field, reducing one dimension of the three-dimensional problem. Over the last decades, improvements (e.g. Madsen and Sørensen Citation1992; Nwogu Citation1993; Wei et al. Citation1995) have been obtained with respect to classical restrictions to both weak dispersion and weak nonlinearity of the classical Boussinesq equations (Peregrine Citation1967). Moreover, as wave breaking does not naturally arise as weak discontinuous solutions in the dispersive Boussinesq formulations, ad-hoc dissipation terms as empirical breaking models (e.g. Schäffer et al. Citation1993; Kennedy et al. Citation2000) were added to the improved Boussinesq equations for accommodating wave breaking in the surf zone. “Slot” technique or porous-beach method (e.g. Tao Citation1983; Madsen et al. Citation1997; Kennedy et al. Citation2000) were also used for handling the moving shoreline in the swash zone. With these developments, Boussinesq-type models have been applied to simulate the regular (e.g. Skotner and Apelt Citation1999; Yao et al. Citation2012; Zhang et al. Citation2018), irregular (e.g. Nwogu and Demirbilek Citation2010; Yao et al. Citation2016; Zhang et al. Citation2018), and solitary (Yao et al. Citation2018) wave processes over fringing reefs. Comparing to the other depth-integrated models based on nonlinear shallow equations, the Boussinesq-type models are reported better in predicting the wave processes in deeper water in front of the fore-reef slope because of retention of dispersive terms (Yao et al. Citation2012, Citation2018). Recently, considerable efforts have been devoted to developing shock-capturing Boussinesq models (Tonelli and Petti Citation2009; Roeber and Cheung Citation2012; Shi et al. Citation2012; Fang et al. Citation2013; Kazolea et al. Citation2014), which use a hybrid method combining finite-volume and finite-difference schemes. Wave breaking can be treated in a more natural approach by switching the Boussinesq equations to nonlinear shallow water equations, making the implementation of an ad-hoc dissipation term for breaking wave unnecessary. Shoreline movement can also be captured in an efficient and accurate manner, avoiding the poor performance of “slot” technique in several cases involving inundations over complex bathymetries (Shi et al. Citation2016). Therefore, this study adopted a shock-capturing Boussiensq model named FUNWAVE-TVD (Shi et al. Citation2012), which developed a high-order shock-capturing TVD (total variation diminishing) scheme for the well-known original FUNWAVE model (Kirby et al. Citation1998). Kazolea et al. (Citation2019) has validated the present model with wave flume experiments (Roeber et al. Citation2010), involving solitary wave shoaling, breaking and bore propagation over fringing reefs. The computational time of the present model is significantly less compared to the models based on Navier-Stokes equations. But the run-up process over the back-reef beach was not included. In this study, the present model, version 3.0, released in December 2016, will be calibrated and validated with the newly performed laboratory measurements (Ning et al. Citation2018), including shoaling, breaking, bore propagation and running up a sloping back-reef beach. Using the validated model, the responses of solitary wave inundation distance and fluid forces within the inundation zone to climate change-related sea-level rise and reef surface roughness degrading were investigated and discussed to give some insights into the influence of climate change on tsunami hazards over fringing reefs.
The remainder of the paper is organized as follows. In Section 2, the numerical model utilized in this study is briefly introduced. In Section 3, laboratory experiments are introduced and numerical model are calibrated and validated with the experimental data for solitary wave transformation and run-up over a reef-beach system. In Section 4, setup and designed wave cases of numerical experiments are introduced. Numerical results of how solitary wave inundation distance and fluid force distribution within the inundation zone change in response to sea-level rise and the degrading of the reef surface roughness are presented. In Section 5, presented results are then discussed. Finally, in Section 6, overall conclusions are summarized.
2. Numerical model
In FUNWAVE-TVD, a hybrid finite volume-finite difference scheme is developed for the fully nonlinear and dispersive Boussinesq equations of (Chen Citation2006), extended to incorporate a moving reference level of Kennedy et al. (Citation2001). The governing conservation equation and momentum equation are expressed as:
where η is the surface elevation, the subscript t indicates partial derivatives with regard to time, is the horizontal gradient operator, M is the horizontal volume flux, which is expressed as:
where H = h + η denotes the total water depth, h is the still water depth. represents the horizontal velocity at a reference elevation z = zα, where zα = ζh + βh with ζ = −0.53 and β = 0.47 (Kennedy et al. Citation2001).
is the depth-dependent correction of velocity at o(μ2) (μ is the ratio of water depth to wave length) and written as:
where and
.
is the depth-averaged contribution to the horizontal velocity field given by:
V1 and V2 are dispersive Boussinesq terms and V3 represents the vertical vorticity at o(μ2). The term R stands for diffusive and dissipative terms, which include sub-grid lateral turbulent mixing and bottom friction. The bottom friction in this study is calculated by a quadratic friction law:
where n is the Manning coefficient.
In numerical scheme, dispersive terms are reorganized to construct a tridiagonal structure of spatial derivatives within time-derivative terms. The surface elevation gradient terms are also rearranged to obtain a numerically well-balanced form, which is suitable for any numerical order. Adaptive time steeping is implemented by a third-order Runge-Kutta method. Spatial derivatives are discretized using a combination of finite-volume and finite-difference methods. A high-order MUSCL (Monotone Upstream-centered Schemes for Conservation Laws) reconstruction technique, which is accurate up to the fourth order, is used in the Riemann solver. The wave breaking scheme follows the approach of Tonelli and Petti (Citation2009), who used the shock-capturing ability of the nonlinear shallow water equations (NSWE) with a TVD solver to simulate moving hydraulic jumps. Only the ratio of wave height to water depth ε is used to trigger wave breaking and all the dispersive terms are set to be zero when ε > 0.8. Shoreline movement is handled quite naturally as part of the Riemann solver underlying the finite volume scheme. More details about the present model can be referred to (Shi et al. Citation2012) and the users’ manual of FUNWAVE-TVD, version 3.0 (Shi et al. Citation2016).
3. Model calibration and validation
In this section, the present model is calibrated and validated with wave flume experiments. Laboratory experiments of solitary wave transformation over fringing reefs have been performed by Roeber et al. (Citation2010), but they did not include the run-up process. It is becoming clear that an accurate assessment of how waves affect fringing-reef coasts must consider shoreline run-up (Cheriton et al. Citation2016) since run-up is the primary process connecting reef flat wave dynamics and the beach (Beetham et al. Citation2016). Therefore, physical experiments were performed in the Ocean Experiment Hall of Zhejiang University to reproduce the whole process of solitary wave propagation over fringing reefs, including shoaling, breaking, bore propagation and running up a sloping back-reef beach. The experimental setup is shown in . The wave flume equipped with a piston-type wave maker at one side and a wave absorber at the other side is 35 m long, 0.6 m wide, and 0.8 m high. A physical model of an idealized reef-beach system, which consists of a 1:3 fore-reef slope, a 5.0 m horizontal reef flat with the height of 0.383 m and a 1:11.9 back-reef slope, was installed in the flume. The surface material of the flume bottom and physical model is smooth steel. The toe of the fore-reef slope is 12.75 m (x = 14.25) away from the wave maker. Six capacitance-wire wave gauges were placed vertically along the flume, where G1 was placed 6 m (x = 8.25 m) away from the toe of the fore-reef slope to record the incoming solitary wave height in deeper water in front of the fore-reef slop, G2, G3 and G4 were placed at x = 14.25 m, x = 14.8 m and x = 15.4 m to record the wave shoaling and breaking over the steep fore-reef slope and the remaining two (G5, G6) were installed at x = 17.4 m and x = 20.33 m over the reef flat. A 2.0-m-long capacitance-wire wave gauge G7 was laid on the back-reef slope to measure the run-up height. The run-up height is defined as the vertical distance between the surface elevation and still water level. Therefore, the data of G7 were transformed to the vertical run-up height R based on the geometrical relationship and corresponding horizontal inundation distance L can be calculated as 11.9R. The deeper water depth in front of the fore-reef slope in the experiments is 0.413 m, in which case the water depth above reef flat is 0.03 m. Incident solitary wave height is from 0.05 to 0.07 m. All generated solitary wave in the physical experiments were breaking over the fore-reef slope and formed bore-propagation over the reef flat. The design of the fore-reef slope, water depth over the reef flat, reef flat width and back-reef slope was based on the reported literatures (Quataert et al. Citation2015; Yao et al. Citation2018), following Froude similarity with a geometric scale factor of 1:100. Although actual tsunamis often appear as a combination of transient and nonperiodic waves, the solitary waves adopted in this study have been employed in many related studies as a benchmark in modelling the behaviours of tsunamis (e.g. Chang et al., Citation2009; Synolakis Citation1987; Maiti and Sen Citation1999; Quiroga and Cheung Citation2013) because the solitary wave is believed to represent many important properties of the leading wave of a tsunami.
In the model validation, the model setup is the same as that in the laboratory experiments. Internal wave maker (Wei and Kirby Citation1995) was implemented for the incident solitary wave. A small threshold as the minimum flooding depth, 1 mm, was used to simulate the flooding and drying of computational cells for run-up. Manning coefficient was set as 0.012 for the smooth steal based on standard hydraulic books. and show the comparison of measured and predicted time series of surface fluctuations at G1-G6 for the cases with the incident wave height of 0.05 and 0.07 m. Numerical results with dx = 0.05, 0.02 and 0.01 m are presented to demonstrate the sensitivity of grid spacing since the grid size is regarded as an important factor to introduce the right amount of energy dissipation for the present model (Shi et al. Citation2012; Chakrabarti et al. Citation2017), which uses the TVD technique in conjunction of non-linear shallow water equations. As seen in and , the grid size does not affect the performance of the present model much in predicting the solitary wave transformation over the fore-reef slope and reef flat since the solitary wave generated in this study has longer wave length than the short waves in Shi et al. (Citation2012) and Chakrabarti et al. (Citation2017). It is noted that there are phase discrepancies between the numerical results and experimental data at G6, which can be seen from the bore-arrival time. The finer the grid size is, the lager the phase discrepancy is. The largest phase discrepancy is observed between the numerical results with dx = 0.01 m and the experimental data at G6 in , which is about 0.6 s. Based on the Froude similarity in this study, 0.6 s is 6 s at the prototype scale, which may be negligible in the prediction of tsunami propagation. Therefore, despite phase discrepancies observed at G6, numerical results with all grid sizes reproduce the solitary wave motions with reasonable accuracy.
Figure 2. Measured and predicted time series of surface fluctuations at G1-G6 for the case with the incident wave height of 0.05 m.

Figure 3. Measured and predicted time series of surface fluctuations at G1-G6 for the case with the incident wave height of 0.07 m.

and show the comparison of measured and predicted time series of run-up height at G7 for the cases with incident wave heights of 0.05 m and 0.07 m. As seen in and , numerical results with fine gird sizes (i.e. dx = 0.02 m and dx = 0.01 m) are close to each other and predict better than the ones with coarse grid size (i.e. dx = 0.05 m). Simulated results of coarse grid size tend to underestimate the maximum run-up height, which may be due to the inadequate capacity of coarse gird in detection of the end point of run-up. Therefore, in order to capture the solitary wave run-up over fringing reefs accurately, we adopted dx = 0.02 m, which has shown enough accuracy, for the subsequent numerical study. With calibrated grid size, the present model shows reasonable prediction of solitary wave transformation and run-up height over sharply varying reef bathymetry. Notably, comparing to the empirical breaking models widely used in conventional Boussinesq-type models, the present model using shock-capturing method does not require several empirical parameters as breaking criteria to tune the additional dissipation term and only the ratio of wave height to water depth is used to trigger wave breaking. This ratio has been proved to be robust for the wave processes over the sloping plane beach (Shi et al. Citation2012) and here we demonstrate that this ratio is also valid for the solitary wave propagation over the sharply varying reef bathymetry, where parameters of empirical breaking models have to be tuned (Yao et al. Citation2012), with no need of tuning. The validation results presented in this paper are consistent with the previous work (Ning et al. Citation2018), but the results for the case with the incident wave height of 0.07 m was not presented in Ning et al. (Citation2018). Moreover, Ning et al. (Citation2018) suggested dx = 0.01 m is accurate enough for the case with the incident wave height of 0.05 m while this study confirms dx = 0.02 m is also accurate enough and more computationally efficient even for the case with the incident wave height of 0.07 m, which has smaller incident wave length.
4. Numerical experiment results
Using the validated model, several numerical experiments based on Froude similarity with the geometric scale factor of 1:100 were further carried out to investigate the influence of climate change on tsunami hazards over fringing reefs. Effects of climate change-related water depth increase over the reef flat and degrading of the reef surface roughness were focused on in this section. Numerical experiment setup is shown in . The solitary wave is initially located at x = 15 m, which is 12.75 m from the toe of the fore-reef slope and the whole computational domain of 40 m is applied with grid size dx = 0.02 m as calibrated above. Twelve cases were designed accordingly to investigate how the water-level rise over the reef flat and degrading of the reef surface roughness affect the maximum horizontal inundation distance and fluid force distribution in the inundation zone. Numerical experiment conditions, including incident wave height Hi, deeper water in front of the fore-reef slope hd, water depth over the reef flat hr, fore-reef slope angle , reef flat width w, back-reef slope angle
, manning coefficient for the horizontal bottom in front of the fore-reef slope n1, manning coefficient for the fringing reef n2 and manning coefficient for the back-reef slope n3 are listed in . In order to demonstrate the effect of sea-level rise and the degrading of the reef roughness independently, parameter hr is adjusted by changing the deeper water depth hd in Cases 1–6 and n2 is adjusted in Cases 7–12 while other parameters are kept unchanged. Parameters of Hi,
, w,
in have the same values as these in the laboratory experiments. It is reported that the water depth over the reef flat hr in reality is usually less than 4 m (Quataert et al. Citation2015) while the Fourth Assessment Report of the Intergovernmental Panel on Climate Change indicated that global sea level will rise by up to 60 cm by the end of 21th century in response to ocean warming and glaciers melting (IPCC Citation2007). Moreover, more researches also revealed the possibility of future sea-level rise of 2 m by 2100 (e.g. Vermeer and Rahmstorf Citation2009; Grinsted et al. Citation2010). Therefore, the maximum value of hr in was set as 6 cm to include the effect of sea-level rise. On the other hand, the varying range of n2 in is from 0.05 to 0.02. The minimum and maximum values of this range were set based on the values suggested by previous studies (Kunkel et al. Citation2006; Gelfenbaum et al. Citation2011), in which n2 = 0.02 represented a dead and smooth reef and n2 = 0.05 represented a healthy and normal reef. Besides, n1 was fixed as 0.02 (sand) for the horizontal bottom in front of the fore-reef slope and n3 was fixed as 0.04 (cobbles) for the back-reef beach.
Table 1. Numerical experiment conditions.
shows the computed maximum inundation distance Lmax as a function of the water depth over the reef flat hr for Cases 1–6. As seen in , the inundation distance increases significantly with the rise of the water depth over the reef flat.
Figure 7. Computed maximum inundation distance as a function of the water depth over the reef flat for Cases 1–6.

shows the computed spatial distribution of maximum momentum flux (Hu2)max within the inundation zone for Cases 1–6. H is the total water depth and u is the depth-averaged velocity. The horizontal axis of stands for the horizontal distance between any location in the inundation zone and the initial shoreline. Therefore, the origin of the horizontal axis of is the position of the initial shoreline. The actual tsunami flow is not steady, however, considering a long tsunami period, the run-up flow can be considered as quasisteady and the drag force F exerted on a surface-piercing surface in a steady flow can be applicable to represent the fluid force in the tsunami inundation zone (Yeh Citation2006a), which can be expressed as
Figure 8. Computed spatial distribution of maximum momentum flux within the inundation zone over the reef flat for Cases 1–6.

where ρ is fluid density, Cd is the drag coefficient and A is the wetted area of the body projected on the plane normal to the flow direction (i.e. A = Hb, b is breadth of the object). The drag coefficient Cd for steady flow varies from 1.2 to 2 (FEMA Citation2000), depending on the width-depth ratio. Therefore, with a proper value of the drag coefficient, an estimate of the fluid force on objects at a given location in the tsunami inundation zone can be obtained from Equation 7, once the momentum flux Hu2 in Equation 7 is estimated. As seen in , the fluid force distribution in the inundation zone is elevated obviously as a whole with the rise of the water depth over the reef flat.
shows the computed maximum inundation distance Lmax as a function of the Manning coefficient of the fringing reef n2 for Case 2 and Cases 7–12 and shows the computed spatial distribution of maximum momentum flux (Hu2)max within the inundation zone for Case 2 and Cases 7–12. As seen in and , the maximum inundation distance increases with the degrading of the reef surface roughness and the fluid force distribution in the inundation zone is elevated as a whole with the degrading of the reef surface roughness. But the effect of the degrading of the reef surface roughness is not as significant as the effect of the rise of the water level over the reef flat even the water depth over the reef flat was set to be shallow (2 cm) in these cases.
5. Discussion
Vertical accretion rates of the coral reefs are reported to be about 1–4 mm/year (Montaggioni Citation2005), which is an order of magnitude smaller than the anticipated rates of sea-level rise. This will result in a net increase in water depths over reef flats. Considering the rate of coral reef accretion and sea-level rise, the range of the water depth over the reef flat within Cases 1–6 definitely includes the effect of sea-level rise for the most of fringing reefs. The results presented in demonstrate the maximum inundation distance of the tsunami-like solitary wave increases more or less linearly with increasing the water depth over the reef flat. By nature, deeper water depth means less bottom friction induced by the reef flat. Moreover, it has been revealed by Zhou et al. (Citation2016) that deeper water depth also means less reflection by the fore-reef slope and less energy dissipation by the wave breaking for the solitary wave. shows the spatial distribution of the maximum surface elevation for Case 2 and Case 6. Reef profile is also demonstrated to indicate the locations. As seen in , shallower water depth over the reef flat induces early and more decay of the incident wave over the fore-reef slope, which is consistent with Zhou et al. (Citation2016). Therefore, the effect of sea-level rise over the reef flat on the solitary wave processes over fringing reefs should be significant, resulting in an obvious change of horizontal inundation distance over the back-reef beach.
Larger inundation distance indicates larger disaster area. The fluid forces in the inundation zone, on the other hand, serves as a measure of tsunami damage potential with engineering and environmental significance (Yeh Citation2006a). The fluid forces here mainly refer to the direct water forces, which is the fundamental cause of the tsunami damage (Yeh Citation2006b), while other local causes, such as impact of water-borne missiles, soil liquefactions and scours, winds induced by the wave motion and so on, are not covered. Nowadays, many reef-lined coasts are already heavily populated and engineered. The greater the fluid forces within the tsunami inundation zone, the greater the impact of the tsunami on structures and the greater the potential for loss of life. To better demonstrate the influence of the climate change on tsunami hazards over fringing reefs, the fluid forces distribution within the inundation zone is therefore investigated in this study. The results presented in demonstrate the whole fluid force distribution within the inundation zone is elevated with sea-level rise, which clearly indicates the tsunami damage should be enhanced over the back-reef beach as the sea level rises. It is also demonstrated the negative impact of sea-level rise on the tsunami damage is much more significant for the locations closer to the initial shoreline. In the face of inevitable sea-level rise, human must choose between protection and adaption (Nicholls and Cazenave Citation2010) and so far, coastal structures, such as seawalls and breakwaters, have been widely used in coastal areas including fringing reefs for shore and harbour protections from coastal disasters. Raising the design standard of these protecting structures should be the option. However, the results here suggests this option should be well assessed for fringing reefs as low-lying areas of the reef-lined coasts are highly threatened by the tsunami damage enhanced by sea-level rise and finding sustainable sources of aggregate and armour stone to maintain coastal protections is challenging and costly in many atoll islands (Yao et al. Citation2016). Additional protective methods or adaption, such as building coast seaward, against sea-level rise may be worthy of consideration for fringing reefs.
The results presented in and indicate the degrading of the reef surface roughness will also have a negative impact on tsunami hazards over fringing reefs, but the impact of it is not as significant as sea-level rise. shows the spatial distribution of the maximum surface elevation for Case 2 and Case 12. As seen in , unlike the water depth over the reef flat, the varying reef surface roughness has little effect on the wave processes over the steep fore-reef slope and just affects the wave propagation near the shoreline. Even so, it is worth mentioning that sea-level rise and coral bleaching are happening simultaneously in reality, in which case the degrading of the reef surface roughness may somehow enhance the negative impact of sea-level rise.
Figure 9. Computed maximum inundation distance as a function of the Manning coefficient of the fringing reef for Case 2 and Cases 7–12.

Figure 10. Computed spatial distribution of maximum momentum flux within the inundation zone for Case 2 and Cases 7–12.

In addition, this study only focuses on tsunami hazards over fringing reefs while extensive damage induced by stormy waves is another flooding hazard frequently reported in reef-lined coasts (e.g. Yamano et al. Citation2007; Tajima et al. Citation2014, Citation2017). Existing studies (e.g. Nwogu and Demirbilek Citation2010; Cheriton et al. Citation2016) have clearly found infragravity waves released from stormy wave propagation and amplified by the reef-beach system are the main cause of the large wave inundation along reef-lined coasts during typhoon events and the maximum infragravity wave height at the end of the reef flat are correlated with the relative submergence and wave steepness. The rough reef flat also plays an important role to determine the dissipation of incoming storm waves and the behaviour of infragravity waves (Shimozono et al. Citation2015; Tajima et al. Citation2016). Therefore, stormy wave run-up over fringing reefs may be affected largely by the climate change-related sea-level rise and coral bleaching. Moreover, recent projections (Storlazzi et al. Citation2015) suggest that climate change will also drive changes in extreme wave heights and their directions, which may further change the incident wave heights, water level over the reef flat and infragravity motions. Thus, the impact of climate change on stormy wave run-up over fringing reefs should be investigated more carefully and comprehensively. The present model with TVD shock-capturing scheme has shown its capability of predicting the irregular wave evolution and run-up over fringing reefs in recent numerical studies (Su et al. Citation2015; Ning et al. Citation2019). The present model, which can reasonably reproduce the mean water level, generation and spatial variations of infragravity waves and the run-ups dominated by the low-frequency motions, should be useful and efficient for further investigation of the impact of climate change on stormy wave run-up over fringing reefs.
6. Conclusions
In this paper, the shock-capturing Boussinesq wave model FUNWAVE-TVD was utilized to study the influence of the climate change on the tsunami-like solitary wave inundation over fringing reefs. Laboratory experiments, reproducing solitary wave shoaling, breaking, bore propagation and running up over fringing reefs, were used to calibrate and validate the numerical model. The present model with shock-capturing scheme, in which only the ratio of wave height to water depth is used to trigger wave breaking, shows reasonable prediction of solitary wave transformation and run-up height over sharply varying reef bathymetry. The grid size is especially important and needs to be calibrated to predict the maximum solitary wave run-up height over the back-reef beach accurately. The validated model was then utilized to investigate the effects of the water-level rise over the reef flat and degrading of the reef surface roughness on the solitary wave inundation distance and fluid force distribution within the inundation zone to better understand the influence of the climate change on tsunami hazards over fringing reefs. The numerical experiments clearly revealed a significant increase of horizontal inundation distance over the back-reef beach with the increase of the water depth over the reef flat. The tsunami damage will also be enhanced over the back-reef beach as the sea level rises, especially for the locations closer to the initial shoreline in the inundation zone. Additional protective methods or adaption may be necessary to reduce the tsunami damage highly enhanced by future sea-level rise for the low-lying areas of the reef-lined coasts. The negative effects of the degrading of the reef surface roughness on both inundation distance and tsunami damage are not as significant as sea-level rise. The results presented here provide coastal managers an estimate of how tsunami hazards change over fringing reefs in response to climate change-related sea-level rise and coral bleaching. Since climate change will have a significant negative influence on the protective capability of fringing reefs against tsunami hazards in the future, efforts in response to climate change, especially sea-level rise, will be necessary to mitigate the increased future tsunami hazards many tropical and sub-tropical shorelines will face.
Disclosure statement
No potential conflict of interest was reported by the authors.
Additional information
Funding
References
- Beetham E, Kench PS, O‘Callaghan J, Popinet S. 2016. Wave transformation and shoreline water level on Funafuti Atoll, Tuvalu. J Geophys Res. 121(1):311–326.
- Chakrabarti A, Brandt SR, Chen Q, Shi F. 2017. Boussinesq modeling of wave-induced hydrodynamics in coastal wetlands. J Geophys Res. 122(5):3861–3883.
- Chang Y-H, Hwang K-S, Hwung -H-H. 2009. Large-scale laboratory measurements of solitary wave inundation on a 1:20 slope. Coastal Eng. 56(10):1022–1034.
- Chen Q. 2006. Fully nonlinear Boussinesq-type equations for waves and currents over porous beds. J Eng Mech. 132(2):220–230.
- Cheriton OM, Storlazzi CD, Rosenberger KJ. 2016. Observations of wave transformation over a fringing coral reef and the importance of low-frequency waves and offshore water levels to runup, overwash, and coastal flooding. J Geophys Res. 121(5):3121–3140.
- Fang K, Zou Z, Dong P, Liu Z, Gui Q, Yin J. 2013. An efficient shock capturing algorithm to the extended Boussinesq wave equations. Appl Ocean Res. 43:11–20.
- FEMA. 2000. Federal Emergency Management Agency (FEMA) coastal construction manual. Washington (D.C): Publication number FEMA55.
- Fernando HJS, McCulley JL, Mendis SG, Perera K. 2005. Coral poaching worsens tsunami destruction in Sri Lanka. Eos. Trans Am Geophys Union. 86(33):301–304.
- Fernando HJS, Samarawickrama SP, Balasubramanian S, Hettiarachchi SSL, Voropayev S. 2008. Effects of porous barriers such as coral reefs on coastal wave propagation. J Hydro-environ Res. 1(3):187–194.
- Gelfenbaum G, Apotsos A, Stevens AW, Jaffe B. 2011. Effects of fringing reefs on tsunami inundation: American Samoa. Earth-Sci Rev. 107(1):12–22.
- Goff J, Liu PLF, Higman B, Morton R, Jaffe BE, Fernando H, Lynett P, Fritz H, Synolakis C, Fernando S. 2006. Sri Lanka field survey after the December 2004 Indian ocean Tsunami. Earthquake Spectra. 22(S3):155–172.
- Grinsted A, Moore JC, Jevrejeva S. 2010. Reconstructing sea level from paleo and projected temperatures 200 to 2100 ad. Clim Dyn. 34(4):461–472.
- IPCC, 2007. Climate Change 2007: The Physical Science Basis, Contribution of Working Group I to the Fourth Assessment Report of the Intergovernmental Panel on Climate Change. Cambridge University Press.
- Kazolea M, Delis AI, Synolakis CE. 2014. Numerical treatment of wave breaking on unstructured finite volume approximations for extended Boussinesq-type equations. J Comput Phys. 271:281–305.
- Kazolea M, Filippini A, Ricchiuto M, Abadie S, Martin Medina M, Morichon D, Journeau C, Marcer R, Pons K, LeRoy S, et al. 2019. Wave propagation, breaking, and overtopping on a 2D reef: A comparative evaluation of numerical codes for tsunami modelling. Eur J Mech. 73:122–131.
- Kennedy A,B, Chen Q, Kirby James T, Dalrymple Robert A. 2000. Boussinesq modeling of wave transformation, breaking, and Runup.I: 1D. J Waterw, Port, Coastal, Ocean Eng. 126(1):39–47.
- Kennedy AB, Kirby JT, Chen Q, Dalrymple RA. 2001. Boussinesq-type equations with improved nonlinear performance. Wave Motion. 33(3):225–243.
- Kirby JT, Wei G, Chen Q, Kennedy AB, Dalrymple RA. 1998. Fully nonlinear Boussinesq wave model. Documentation and user‘s manual. Center for Applied Coastal Research, Department of Civil and Environmental Engineering, University of Delaware. Report No.: CACR-98-06.
- Kunkel CM, Hallberg RW, Oppenheimer M. 2006. Coral reefs reduce tsunami impact in model simulations. Geophys Res Lett. 33(23):265–288.
- Madsen PA, Sørensen OR. 1992. A new form of the Boussinesq equations with improved linear dispersion characteristics. Part 2. A slowly-varying bathymetry. Coastal Eng. 18(3):183–204.
- Madsen PA, Sørensen OR, Schäffer HA. 1997. Surf zone dynamics simulated by a Boussinesq type model. Part I. Model description and cross-shore motion of regular waves. Coastal Eng. 32(4):255–287.
- Maiti S, Sen D. 1999. Computation of solitary waves during propagation and runup on a slope. Ocean Eng. 26(11):1063–1083.
- Michael S, 2016. The great barrier reef: a catastrophe laid bare. The Guardian.
- Montaggioni LF. 2005. History of Indo-Pacific coral reef systems since the last glaciation: development patterns and controlling factors. Earth-Sci Rev. 71(1):1–75.
- Nicholls RJ, Cazenave A. 2010. Sea-level rise and its impact on coastal zones. Science. 328(5985):1517.
- Ning Y, Liu W, Sun Z, Zhao X, Zhang Y. 2018. Parametric study of solitary wave propagation and runup over fringing reefs based on a Boussinesq wave model. J Mar Sci Technol.
- Ning Y, Liu W, Zhao X, Zhang Y, Sun Z. 2019. Study of irregular wave run-up over fringing reefs based on a shock-capturing Boussinesq model. Appl Ocean Res. 84:216–224.
- Nwogu O. 1993. Alternative form of Boussinesq equations for nearshore wave propagation. J Waterw, Port, Coastal, Ocean Eng. 119(6):618–638.
- Nwogu O, Demirbilek Z. 2010. Infragravity wave motions and runup over shallow fringing reefs. J Waterw, Port, Coastal, Ocean Eng. 136(6):295–305.
- Okal EA, Borrero JC, Synolakis CE. 2006. Evaluation of Tsunami risk from regional earthquakes at Pisco, Peru. Bull Seismol Soc Am. 96(5):1634–1648.
- Peregrine DH. 1967. Long waves on a beach. J Fluid Mech. 27(4):815–827.
- Quataert E, Storlazzi C, Rooijen A, Cheriton O, Van Dongeren A. 2015. The influence of coral reefs and climate change on wave-driven flooding of tropical coastlines. Geophys Res Lett. 42(15):6407–6415.
- Quiroga PD, Cheung KF. 2013. Laboratory study of solitary-wave transformation over bed-form roughness on fringing reefs. Coastal Eng. 80:35–48.
- Roeber V, Cheung K. 2012. Boussinesq-type model for energetic breaking waves in fringing reef environments. Coastal Eng. 70:1–20.
- Roeber V, Cheung KF, Kobayashi MH. 2010. Shock-capturing Boussinesq-type model for nearshore wave processes. Coastal Eng. 57(4):407–423.
- Schäffer HA, Madsen PA, Deigaard R. 1993. A Boussinesq model for waves breaking in shallow water. Coastal Eng. 20(3):185–202.
- Shi F, Kirby JT, Harris JC, Geiman JD, Grilli ST. 2012. A high-order adaptive time-stepping TVD solver for Boussinesq modeling of breaking waves and coastal inundation. Ocean Modell. 43-44:36–51.
- Shi F, Kirby JT, Tehranirad B, Harris JC, Choi Y-K, Malej M. 2016. FUNWAVE-TVD fully nonlinear Boussinesq wave model with TVD solver - documentation and user‘s manual (Version 3.0). Center for Applied Coastal Research, University of Delaware. Report No.: CACR-11-03.
- Shimozono T, Tajima Y, Kennedy AB, Nobuoka H, Sasaki J, Sato S. 2015. Combined infragravity wave and sea-swell runup over fringing reefs by super typhoon Haiyan. J Geophys Res. 120(6):4463–4486.
- Skotner C, Apelt CJ. 1999. Application of a Boussinesq model for the computation of breaking waves: part 1: development and verification. Ocean Eng. 26(10):905–925.
- Storlazzi CD, Shope JB, Erikson LH, Hegermiller CA, Barnard PL. 2015. Future wave and wind projections for United States and United States-affiliated Pacific Islands. U.s.geological Survey Open-File Report 2015–1001.
- Su S-F, Ma G, Hsu T-W. 2015. Boussinesq modeling of spatial variability of infragravity waves on fringing reefs. Ocean Eng. 101:78–92.
- Synolakis CE. 1987. The runup of solitary waves. J Fluid Mech. 185:523–545.
- Tajima Y, Lapidez JP, Camelo J, Saito M, Matsuba Y, Shimozono T, Bautista D, Turiano M, Cruz E. 2017. Post-disaster survey of storm surge and waves along the coast of Batanes, the Philippines, caused by Super Typhoon Meranti/Ferdie. Coastal Eng J. 59(1):1750009–1750011.
- Tajima Y, Shimozono T, Gunasekara KH, Cruz EC. 2016. Study on locally varying inundation characteristics induced by Super Typhoon Haiyan. Part 2: deformation of storm waves on the beach with fringing reef along the East Coast of Eastern Samar. Coastal Eng J. 58(1):1640003–1640024.
- Tajima Y, Yasuda T, Pacheco BM, Cruz EC, Kawasaki K, Nobuoka H, Miyamoto M, Asano Y, Arikawa T, Ortigas NM, et al. 2014. Initial report of JSCE-pice joint survey on the storm surge disaster caused by typhoon Haiyan. Coastal Eng J. 56(01):1450006.
- Tao J, 1983. Computation of wave runup and wave breaking. Internal Report, Danish Hydraulic Institute, Hørsholm, 40.
- Tonelli M, Petti M. 2009. Hybrid finite volume – finite difference scheme for 2DH improved Boussinesq equations. Coastal Eng. 56(5):609–620.
- Vermeer M, Rahmstorf S. 2009. Global sea level linked to global temperature. Proc National Acad Sci. 106(51):21527.
- Wei G, Kirby J,T. 1995. Time-dependent numerical code for extended Boussinesq equations. J Waterw, Port, Coastal, Ocean Eng. 121(5):251–261.
- Wei G, Kirby JT, Grilli ST, Subramanya R. 1995. A fully nonlinear Boussinesq model for surface waves. Part 1. Highly nonlinear unsteady waves. J Fluid Mech. 294:71–92.
- Yamano H, Kayanne H, Yamaguchi T, Kuwahara Y, Yokoki H, Shimazaki H, Chikamori M. 2007. Atoll island vulnerability to flooding and inundation revealed by historical reconstruction: Fongafale Islet, Funafuti Atoll, Tuvalu. Global Planet Change. 57(3):407–416.
- Yao Y, Becker JM, Ford MR, Merrifield MA. 2016. Modeling wave processes over fringing reefs with an excavation pit. Coastal Eng. 109:9–19.
- Yao Y, He F, Tang Z, Liu Z. 2018. A study of tsunami-like solitary wave transformation and run-up over fringing reefs. Ocean Eng. 149:142–155.
- Yao Y, Huang Z, Monismith SG, Lo EYM. 2012. 1DH Boussinesq modeling of wave transformation over fringing reefs. Ocean Eng. 47:30–42.
- Yeh H. 2006a. Maximum fluid forces in the Tsunami Runup Zone. J Waterw, Port, Coastal, Ocean Eng. 132(6):496–500.
- Yeh H. 2006b. Tsunami forces in the runup zone, caribbean Tsunami hazard. Singapore: World Scientific.
- Zhang S, Zhu L, Li J. 2018. Numerical simulation of wave propagation, breaking, and setup on steep fringing reefs. Water. 10(9):1147.
- Zhou Q, Zhan J-M, Li YS. 2016. Parametric investigation of breaking solitary wave over fringing reef based on shock-capturing Boussinesq model. Coastal Eng J. 58(02):1650007.