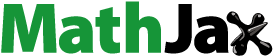
ABSTRACT
Saltwater intrusion has immediate influences on the water resources utilization, aquatic environment and ecological system. Hence, it is essential to detect the mechanisms of salt transport. This study adopted observations including instantaneous flow velocities, tide levels and salinity during 10 tide cycles at 2 stations in upstream and downstream of Qiantang Estuary in China. The variables of instantaneous flow velocity and salinity were decomposed into the time-average, time-varying and vertical-varying components using the flux decomposition model. Then, the salt flux components attributed to various physical processes were quantified. During spring tides, the longitudinal salt transport is controlled by advection transport and tidal pumping transport in the upper river reaches, while being mainly controlled by advection transport in the lower river reaches. The net water flow fluxes and salt fluxes are landward in the lower river reaches. However, the transport direction of the salt fluxes is inconsistent with that of the net water flow fluxes in the upper river reaches, where the net water flow fluxes are mainly seaward while the net salt fluxes are mainly landward. In general, there are significant positive correlations between the net landward salt flux and the tidal range. Thus, the tidal range can be used as an important indicator for determining the required amount of freshwater to be discharged from the reservoir in order to resist saltwater intrusion. The vertical distribution of salt in Qiantang Estuary is relatively uniform. The vertical shear force has limited influence on the salt transport. The research results are expected to supply a reference to future research on other macro-tide estuaries.
1. Introduction
An estuary is a place where water transits from land to sea and changes from freshwater to saltwater reciprocally. Due to the tide effects, the movement of estuarine currents is of the rectilinear type. The saltwater from the sea enters the estuary along with the tidal flood current. Therefore, saltwater intrusion is ubiquitous in an estuarine region, which can significantly influence the water resources utilization, aquatic environment and ecological system (Qi and Qiu Citation2011; Liu et al. Citation2017; Ye et al. Citation2017; Deng et al. Citation2017). To intake freshwater from the estuary, the decision-makers must understand the mechanisms of saltwater intrusion (Han et al. Citation2014). Meanwhile, the salt can be considered as a conservative tracer to quantify the convection and diffusion processes, and further used to determine the corresponding parameters for simulating pollution movement (Ali et al. Citation2010). Thus, it is essential to conduct research on the mechanisms of salt transport.
On the timescale of one tide cycle, the major part of materials transported by the periodic tidal currents that reciprocate in opposite directions tend to be offset. According to previous studies, the residuals are usually two orders of magnitude smaller than the variations of transported materials within a tide cycle (Medeiros and Kjerfve Citation2005). That is, on the timescale of one tide cycle, the transport amount of net material flux is not large. But the net transport is important to the long-term transport of materials in the estuary.
The net transport over the timescale of 10 or more tide cycles is normally recognized as the long-term transport (Shen and Wang Citation2007). Studies on the long-term transport of materials (such as water, sediment and salt) in estuaries generally focus on the residual flow, and the Euler residual current is widely used. However, it is incomplete to describe the long-term transport of materials as Euler residual current since the stocks drift velocity should also be included (Longuet-Higgins Citation1969). It is more appropriate to describe the long-term transport of materials as Lagrange residual current (Bowden Citation1967). Specifically, Feng et al. (Citation1984) proposed a weak nonlinear method to describe the Lagrange residual current and the corresponding transport process. Bowden (Citation1963) developed a flux mechanism decomposition method, which was applied to estuarine material transport. Other studies (e.g. Fischer Citation1972; Dyer Citation1974; Uncles and Jordan Citation1979; Uncles et al. Citation1985) have also been conducted to calculate substance transport based on the mechanism of flux decomposition. According to the mechanism of flux decomposition, the transport of substances can be decomposed into advection transport, tidal pumping transport and vertical circulation transport. The mechanism of flux decomposition, with its explicit physical concept, has been widely used to calculate estuarine fluxes and analyse the contribution of each dynamical factor of substance transport in different estuaries (Lewis and Lewis Citation1983; David and Kjerfve Citation1998; Miranda et al. Citation1998; Yu et al. Citation2014). According to previous studies carried out in San Juan River delta (Restrepo and Kjerfve Citation2002), Hudson Estuary (Lerczak et al. Citation2006), Merrimack Estuary (Ralston et al. Citation2010), Tampa Bay (Zhu et al. Citation2015) and other estuaries (Simpson et al. Citation2001; Sylaios et al. Citation2006), the salt flux is mainly controlled by tidal effects, and the advection and tidal pumping effects also play an important role.
However, the researches on the long-term transport mechanisms of salt in estuaries are insufficient, due to the difficulty in obtaining observational data with high quality of frequency and duration information. This study adopted observations including high-precision instantaneous flow velocities, tidal levels, salinity and other measured data of 10 tide cycles during spring tides at 2 stations in upstream and downstream of Qiantang Estuary. After that, the longitudinal residual currents and salt fluxes in the upstream and downstream of the estuary using the flux decomposition method were calculated, respectively. Lastly, the mechanisms of the longitudinal salt transport in Qiantang Estuary were detected and discussed. The results are expected to be used for estimating the roles of advection transport, tidal pumping transport and vertical circulation transport in the longitudinal transport of salt. Furthermore, this study contributes to a better understanding of the physical processes in a macro-tidal estuarine system.
2. Study area
Qiantang River is the largest river in Zhejiang Province, China. The location is shown in . It runs from west to east and flows into the East China Sea, with a watershed area of about 55,558 km2. The downstream part of Qiantang River starting from Fuchunjiang Power Station (in ) with a length of 282 km is recognized as the tidal reach, that is, Qiantang Estuary. Qiantang Estuary is a macro-tide estuary, with a maximum tidal range of 9.0 m, and 85% of the domestic water in Hangzhou city is taken from Qiantang estuary. Qiantang Estuary can be divided into three reaches according to its different hydrodynamic characteristics, which are runoff reach (from Fuchunjiang Power Station to Wenyan, 78 km), tidal current- and runoff-effected reach (Wenyan to Ganpu, 120 km), and tide-dominated reach (Ganpu to the mouth of the estuary, i.e. the Hangzhou Bay, 84 km). Water intakes of water plants are densely distributed by the river bank from Wenyan to Qibao (about 30 km), where the water quality is seriously affected by saltwater intrusion.
Hangzhou Bay has its mouth with a width of about 100 km and is narrowed down upstream to 16.5 km wide at Ganpu. With rapid narrowing, the tidal range is increased by up to 75% from the bay mouth to Ganpu, and the average tidal range at Ganpu is 5.60 m (in ). As the width of the upstream river section continues to narrow down, the tidal range decreases. The width at Qibao is 1.6 km, and the average tidal range is around 0.79 m. Tidal waves deform as they move upstream, and the duration of flood tide is gradually reduced, while the duration of ebb tide is gradually extended. For example, the average flood tide duration at Ganpu and Qibao is 5.47 and 1.42 h, respectively (in ).
Table 1. Tide characteristics along the Qiantang Estuary.
The average annual runoff of the estuary is 950 m3/s, but the runoff is unevenly distributed in different seasons. Seventy per cent of the rainfall is concentrated in March–July. Due to changes in runoff, the affected area of saltwater intrusion and the salinity in Qiantang Estuary exhibit significant seasonal variations. In the wet season (from March to July), the runoff is large and the degree of saltwater intrusion is low. The runoff from August to November is small while the tide is strong, thus large tidal flood current exacerbates saltwater into Qiantang estuary. The saltwater during spring tides can reach as far as 200 km upstream from the mouth of the bay. From December to February, the degree of saltwater intrusion is also relatively low, due to the reduced upward currents caused by the siltation in the riverbed above the Ganpu. Hence, to detect the tide effects on the longitudinal salt transport during spring tides, the measurements of tide process and salinity were conducted in October.
3. Analysis methodology
In this paper, the flux mechanism decomposition method based on field observation data (Medeiros and Kjerfve Citation2005; Ali et al. Citation2010) was adopted to detect the mechanisms of longitudinal salt transport. To calculate the long-term salt fluxes accurately, observational data with high-quality of frequency and duration information were collected.
3.1. Field measurement and data processing
Observations in two major stations, which are Qibao (Station A) and Ganpu (Station B), were selected to analyse the saltwater intrusion. Because the upper river reaches near Qibao provide water resource, the salinity in Qibao can be used to determine if there is saltwater intrusion in the water resource area. Ganpu is located at the upper reach of Hangzhou Bay and can be considered as the starting point of saltwater intrusion. The saltwater intrusion in Qiantang Estuary mainly occurs during spring tides, and in general the spring tides last 5–6 days. Thus, we investigated and measured the entire tide processes during spring tides from 25 to 30 October 2007 at the two stations. A relatively small survey vessel was used at a fixed point in Qibao, where the water depth is shallower. A large survey vessel (in ) was used to carry out the observation at a fixed point in Ganpu, which could resist the surge impact efficiently. A decimeter differential global positioning system receiver was used to orientate the survey vessel. The fixed position was detected at 3 h intervals, which could assure that there was no displacement. An auto-recording water level meter was adopted to measure the tide levels, and the records were verified with the observations measured by a manual water level. The water depth was measured using a depth sounder. The flow velocities and the flow directions were measured using a direct read out current meter and automatic data processing instruments. Water samples were extracted using horizontal trap samplers and their salinity was measured using high-precision salinometers. Six-point method was adopted to observe the flow velocity and salinity in the vertical direction, namely, surface layer (0.5 m beneath the water surface), 0.2 × water depth, 0.4 × water depth, 0.6 × water depth, 0.8 × water depth and bottom layer (0.5 m above the river bottom). They were measured every half an hour.
Runoff and tides are the two primary dynamic factors determining the saltwater intrusion in Qiantang Estuary (Han et al. Citation2014). During the observation period, the average daily runoff discharges ranged from 320 to 343 m3/s, which kept almost unchanged. Thus, the temporal variations of the observations were mainly determined by the variations of tides, rather than the variations of runoff discharge. Therefore, this measurement can be applied for analysing the effects of tides on saltwater intrusion specifically.
3.2. The mechanism analysis on flux transport
Flux refers to the physical quantity flowing through a given area (usually perpendicular to the direction of flow) per unit time. The mechanism analysis is to decompose the tidal current and concentration according to certain rules. The amount of material transport, the water flow transport and their corresponding decomposition terms are also calculated. Therefore, the relationships between the material fluxes transport and water flow fluxes transport can be analysed.
The crosswise distribution of salinity in Qiantang Estuary is relatively uniform. Therefore, the unit width fluxes decomposition was conducted along the main channel, while the lateral transport was neglected. The calculation formulas are expressed as follows.
Residual flow calculation and decomposition formulas
Let x be the longitudinal coordinate along the river channel, t is the time and z is the relative water depth (. The instant flow velocity u can be decomposed into a summation of a depth-average term
and its corresponding deviation term
, that is,
.
and
can be decomposed into the summation of mean term in one tide cycle and a tidal oscillation term, which are,
and
, respectively. Therefore, the instant velocity u can be expressed as
. Similarly, the instant salinity can be decomposed into
. The instant water depth
can be decomposed into mean term in one tide cycle
and a tidal oscillation term
, that is,
. The average water transfer amount per unit width along the river channel during one tide cycle is:
where is the average value during one tide cycle,
is average flow-related term;
relates to tidal range and flow velocity term, and is also called Stokes effect,
is the time of one tide cycle. Equation (1) can also be expressed as
where
where ,
,
are referred to as one-dimensional vertical average Euler, Stokes, and Lagrange residual currents, respectively. Euler residual current refers to the average transport caused by the average flow with removal of the periodic astronomical tide. The magnitude and direction of the Euler residual current mainly depend on the contrast of the flow velocity and duration of flood tide and ebb tide in one tide cycle; Stokes residual current characterizes the net drifting offset of water, and the value directly reflects the correlation between the change of water level and the change of flow velocity in one tide cycle; the Lagrange residual current refers to the average velocity of the fluid micelles during one tide cycle, that is, the net transport velocity of the water body.
(2) Salt flux mechanism decomposition method
According to the above-mentioned decomposition method of water depth, flow velocity and salinity, the salt flux transport per unit width in one tide cycle along the axis of the channel is:
where
where is the transport flux contributed by Euler residual current;
is connected with tidal currents, recognized as stocks drifting term; T1+ T2 is the Lagrange transport flux term, i.e. the advection transport;
is the tidal currents and salinity term,
is connected with tidal, tidal currents and salinity term,
is the changes of tidal water level and salinity term, and
is contributed by the tidal pumping effect;
is salinity changes correlated with the vertical velocity changes, that is, shear diffusion;
is flow velocity on the vertical section under tidal wave deformation;
is contributed by the vertical circulation effect.
4. Results and discussions
4.1. Hydrodynamic property processing
shows the observations of semi-menstrual tide level and salinity changes at Station A. During the neap tide period, the tidal range was small. As it transferred from the neap tide to the spring tide, the tidal range gradually increased, and both the high and low tide levels gradually increased. From 23 to 29 October, the low tide level and the high tide level increased by 1.3 and 2.1 m, respectively, and the tidal range also increased by 0.8 m. The salinity was very low during the neap tide period. While during the spring tide period, the salinity increased rapidly. After the spring tide period, salinity returned to low values. Thus, the saltwater intrusion at Station A occurs mainly during spring tides.
and show the changes of the tide levels, the depth-average flow velocities and the depth-average salinity during spring tides at Station A and Station B, respectively. As shown in , the tide level increased rapidly while ebb tide quickly turned into flood tide, and then the salinity increased rapidly. Although the flood tide duration of about 3 h was much less than the ebb tide duration of about 9 h, the velocities of the flood tide with maximum value of 1.8 m/s were far greater than those of the ebb tide with maximum value of 0.8 m/s at Station A. The maximum salinity was 1.3 g/L. However, the minimum salinity of each tide cycle was only about 0.05 g/L, indicating that salinity changed greatly during one tide cycle.
Figure 4. (a) Water level and (b) velocity versus salinity data collected at Station A (the velocity was positive if it was landward, while it was negative if it was seaward).

Figure 5. (a) Water level and (b) velocity versus salinity data collected at Station B (the velocity was positive if it was landward, while it was negative if it was seaward).

In , the durations of flood and ebb tidal phases at Station B were different from those at Station A. The flood tide duration at Station B in one tide cycle was about 5–6 h, close to that during the ebb tide periods. The maximum flood velocity was equivalent to the maximum ebb velocity. The changes of salinity were basically synchronized with the tide levels. The changes of salinity lagged behind the changes of flow velocity, and the occurrence time of salinity peak values was about 3 h later than that of the maximum flow velocity. During spring tides, the salinity increased gradually, indicating that the salt from the sea continued to accumulate in the river section.
shows the average water depths and tidal ranges at Station A and Station B during each tide cycle. With reference to ) and , during the first five tide cycles (about 60 h), the river section at Station A was in a tide-storing stage. The mean water depth, the tide level, tidal range, maximum flood velocity and salinity increased gradually. After that, the low tide level remained basically unchanged, and the high tide level alternated between high and low, and so did the mean tide depth, tidal range and maximum flood velocity. The changes of average depths and tidal ranges at Station B were similar to those at Station A.
4.2. Net velocity
Based on Equations (1)–(3), the residual currents of each tide cycle at Stations A and B were calculated and decomposed, respectively (in and , and in ). For Station A, as indicated in and ), the Stokes residual currents caused by tidal deformation were always transported to the land during the entire spring tide period and were closely correlated with the tidal ranges (in )). However, the Euler residual currents were mainly transported to the sea, and were negatively correlated with the tidal ranges (in )). The velocities of Euler residual currents ranged from −0.054 to 0.310 m/s, and the velocities of Stokes residual currents ranged from −0.040 to −0.006 m/s. The velocities of Euler residual currents were significantly larger than those of the Stokes residual currents, indicating that the residual current at station A was mainly controlled by Euler residual current. The velocities of Lagrange current ranged from −0.075 to 0.304 m/s and were correlated with the tidal ranges (in )). The transport directions of Lagrange residual currents during the 1–3 tide cycles were landward (in ), which corresponded to the rapid increase in the mean water depth during the 1–3 tide cycles (in )).
Table 2. Computed residual currents, tidal ranges and average water depths at Station A in each tide cycle during spring tides.
Table 3. Computed residual currents, tidal ranges and average water depths at Station B in each tide cycle during spring tides.
As to Station B, the Stokes residual currents caused by tidal deformation were similar to those at Station A and the corresponding transport directions were landward. The velocities of Stokes residual currents were significantly larger than those of Euler residual currents, indicating that the residual currents at station B were mainly controlled by the Stokes residual currents. shows that the Euler residual currents and the Lagrange residual currents were correlated with the tidal ranges. However, there was no obvious correlation between the Stokes residual currents and the tidal ranges.
4.3. Salt fluxes
and list the salt fluxes during each tide cycle at Stations A and B, respectively. For Station A, as illustrated in , the directions of net salt fluxes transport were landward during most of the tide cycles, which were opposite to those of the Lagrange residual currents during the 4–5 tide cycles (in ), indicating the inconsistency of water flow and salt transport. In ), T1 (Euler residual current transport) transported salt to the sea, and T2 (Stokes residual current transport) and T3 + T4 + T5 (tide pumping effect transport) transported salt to the land. They determined the directions of the net salt fluxes together. T6 + T7 (vertical circulation transport) was relatively small, indicating that the water in the river section was well mixed and the vertical distribution of salinity was relatively uniform. This is consistent with the previous research results (Han et al. Citation2014). T2 and net salt fluxes were closely correlated with the tidal range (in ). The net landward salt fluxes in the third, fifth and seventh tide cycles were larger, corresponding to the larger tidal ranges (in and ).
Table 4. Computed salt fluxes (kg/s) at Station A in each tide cycle during spring tides.
Table 5. Computed salt fluxes (kg/s) at Station B in each tide cycle during spring tides.
As to Station B, the directions of net salt fluxes transport were landward during the entire spring tidal period (in and )). T2 played a leading role in the salinity transportation, followed by T1. T3 + T4 + T5 and T6 + T7 had limited effects. The directions of T2and T6 + T7 in all the tide cycles were landward, and those of T1and T3 + T4 + T5in most of tide cycles were also landward. There was no obvious correlation between T2 and tidal range, but T1 and net salt fluxes were closely correlated to the tidal ranges (in ). When the tidal ranges were larger, more net salt fluxes were transported to land. T6 + T7 was relatively small, indicating that the vertical distribution of salinity in the river reach was relatively uniform.
4.4. Implications for society and environmental policy
With the rapid development of the economy and society, the water resources, shoreline resources, intertidal resources and ecological resources in Qiantang Estuary have been more extensively used than ever, and the problem of saltwater intrusion has attracted more and more attention.
Based on the above calculation results, the transport directions of net water flow and net salt fluxes were landward in the lower reach of Qiantang Estuary. While the net water flow fluxes in the upper reach were mainly transported to sea, which were opposite to those of the net salt fluxes during part of tide cycles. Therefore, it is improper to determine if saltwater intrusion will occur based solely on the directions of net water flow fluxes.
To ensure the safety of intaking water, freshwater discharged from the upstream reservoir is required to resist the saltwater intrusion in Qiantang Estuary. According to the results derived from this study, there were high positive correlations between the net landward salt fluxes and the tidal ranges. This finding is also consistent with previous studies (Qiu et al. Citation2012; Han et al. Citation2014). The tidal range can be used as an important indicator of saltwater intrusion in Qiantang Estuary. Nevertheless, this study mainly focuses on the influence of tide changes on saltwater intrusion, data were collected in a narrow range of river discharges, and therefore the effects of runoff were not discussed. In general, larger discharges from the reservoir are required during spring tides, compared with those during neap tides. To save the limited freshwater resources, decision-makers can optimize discharges of the upstream reservoir according to the tidal ranges. Further study on the relationship between the tidal ranges and the required discharges is essential.
In general, the shear force had limited influence on the salt transport, indicating that the vertical distribution of salinity was relatively uniform. Therefore, vertical differences in salinity are generally negligible when studying saltwater intrusion problems in Qiantang Estuary.
5. Conclusions
To investigate the saltwater intrusion in Qiantang Estuary, the salinity changes are analysed during spring tides in dry season when the runoff discharges had a narrow range in this study. High-accuracy observations of instantaneous flow velocities, tide levels, salinity and other measurements during 10 tide cycles at 2 stations in Qiantang Estuary are adopted and investigated. Based on the flux decomposition method, the longitudinal residual currents and salt fluxes in each tide cycle are calculated. The intrusion mechanisms of saltwater during spring tides in Qiantang Estuary are detected. The following conclusions can be drawn:
During spring tides, the net water flow fluxes are mainly transported to the sea, and the net residual currents are mainly controlled by the Euler residual flow in the upper reach of Qiantang estuary. While the net salt fluxes are mainly transported to the land, which are opposite to the net water flow fluxes. The Euler residual currents transport salt to the sea, while the Stokes and the tidal pump currents transport the salt to the land, and they jointly determine the directions of the net salt fluxes. The vertical shear force has limited influence on the salt transport.
In the downstream of the estuary, the transport directions of the net water flow fluxes and the net salt fluxes are landward. The net residual currents are mainly controlled by Stokes residual flow, and the Stokes effect plays a leading role in salt transport, followed by the Euler effect. The tidal pump effect and the vertical net circulation have limited influences on the salt transport.
There are significant positive correlations between the net landward salt fluxes and the tidal ranges. In order to ensure the safety of water intake and save valuable freshwater resources, the salt-resistant flow discharged from the upstream reservoir can be scheduled according to the predicted values of the tidal range.
At the beginning of the spring tides, the upper reaches of the estuary are in a stage of tide storage. During this period, the transport directions of the net residual flows and the net salt fluxes are landward, meanwhile the tidal ranges are enlarged, and the salinity increase, thus the upstream freshwater discharge should be largely increased to resist saltwater intrusion.
Disclosure statement
No potential conflict of interest was reported by the authors.
Additional information
Funding
References
- Ali A, Lemckert CJ, Dunn RJ. 2010. Salt fluxes within a very shallow subtropical estuary. J Coast Res. 26(3):436–443.
- Bowden KF. 1963. The mixing processes in a tidal estuary. Int J Air Water Pollut. 7(4/5):343–356.
- Bowden KF. 1967. Circulation and diffusion. Estuaries. 83:15–36.
- David LT, Kjerfve B. 1998. Tides and currents in a two-inlet coastal lagoon: Laguna de Terminos, Mexico. Cont Shelf Res. 18(10):1057–1079.
- Deng YJ, Young C, Fu XY, Song J, Peng ZR. 2017. The integrated impacts of human activities and rising sea level on the saltwater intrusion in the east coast of the Yucatan Peninsula, Mexico. Nat Hazards. 85(2):1063–1088.
- Dyer KR. 1974. The salt balance in stratified estuaries. Estuarine Coastal Mar Sci. 2(3):273–281.
- Feng SZ, Xi PG, Zhang SZ. 1984. The baroclinic residual circulation in shallow seas. Chin J Oceanol Limnol. 2(1):49–60.
- Fischer HB. 1972. Mass transport mechanisms in partially mixed estuaries. J Fluid Mech. 53(4):671–687.
- Han ZC, Shi YB, You AJ. 2014. Prediction and countermeasures of saltwater intrusion in the Qiantang Estuary. Adv Water Resour Prod. 2:62–74.
- Lerczak JA, Rockwell W, Chant RJ. 2006. Mechanisms driving the time-dependent salt flux in a partially stratified estuary. J Phys Oceanogr. 36(12):2296–2311.
- Lewis RE, Lewis JO. 1983. The principal factors contributing to the flux of salt in a narrow, partially stratified estuary. Estuar Coast Shelf Sci. 16(6):599–626.
- Liu BJ, Liao YY, Yan SL, Yan HH. 2017. Dynamic characteristics of saltwater intrusion in the Pearl River Estuary, China. Nat Hazards. 89(3):1097–1117.
- Longuet-Higgins MS. 1969. On the transport of mass by time-varying ocean currents. Deep-Sea Research. 16(5):431–447.
- Medeiros C, Kjerfve B. 2005. Longitudinal salt and sediment fluxes in a tropical estuary: itamaraca´, Brazil. J Coast Res. 21(4):751–758.
- Miranda LB, Castro BM, Kjerfve B. 1998. Circulation and mixing due to tidal forcing in the Bertioga Channel, Sao Paulo, Brazil. Estuaries. 21(2):204–214.
- Qi SZ, Qiu QL. 2011. Environmental hazard from saltwater intrusion in the Laizhou Gulf, Shandong Province of China. Nat Hazards. 56(3):563–566.
- Qiu C, Zhu JR, Gu YL. 2012. Impact of seasonal tide variation on saltwater intrusion in the Changjiang River estuary. Chin J Oceanol Limnol. 30(2):342–351.
- Ralston D, Geyer WR, Lerczak JA. 2010. Structure, variability, and salt flux in a strongly forced salt wedge estuary. J Geophys Res Atmos. 115:1–21.
- Restrepo JD, Kjerfve B. 2002. The San Juan Delta, Colombia: tides, circulations, and dispersion. Cont Shelf Res. 22(8):1249–1267.
- Shen J, Wang HV. 2007. Determining the age of water and long-term transport timescale of the Chesapeake Bay. Estuar Coast Shelf Sci. 74(4):585–598.
- Simpson JH, Vennell R, Souza AJ. 2001. The salt fluxes in a tidally energetic estuary. Estuar Coast Shelf Sci. 52(1):131–142.
- Sylaios GK, Tsihrintzis VA, Akratos C, Haralambidou K. 2006. Quantification of water, salt and nutrient exchange processes at the mouth of a mediterranean coastal lagoon. Environ Monit Assess. 119(1–3):275–301.
- Uncles RJ, Elliott RCA, Weston SA. 1985. Observed fluxes of water, salt and suspended sediment in a partially mixed estuary. Estuar Coast Shelf Sci. 20(2):147–167.
- Uncles RJ, Jordan MB. 1979. Residual fluxes of water and salt at two stations in the Severn Estuary. Estuarine Coastal Mar Sci. 9(3):287–302.
- Ye RH, Song ZY, Zhang CM, He Y, Yu SC, Kong J, Li L. 2017. Analytical model for surface saltwater intrusion in estuaries. J Coast Res. 33(3):712–719.
- Yu Q, Wang YW, Gao JH, Gao S, Flemming B. 2014. Turbidity maximum formation in a well-mixed macrotidal estuary: the role of tidal pumping. J Geophys Res. 119(11):7705–7724.
- Zhu J, Weisberg RH, Zheng LY, Qi HS. 2015. On the salt balance of Tampa Bay. Cont Shelf Res. 107(15):115–131.