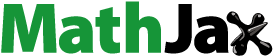
Abstract
The magnitude and direction of the in-situ stress field significantly influence the Geodynamic disaster. Taking the Gaojiapu Coal Mine in the northwest Binchang mining area as the study area, the distribution law of the in-situ stress field and its relationship with microseismic events were analyzed and discussed through field geological investigation, in-situ measurement, and numerical simulation. The results show that: (1) The Maximum horizontal principal stress (MaxHPS) is mainly concentrated in the turning end of the fold, the axis of the syncline, the undulation end of the anticline, the footwall of the fault, and the two ends of the fault. (2) The MaxHPS in the footwall area of the fault is greater than that in the hanging wall area. (3) The MaxHPS at the axial part of the syncline is greater than that at the wing part, and the MaxHPS at the wing part is greater than that at the anticline. (4) In areas with high MaxHPS and Lateral pressure coefficient (LPC), the frequency of high-energy microseismic events is high. (5) The 105J high-energy microseismic events more easily occur in areas with MaxHPS greater than 38 MPa and LPC greater than 1.50.
HIGHLIGHTS
Based on the stereographic projection analysis of the outcrop trace of the field rock stratum, combined with the in-situ stress test and structural evolution analysis, the regional in-situ stress field’s evolutionary history and distribution characteristics are analyzed.
The three-dimensional geometric simulation model is established by the numerical analysis method, the three-dimensional field stress field is inversed by multiple linear regression method, and the inversion results are tested and verified.
According to the mine seismic monitoring results and inversion results of the in-situ stress field, the relationship between the in-situ stress field and mining-induced seismicity was analyzed and discussed.
Introduction
In-situ stress affects engineering activities such as coal mining and rock tunnelling and is the fundamental force leading to the deformation and failure of underground engineering construction. The direction and magnitude of the in-situ stress field also have a significant influence on rock bursts (Hoek and Marinos Citation2009). In the high tectonic stress area, the rock mass would be deformed, destroyed, and broken during the excavation, and microseismic events and rock bursts usually occur in some areas (Gong et al. Citation2010; Xia and Ren Citation2013). Rock burst is a typical dynamic disaster that occurs in coal mines; it is characterized by abrupt, explosive, and urgent. It often causes heavy casualties and serious damage to the shaft and roadway (Dou et al. Citation2020). In the past decade, according to incomplete statistics, rock bursts have occurred in more than 100 mines in China, causing more than 300 deaths and nearly 1,000 injuries (Yuan et al. Citation2018). Therefore, it is essential to study the state of regional stress and analyze the stability of the surrounding rock to achieve safe excavation and underground engineering works.
Many studies have shown that in-situ stress measurement is a direct and effective method for studying the present in-situ stress field ( Zhao et al. Citation2013). Many scholars studied the distribution characteristics of regional stress through the field measurement of in situ stress (Kang et al. Citation2010; Zhang et al. Citation2020). However, the measurement of in-situ stress is usually expensive and time-consuming, and the causes of the in-situ stress field are complex; there are many influencing factors, and the measuring points are relatively scattered, which makes the measured results of the in-situ stress field prone to errors. To better analyze the distribution of the in-situ stress field, numerical modelling works have been widely carried out to understand the relation between regional stress regimes and scattered measuring points (Stephansson and Zang Citation2012; Ning et al. Citation2021). There are many methods for inverting the initial stress field, including multiple linear regression analysis, support vector machine, and genetic algorithm optimization (Li et al. Citation2012; Samui et al. Citation2015). Of course, The approximation degree of the value of inversion stress and the actual stress is an important criterion for validating inversed stress field results (Zhao et al. Citation2012).
The Binchang mining area is located in the Ordos Basin, which is one of the main mining areas of 13 coalfield bases in China-HuangLong Coal Field Base (Xu et al. Citation2012). Rock burst disasters have occurred frequently in recent years, along with the deep and large-scale development of coal resources. Since 2013, the Hujiahe coal mine, Tingnan coal mine, Gaojiapu coal mine, and Mengcun coal mines in the Binchang mine area have experienced different degrees of rock burst (Liu et al. Citation2017). Since the beginning of mining in July 2014, a coal burst has occurred many times in the Gaojiapu coal mine, and the situation of the coal burst disaster is very serious. This study takes the Gaojiapu coal mine in the Binchang mining area as the study area. The casing hole stress relief method was used to measure the in-situ stress field in the study area. Combined with the field measurement of stereo projection and the regional tectonic evolution analysis, the current in-situ stress field characteristics in the study area were determined. Considering the engineering geological conditions, the three-dimensional stress field in the study area was inversed, and the distribution law of the initial geo-stress field was analyzed. The relationship between the in-situ stress field and microseismic events was further investigated based on the results of microseismic monitoring in the mine area. The research results could serve as a reference for the design of good deep excavations and the evaluation of mine stability and provide support, which could also help predict and evaluate mine burst risks.
Study area
Engineering geological conditions and mining situation
Gaojiapu coal mine is located in the Binchang mining area, in the beam and gully region of the loess plateau of northern Shaanxi and eastern Gansu. According to the appraisal report on coal seam, roof, and floor impact tendency submitted by China Coal Research Institute, the uniaxial compressive strength of No.4 Coal Seam in Gaojiapu Coal Mine is 20.47 MPa, the dynamic failure time is 278.40 ms, the elastic energy index is 13.36, and the impact energy index is 3.20, respectively, and is identified as a coal seam with strong impact propensity; The bending energy index of the top and bottom strata of Coal Seam 4 is 54.52 kJ and 20.08 kJ respectively, which are identified as weak impact prone strata. The mine is seriously threatened by coal bursts during production. The geological structure of the study area is simple, with most of the strata being covered by the Quaternary loess layer, and the occurrence of the coal seam is relatively stable.
According to the planning and design data of the mine, the mining area is divided into four panels. Among them, the first and second panels have been mined. The 302 coalface of the third panel is presently being mined, the fourth panel is undergoing roadway excavation work, and the next replacement working face is the 301 coalface. There are 12 main faults and eight-fold structures in the mining area. Among them, nine faults with a throw of more than 10 m. The distributions of the main geological structures and the mining area are shown in . The strata in the study area from old to new are Jurassic Yanan Formation (J2y), Zhiluo Formation (J2z), Anding Formation (J2a), Lower Cretaceous Yijun Formation (K1y), Luohe Formation (K1l), Huachi Formation (K1h), and Quaternary strata. The research area’s engineering geological profile is shown in .
Microseismic monitoring system
An ‘SOS’ microseismic monitoring system has been installed in the first and second blocks of the mining area, which can be used to monitor the seismic activity in real-time and determine the source position and energy of the seismic (Zhou et al. Citation2020). From May 2019 to January 2021, 542 high-energy microseismic events with the energy larger than 105J occurred in the mining area (). Among them, there were 20 high-energy microseismic events above 106J. As shown in , most microseismic events occurred in the main roadway area of the first plate area, the middle part of the 204 and 205 coalfaces, and the 302 coalface.
Methodology
Measurement of in-situ stress
There are many methods to measure the in-situ stress in a rock mass (Sakurai and Akutagawa Citation1994; Ljunggren et al. Citation2003; Zang and Stephansson Citation2006), such as the hydraulic fracturing method, the stress relief method, and the acoustic emission method. Among them, the stress relief method has been more widely used, and the technology is more mature. When a rock is taken out of a rock mass under force, the elastic deformation accumulated in the rock will cause it to expand and recover. The YHY16 monitoring substation () and the KX2011 hollow core inclusion strain gauge () can be used to measure the expansion deformation of the core before and after the stress is relieved, and the elastic modulus and Poisson’s ratio of the rock can be measured with the calibrator for transducer calibrating confining pressure. The main body of the KX2011 hollow core is a hollow cylinder made of epoxy resin. Three groups of strain flowers are embedded at an equal distance along the same circumference in the middle layer of the cylinder. Each strain group consists of four strain plates, with an interval of 45°, as shown in .
Figure 2. In-situ stress testing equipment and Structural diagram of sensors. (a) YHY16 monitoring substation, (b) KX2011 hollow core inclusion strain gauge, (c) Hollow inclusion strain cell (Modified from Liu et al. Citation2021).

When the core material was released from a drill, 12 groups of deformation sensor readings changed, as shown in . Based on the elastic stress distribution of surrounding rocks in the circular cave, the least square method can be used to inversely calculate the magnitude and direction of the stress in the rock mass before the stress in the surrounding rock. The stress relief method was used to complete the testing of the eight measurement points (). These results are shown in .
Table 1. The measuring results in the study area.
Multiple linear regression
Currently, the multiple regression numerical calculation method of stress reverse analysis is widely used in engineering; it is a multiple linear regression method combining numerical calculation and multiple regression (Qiu et al. Citation2003). This method considers the gravity and geological structure effects of the initial stress field, and its reliability has been demonstrated in many engineering studies. Assuming that all influencing factors are linearly superimposed, the initial in-situ stress can be expressed by the following formula (Korkmaz Citation2021; Meng et al. Citation2021):
where σ0 is the stress regression value, σi is the regression of stress under condition i, wi is the regression coefficient, ε is the model error, C is the constant value, and n is the total number of basic boundaries.
To measure the significance of regression, the analysis is carried out through the test of the complex correlation coefficients R and F, and the correlation calculation formula is as follows (Wang and Li Citation2015; Deng Citation2018):
The complex correlation coefficient R is between 0 and 1, and the greater the magnitude, the better the regression effect. When the statistic value of F satisfies a certain confidence level a, the regression effect of the equation is remarkable.
In the inversion of the geo-stress field using the multiple linear regression method, the significance of the regression model does not mean that each independent variable has a significant impact on the dependent variable. Therefore, the t-test is used to test the significance of each independent variable. The correlation calculation formula is as follows (Meng et al. Citation2021):
where wii is the inverse matrix element of the coefficient matrix of the normal equation.
Determination of the boundary conditions of the in-situ stress inversion
The initial in-situ stress field is the stress state in the original rock mass itself and is generated by gravity and various tectonic stresses. It is also affected by external effects such as weathering and denudation. Energy is constantly released, and the stress is continuously redistributed, finally forming the present residual stress state. There are many causes of the initial in-situ stress field of a rock mass, such as its own characteristics, geological structure, temperature, and groundwater (Zhang et al. Citation2016). Actual engineering experience shows that the gravity of the rock mass and geological tectonics are the main factors affecting the initial in-situ stress in the rock mass (Hoek Citation2007). Therefore, in the inversion process, the following factors and calculation methods are mainly selected to invert the in-situ stress field (Hu et al. Citation2005; Su et al. Citation2011):
Gravity-weight stress: many actual engineering in-situ stress regression studies show that gravity stress is one of the = jm\][polkhgbv main causes of the formation of the in-situ stress field in a rock mass. Therefore, in the simulation of deadweight action, rigid displacement constraints are applied to all boundary surfaces other than the upper ground surface, and deadweight stresses are applied in the entire range of the calculation model ().
The horizontal uniform compression tectonic movement and the plane shear tectonic movement are important sources of tectonic stress and a key embodiment of plate movement. Therefore, five kinds of calculation methods are selected in the horizontal plane for simulation.
Horizontal compression tectonic movement in the X-axis direction of the horizontal direction: we select the boundary condition of applying displacement parallel to the X-axis direction. The displacement boundary conditions are shown in .
Horizontal compression tectonic movement in the Y-axis direction: the boundary condition of the displacement applied parallel to the Y-axis direction was selected. The displacement boundary conditions are shown in .
YZ plane shear tectonic movement (): we apply pairwise relative tangential displacement in the Z direction; a rigid displacement constraint was applied on the two boundary surfaces perpendicular to the X axis.
XZ plane shear tectonic movement (): we apply pairwise relative tangential displacement in the Z direction; Rigid displacement constraints were applied on two boundary surfaces perpendicular to the Y axis.
XY plane shear tectonic movement (): we apply pairwise relative tangential displacement in the Y direction; a rigid displacement constraint was applied on the two boundary surfaces perpendicular to the Z axis.
Analysis and significance test of the in-situ stress field inversion
Finite element software has been favoured by increasingly more researchers due to its powerful simulation and analysis capability, and is used to perform stress field inversion during the geotechnical engineering activity (Li et al. Citation2020). Based on the morphological characteristics of the main geological structures and the geological data of the study area, a three-dimensional geological model was established using the ANSYS finite element software. It included a total of 56995 nodes and 213272 units. Assign different rock mechanics parameters () to different elements in the geometric model according to the indoor physical and mechanical index experiments.
Table 2. Physical and mechanical parameters of the rock mass.
The stress field under each boundary condition was calculated according to the selected boundary conditions and the ANSYS 3D numerical model. The measured results of in-situ stress at the measured point are the results of the joint action of these boundary conditions. Hence, the above six boundary conditions are superimposed. The complete initial in-situ stress field can be obtained by multiple linear regression between the simulated data and the measured data. The simulated data and the measured data were imported into the software for Multiple linear regression calculation, and the regression coefficients of each boundary condition were obtained.
Two methods, the R test and the F test, were used to test the correlation between the independent and dependent variables. The significance of several influencing factors in the equation was obtained through regression. The correlation coefficients R = 0.934 and F = 46.97 were obtained by statistical calculation. The closer R is to 1, the stronger the correlation between the independent and dependent variables under the six calculation methods. In the F test, when the significance level is set as a = 0.05, the critical value Fa=(n,mn-n-1)=F(6,41)=2.33, Fa<F = 46.97 can be obtained from the distribution table of the F test, indicating that the independent variables selected in this article are significant as a whole. In addition, we used the t-test to test the significance of each independent variable further. The independent variable regression coefficient and t-test results are shown in .
Table 3. The independent variable regression coefficient and t-test results.
At the level of significance α = 0.05, t1-a/2 (mn-n)=t0.975(42)=2.019. Therefore, according to the comparison of test results in , at the level of significance α = 0.05, >t0.975(42)=2.019, meeting the significance test of the regression coefficient. Hence, the regression equation of the in-situ stress field of the mining area was established as follows,
where
and
represent the calculated results of the above six boundary conditions.
To further verify and analyze the accuracy and reliability of the inversion model, we compare the test data and regression data of Test points 1–3 of the above eight measured points. The comparison results are shown in .
It can be seen from that there are specific differences between the value of inversion stress and actual stress. Among them, the regression result of Test point 1 is greater than the test result, while the regression result of measuring point 3 is less than the test result. Overall, the regression results are very close to the test results, the error is basically within 10%, and the error between the regression data and the test data is within an acceptable range. Combined with the results of the above R test and F test, the in-situ stress at each node in the mining area can be calculated by regression formula. Therefore, the in-situ stress values of the 3 D model were calculated using the regression equation. The three-dimensional in-situ stress field distribution in the study area was finally obtained.
Results
Investigation and analysis of field structural features
The constructor is a rupture structure surface generated under the action of a constructive field effect or only a slight displacement. It can be used to restore the structural stress field. From the perspective of geomechanics, Sun et al. (Citation2006) used the measurement of conjugate shear joints and fault plane occurrence, scratch angle, and displacement sign together with stereographic projection to determine the direction of the principal stress axis. Sun et al. (Citation2008) conducted on-site in-situ stress testing using the casing hole stress relief method and analyzed the in situ stress field in combination with geomechanics and stereographic projection. The measured original rock stress in such studies agrees with the geomechanical analysis results. Lin and Xie (Citation2018) analyzed the fracture surface scratch and other tectonic traces using the stereographic projection method, judged the stages of the tectonic stress environment in the study area, and analyzed the essential characteristics of the tectonic stress environment in the area.
Through field geological surveys, we found that there are mainly two groups of joints on the surface of the study area and the surrounding areas (). These two sets of joints intersect at an inclination angle of approximately 90°, and there is no filling; they also often cut into each other as conjugate joints. Among them, the strikes of the joint planes are Eastern Northeast (NEE) (65°±10°N) and Northern Northwest (NNW) (335°±10°N). In addition, the joint surface in the NEE direction is more densely developed (). Based on the collected data on the occurrence of this group of conjugate joints in the study area, the stereographic projection method is used to judge the direction of the stress axis in the structural joints in the study area. The results are shown in .
Figure 6. Analysis of field geological survey in the research area. a ‘X’ conjugated heritage of the research area, b Fissures in Cretaceous strata outcrops, c the Stereographic projection method, d the maximum horizontal principal stress (MaxHPS) direction at the measurement point.

As shown in , the research area is mainly subjected to a tectonic stress field in the direction of Northern Northeast-Southern Southwest (NNE-SSW) and Western Northwest-Eastern Southeast (NWW-SEE). Among them, the tectonic stress with the direction of Northern Northeast-Southern Southwest (NNE-SSW) is stronger. Judging from the in-situ stress measured results (), the MaxHPS directions of the measured in-situ stress points in the study area are Northern-Northeast and Northeast, which are consistent with the results of field investigation and analysis.
Gaojiapu coal mine is located on the southwest edge of the Northern Shaanxi Slope structural unit in Ordos Basin, with the Tianhuan depression in the West and Weibei uplift in the south. In recent years, there have been many studies on the regional tectonic evolution of the Ordos Basin (Ding et al. Citation2015). Based on the regional geological tectonic evolution of the Ordos Basin (Zhao et al. Citation2017; Li et al. Citation2018), the current tectonic stress field in the study area is mainly affected by the Yanshan movement and the Himalayan movement. Since the collision of the Indian plate with the Eurasian plate and intracontinental subduction after the collision period, and the westward movement of the Pacific plate, orogenic movement continued to make the study area compressed, forming a tectonic stress field with NNE-SSW as the main compression direction. The field geological survey results and in-situ stress test results in the study area are consistent with the regional stress field analysis results of the above tectonic movement evolution analysis. Among them, the trend of cretaceous outcrop fractures is also consistent with the direction of structural stress field in the Himalayan period ().
Distribution characteristics of the MaxHPS and horizontal difference stress (HDS)
The initial in-situ stress field in the fold and the fault structure area is unevenly distributed. The MaxHPS near the fold is approximately symmetrically distributed along the fold crank axis trace. The MaxHPS near the syncline axis and the undulating end of the anticline are relatively high, showing stress concentration. The magnitude of the MaxHPS gradually decreases from the X3 syncline axis to the B4 anticline (). That is, the MaxHPS in the syncline axis is greater than that in the syncline wing, and the magnitude of the MaxHPS in the syncline wing is greater than that in the back inclined shaft. This result is similar to the results of Kang et al. (Citation2012), Han et al. (Citation2013), and Zhao et al. (Citation2019). It is worth noting that the stress concentration state of the MaxHPS has an obvious relationship with the angle of the fold structure fluctuation. The greater the wrinkle fluctuation, the more obvious the stress concentration and the greater the MaxHPS, while the side wall of the roadway will also have a certain degree of deformation ().
The distribution of the stress field near the fault structure is extremely complicated (Huang et al. Citation1998; Su et al. Citation2003). It can be seen from the inversion results of the in-situ stress field that there is a significant difference in the MaxHPS value between the fault structure area and the footwall on both sides of the fault. Except for the F2, F3, and F7 faults, the MaxHPS values at the footwall of the other faults are higher than that at the fault hanging wall, and the MaxHPS concentration exists in the footwall of the fault. This result is similar to the results of Xie et al. (Citation2019). This is because the stress on the hanging wall is a local stress that has been disturbed by the fault, while the footwall is less disturbed and should be consistent with the regional stress distribution. Among them, the F3 fault is caused by the influence of the X3 syncline, which makes the MaxHPS in the footwall lower than that in the hanging wall. However, the F2 and F7 may be affected by their own existence, resulting in a MaxHPS distribution that is not obvious. In addition, there is a stress concentration area at the end of the fault, which is consistent with the research results of Shen et al. (Citation2007) and Weng et al. (Citation2020). Combined with the effect of the F7 fault, the trace of the fault changes, and the MaxHPS also changes. In addition, the MaxHPS may vary when superposed by the action of faults (Shen et al. Citation2008).
The failure of rock mass is usually caused by shear failure. Therefore, the maximum shear stress reflects the stability of deep surrounding rock to a certain extent (Seredin et al. Citation2013; Cheng and Wang Citation2018). Based on the Rock mechanics theory, the HDS is the difference between the MaxHPS and the minimum horizontal principal stress (MinHPS), and the maximum shear stress is one-half of the HDS. The HDS not only reflects the difference between the MaxHPS and the MinHPS, but also reflects the characteristics of the maximum shear stress.
As shown in , in the fold structural area, the magnitude of the HDS is relatively low, and the local HDS in the axis of the X3 syncline and B2 anticline is larger than that in the two wings, indicating that the shear stress in the crankshaft of the fold is higher. The HDS is obviously higher in the fault structural area, especially in the middle of the two walls of the fault and at the end of the fault. From the middle of the fault trace to the end of the fault, the difference in the HDS values increases from gentle to sharp, indicating that there is a HDS concentration at the two ends of the fault, and the shear stress at the end of the fault is high.
Discussions
Analysis of the relationship between the mining-induced seismicity and the in-situ stress field
The seismicity is affected by many factors, such as in-situ and excavation induced stresses, etc (Naji et al. Citation2019). To further analyze the relationship between the mining-induced seismicity and the in-situ stress field, the plane distribution of microseismic events is analyzed and compared from three aspects: MaxHPS, HDS, and the lateral pressure coefficient (LPC). It is one of the control factors affecting the deformation and failure of underground surrounding rock (Gao et al. Citation2020). Most of the microseismic events are distributed in areas with high MaxHPS. This is especially near the 204 coalface and the 205 coalface, where there are more occurrences of microseismic events; the MaxHPS in this area is also relatively high.
It can be seen from the statistical data of seismic events and the MaxHPS in the study area () that in areas with lower MaxHPS, the number of microseismic events is relatively small. In areas where the MaxHPS is greater than 35.5 MPa, the number of microseismic events is 464, accounting for 85.61% of the total events. When the MaxHPS is greater than 39.5 MPa, there are fewer microseismic events. This is because most of the areas where the MaxHPS is greater than 39.5 MPa belong to the area to be mined, so there are obviously fewer microseismic events. As the MaxHPS increases, the number of microseismic events increases significantly. This indicates that it is easy for microseismic events to occur in areas with a large MaxHPS. With this, more microseismic events will occur in the future in the axis of the X3 syncline and the other regions where the MaxHPS is concentrated.
Looking at the distribution of the LPC and microseismic events in the study area, it can be seen that most high-energy microseismic events are distributed in areas with relatively high LPC (). According to the statistics, there were 432 microseismic events in mining areas with a LPC greater than 1.48, accounting for 79.7% of the total number of microseismic events. Among them, 12 high-energy microseismic events of 106J have a LPC greater than 1.53, accounting for 60% of the total number of high-energy microseismic events of 106J.
It can be seen from that when the LPC ranges from 1.48 to 1.68, a total of 385 microseismic events occurred. This is 3.5 times the number of microseismic events when the LPC ranges from 1.28 to 1.48. When the LPC is low, the number of microseismic events is significantly lower. With the increase in the LPC, the microseismic events increased significantly, showing an obvious proportional relationship. Generally speaking, microseismic events occur more easily in areas with a higher LPC.
Analysis of profile characteristics at 204 coalface and 205 coalface
Due to a large amount of microseismic data in 204 coalface and 205 coalface, the relationship between the initial in-situ stress field and microseismic events has been analyzed and discussed in the above chapters. There is an X2 syncline with NE-SW strike near the open-off cut of coalfaces 204 and 205, and there is an X3 syncline with NE strike in the middle of the coalface. Among them, the X2 syncline is almost consistent with the strike of the coalface, and the X3 syncline is almost perpendicular to the strike of the coalface. A profile line is arranged along the coal pillar in the middle of 204 coalface and 205 coalface. The comprehensive characteristic of the profile at 204 coalface and 205 coalface is shown in .
It can be seen from that the mining method of 204 coalface and 205 coalface is prone to mining first and then overhand mining. The coal seam thickness first increases and then decreases, which is thicker in the middle of the coalface and thinner on both sides. Within 250 m from the cutting hole, due to the low MaxHPS, small LPC, and small mining range, microseismic events are mainly within a certain range of coal seam roof and floor, with few times and low height.
Due to the influence of the X2 syncline, the MaxHPS and LPC first decrease and then increase. Within 250 m-750m from the cut hole, the MaxHPS and LPC increase gradually because the distance from the X3 syncline axis is gradually closer. Moreover, due to the increase in mining range, the number of microseismic events increases, and the height of microseismic events increases. Due to the influence of the X2 syncline, three times 106J high-energy microseismic events occurred near the end of the X2 syncline trace. Among them, within the range of about 750 m from the cutting hole, the MaxHPS is more than 38 MPa, and the LPC is more than 1.50. Many microseismic events have occurred within this range, the height of microseismic events is the largest, and 105J high-energy microseismic events occur frequently. After passing through the X3 syncline axis, the MaxHPS and LPC gradually decrease with the distance from the X3 syncline axis. With the increase of MaxHPS and LPC, the frequency of microseismic events gradually increases. The frequency of high-energy microseismic events will be higher in the areas with high MaxHPS and high LPC.
Conclusions
Through the field investigation, in-situ stress test, and numerical simulation, an accurate in-situ stress inversion model was established in this study, and the characteristics of the current geo-stress field and its impact on mining-induced seismicity were analyzed. The main conclusions are as follows:
Based on the field geological survey and analysis of the stereographic projection method, the study area is mainly affected by the tectonic stress field in NNE-SSW direction; According to the analysis of tectonic evolution, due to the collision of the Indian plate with the Eurasian plate and intracontinental subduction after the collision period, and the westward movement of the Pacific plate, orogenic movement continued to make the study area compressed, forming a tectonic stress field with NNE-SSW as the main compression direction. The MaxHPS directions of the measured in-situ stress points in the study area are Northern-Northeast and Northeast, which is consistent with the results of field investigation and tectonic evolution analysis.
The numerical analysis method establishes the three-dimensional geometric simulation model. Combined with engineering geological conditions, with the aid of 3D finite element numerical simulation and multi-linear regression methods, the initial stress field in the study area was inverted, and the R, F-tests, and t-tests verified the accuracy of the inversion results.
The initial in-situ stress field in the fold and the fault structure area is unevenly distributed. The MaxHPS is mainly concentrated in the turning end of the fold, the axis of the syncline, the undulation end of the anticline, the footwall of the fault, and the two ends of the fault. Among them, the MaxHPS at the axial part of the syncline is greater than that at the wing part, and the MaxHPS at the wing part is greater than that at the anticline. The MaxHPS in the footwall area of the fault is greater than that in the hanging wall area. The variation of the HDS is not obvious in the fold structure area, and there is a certain increment of differential stress locally.
Based on a comprehensive analysis, the frequency of microseismic events gradually increases with the increase of MaxHPS and LPC. The frequency of high-energy microseismic events will be higher in the areas with high MaxHPS and high LPC. When MaxHPS is greater than 38MPa and LPC is greater than 1.50, 105J high-energy microseismic events occur frequently.
Authors’ contributions
Xianggang Cheng performed implementation, organized the field test, and wrote the manuscript. Wei Qiao collected a part of the data and participated in drafting the manuscript. Linming Dou, Hu He, and Wei Ju participated in drafting the manuscript. Jinkui Zhang, Shikang Song, Heng Cui, and Hengzhi Fang collected and analyzed the data.
Disclosure statement
The authors declare that they have no known competing financial interests or personal relationships that could have appeared to influence the work reported in this article.
Data availability statement
The datasets used and analyzed during the current study are available from the corresponding author upon reasonable request.
Additional information
Funding
References
- Cheng Z, Wang JF. 2018. Quantification of particle crushing in consideration of grading evolution of granular soils in biaxial shearing: a probability-based model. Int J Numer Anal Methods Geomech. 42(3):488–515.
- Deng ZG. 2018. Danger evaluation of pressure bump in macro area based on 3D geostress field inversion. Coal Sci Tech. 46(10):78–82.
- Ding X, Tian JC, Chen JS, Yao JL, Deng XQ, Li YH. 2015. Paleogeographic framework and provenance features during Late Triassic Chang 9 time of the Yanchang Formation, Ordos Basin, China. Arab J Geosci. 8(9):6731–6743.
- Dou LM, Wang SC, Gong SY, Cai W, Li XL. 2020. Cloud platform of rock-burst intelligent risk assessment and multi-parameter monitoring and early warning. J China Coal Society. 45(6):2248–2255.
- Gao J, Xie SZ, Zhang XT, Wang HL, Gao WL, Zhou HM. 2020. Study on the 2D optimization simulation of complex five-hole cutting blasting under different lateral pressure coefficients. Complexity. 2020:1–12.
- Gong MF, Qi SW, Liu JY. 2010. Engineering geological problems related to high geo-stresses at the Jinping I Hydropower Station, Southwest China. Bull Eng Geol Environ. 69(3):373–380.
- Han J, Liang B, Zhang HW, Zhu ZH, Rong H, Zhang PT, Liang HP. 2013. Tectonic stress environment of coal and rock dynamic hazard in Kailuan mining area. J China Coal Society. 38(7):1154–1160.
- Hoek E. 2007. Practical rock engineering.
- Hoek E, Marinos PG. 2009. Tunnelling in overstressed rock. In: Vrkljan, I, editor. Rock Engineering in Difficult Ground Conditions-Soft Rocks and Karst. ISRM Regional Symposium EUROCK, Croacia: Taylor and Francis Group; p. 49–72.
- Hu B, Feng XT, Huang XH, Su GS, Zhou H. 2005. Regression analysis of initial geostress field for left bank high slope region at LongTan hydropower station. Chinese J Rock Mech Eng. 22:4055–4064.
- Huang XC, Xia XH, Shen WP. 1998. Measurement and back analysis on the initial rock stress field around the faults. J Shanhai Jiaotong Univ. 32(12):55–59.
- Kang HP, Wu ZG, Gao FQ, Ju WJ. 2012. Effect of geological structures on in-situ stress distribution in underground coal mines. Chinese J Rock Mech Eng. 31(S1):2674–2680.
- Kang HP, Zhang X, Si LP, Wu YZ, Gao F. 2010. In-situ stress measurements and stress distribution characteristics in underground coal mines in China. Eng Geol. 116(3–4):333–345.
- Korkmaz M. 2021. A study over the general formula of regression sum of squares in multiple linear regression. Numer Methods Partial Differential Eq. 37(1):406–421.
- Li LY, Du XL, Zhou J. 2020. Numerical simulation of site deformation induced by shield tunneling in typical upper-soft-lower-hard soil-rock composite stratum site of Changchun. KSCE J Civ Eng. 24(10):3156–3168.
- Li SZ, Zhao SJ, Liu X, Cao HH, Yu S, Li XY, Somerville I, Yu SY, Suo YH. 2018. Closure of the proto-tethys ocean and early paleozoic amalgamation of microcontinental blocks in East Asia. Earth Sci Rev. 186:37–75.
- Li YS, Yin JM, Chen JP, Xu J. 2012. Analysis of 3D in-situ stress field and query system’s development based on visual BP neural network. Procedia Earth Planet Sci. 5:64–69.
- Lin C, Xie XC. 2018. Study on tectonic stress environment based on Stereographic Projection Method. Coal Sci Technol. 46(8):32–37.
- Liu HB, Jiang B, He WY. 2021. Characteristics of the in situ stress field in the Yadian Coal Mine, Central China: implications for roadway differential deformation. ACS Omega. 6(1):739–746.
- Liu YH, Zhang Y, Zhao H. 2017. Current situation of Rock Burst prevention and cure of Shaanxi Binchang coal mine district. Coal Mining Technol. 22(6):92–95.
- Ljunggren C, Chang YT, Janson T, Christiansson R. 2003. An overview of rock stress measurement methods. Int J Rock Mech Min Sci. 40(7–8):975–989.
- Meng W, He C, Zhou ZH, Li YQ, Chen ZQ, Wu FY, Kou H. 2021. Application of the ridge regression in the back analysis of a virgin stress field. Bull Eng Geol Environ. 80(3):2215–2235.
- Meng W, He C, Yan QX, Guo DP, Li RL, Tang JC, Liao YK, Chen ZQ. 2021. Application of p-value approach in inversion of initial geo-stress field of rock masses. China Railway Sci. 42(01):71–79.
- Naji AM, Emad MZ, Rehman H, Yoo H. 2019. Geological and geomechanical heterogeneity in deep hydropower tunnels: a rock burst failure case study. Tunnel Undergro Space Technol. 84:507–521.
- Ning YB, Tang HM, Smith JV, Zhang BY, Shen PW, Zhang GC. 2021. Study of the in situ stress field in a deep valley and its influence on rock slope stability in Southwest China. Bull Eng Geol Environ. 80(4):3331–3350.
- Qiu XB, Li SC, Li SZ. 2003. 3D geostress regression analysis method and its application. Chinese J Rock Mech Eng. 10:1613–1617.
- Sakurai S, Akutagawa S. 1994. Back analysis of in-situ stresses in a rock mass taking into account its non-elastic behaviour. In Proceedings of the ISRM International Symposium on Integral Approach to Applied Rock Mechanics, vol.1, Santiago, Chile, p. 135–43.
- Samui P, Kim D, Aiyer BG. 2015. Pullout capacity of small ground anchor: a least square support vector machine approach. J Zhejiang Univ Sci A. 16(4):295–301.
- Seredin VV, Leibovich LO, Pushkareva MV, Kopylov IS, Khrulev AS. 2013. Evolution of fracture surface morphology in rocks. J Min Sci. 49(3):409–412.
- Shen HC, Yuanfang C, Jingyin W, et al. 2007. 3-D FEM inversion for the in-situ stress field around complex faults based on the theory of constrained optimization. Rock Soil Mech. 28(S1):359–365.
- Shen HC, Cheng YF, Wang JY, Zhao YZ, Zhang JG. 2008. Study on influence of faults on geostress by measurement data and numerical simulation. Chinese J Rock Mech Eng. 27(S2):3985–3990.
- Stephansson O, Zang A. 2012. ISRM suggested methods for rock stress estimation-part 5: establishing a model for the in situ stress at a given site. Rock Mech Rock Eng. 45(6):955–969.
- Su GS, Fu XY, Li SD. 2011. Inversion analysis of three-dimensional ground stress field based on FLAC3D. Yellow River. 33(02):142–145.
- Sun WC, Min H, Wang CY. 2008. Three-dimensional geostress measurement and geomechanical analysis. Chinese J Rock Mech Eng. 27(Suppl.2):3778–3784.
- Sun X, Yang ZR, Zhao ZY. 2006. Application of geomechanical analytic approach of the principal stress orientation. Chinese J Geotech Eng. 28(1):81–83.
- Su SR, Zhu HH, Wang ST, Stephansson O. 2003. Effect of physical and mechanical properties of rocks on stress field in the vicinity of fractures. Chinese J Rock Mech Eng. 22(3):296–296.
- Wang JN, Li F. 2015. Review of inverse optimal algorithm of in-situ stress filed and new achievement. J China Uni Mining Technol. 44(02):189–205.
- Weng JQ, Zeng LB, Lv WY, Liu Q, Kw ZU. 2020. Width of stress disturbed zone near fault and its influencing factors. J Geomech. 26(1):39–47.
- Xia M, Ren GM. 2013. Characteristics and mechanism of concentrated unloading in bank slope of Yangqu hydropower station. J Geol Soc India. 82(4):421–429.
- Xie KK, Shen Z, Huang LH, Chen CL, Wang ZR. 2019. Analysis and simulation of the impact of stress distribution law on rock burst. Chinese J Underground Space Eng. 15(S2):920–925.
- Xu LM, Zhou LF, Zhang YK, Dang B. 2006. Characteristics and tectonic setting of tectono-stress field in Ordos Basin. Geotectonica et Metallogenia. 30(04):455–462.
- Xu H, Tang DZ, Liu DM, Tang SH, Yang F, Chen XZ, He W, Deng CM. 2012. Study on coalbed methane accumulation characteristics and favorable areas in the Binchang area, southwestern Ordos Basin, China. Int J Coal Geol. 95:1–11.
- Yuan L, Jiang YD, He XQ, Dou LM, Zhao YX, Zhao XS, Wang K, Yu Q, Lu XM, Li HC. 2018. Research progress of precise risk accurate identification and monitoring early warning on typical dynamic disasters in coal mine. J China Coal Society. 43(2):306–318.
- Zang A, Stephansson O. 2006. Stress field of the earth’s crust. Heideberg: Springer; p. 115–116.
- Zhang P, Meng ZP, Jiang S, Chen XM. 2020. Characteristics of in-situ stress distribution in Zhengzhuang Region, Southern Qinshui Basin, China and its stress path during depletion. Eng Geol. 264:105413.
- Zhang SR, Hu AK, Wang C. 2016. Three-dimensional inversion analysis of an in situ stress field based on a two-stage optimization algorithm. J Zhejiang Univ Sci A. 17(10):782–802.
- Zhao HJ, Ma FS, Xu JM, Guo J. 2012. In situ stress field inversion and its application in mining-induced rock mass movement. Int J Rock Mech Min Sci. 53:120–128.
- Zhao JY, Zhou XG, Lei QH, Zhao GX, He YA, Shi JC, Zhang LY. 2017. Study on paleo-tectonic and present tectonic stress in Chang 7 tight reservoir of MaLing oilfield. Ordos Basin J Geomech. 23(06):810–820.
- Zhao XG, Wang J, Cai M, Ma LK, Zong ZH, Wang XY, Su R, Chen WM, Zhao HG, Chen QC, et al. 2013. In-situ stress measurements and regional stress field assessment of the Beishan area, China. Eng Geol. 163:26–40.
- Zhao YZ, Zhang NB, Deng ZG, Zhao SK, Chai HT, Li SG. 2019. Distribution characteristics and impact mechanism of in-situ stress field in fold tectonic mining area. Safe Coal Mines. 50(05):23–26 + 30.
- Zhou KY, Dou LM, Gong SY, Li JZ, Zhang JK, Cao JR. 2020. Study of rock burst risk evolution in front of deep longwall panel based on passive seismic velocity tomography. Geofluids. 2020:1–14.