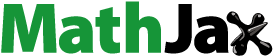
Abstract
Earthquake-induced landslides represent a significant proportion of seismic hazards in mountainous areas. Numerous slope stability analysis methods exist; however, only a few consider the interactions between pulselike seismic waves and landslides. This study investigates the seismic response characteristics of a homogenous step-like slope to evaluate the acceleration ground motion amplification along the surface. Parametric analysis focusing on the effect of pulselike waves on the slope is conducted using the finite difference modeling code Flac 3 D. Based on the numerical simulation results, pulselike seismic waves greatly influence the acceleration and velocity amplification factor (MPGA & MPGV), with maximum amplification usually obtained at the slope’s crest, where sliding failure initiates. The MPGV of rock slope under pulselike seismic waves is 9.3% higher than near-fault non-pulselike and 16% higher than the far-fault. The displacement for pulselike seismic waves is 20% higher than non-pulselike waves. The dynamic failure analysis presented deep failure for the slope under pulselike seismic wave compared to the non-pulselike. The dynamic failure calculated using Flac 3 D agrees with the results of Newmark-type displacement, with a marginal error between 1 and 8%. The study’s findings can be factored into reinforcing seismic engineering design and probabilistic stability analysis.
1. Introduction
Earthquake-induced landslides often threaten life and cause socio-economic losses. The 1999 chi-chi earthquake triggered about 9000 major landslides that killed more than 2415 people (Tsai et al. Citation2000). The Wangjiayan landslide triggered by the 2008 Wenchuan earthquake caused 1600 fatalitiesxu (Yin et al. Citation2009). The 2036 landslides triggered by the 2010 Yushu earthquake caused 2698 deaths, injured 12,135, and destroyed 15,000 houses (Xu et al. Citation2013). The Tarley earthquake-induced liquefaction also caused 74 direct fatalities, injured 124 others, and destroyed many properties (Mase et al. Citation2018, Citation2020, Citation2022). Post-landslide inventory and statistics indicate that seismic waves, slope material properties, and topography mainly induced the most predominant type of landslides (shallow and disrupted). Keefer (1984) showed that most landslides, particularly large-scale rock landslides, occur close to the faults (, less than 100 km).
Figure 1. Map and charts (a) Earthquake propagation direction’s effect on landslide scales at the Daguangbao landslide (Wenham earthquake),(b) Distribution of landslides and seismic fault correlation curve for the Wenchuan earthquake-induced landslides, the landslides inventory from Xu et al. (Citation2013), which contains 197,481 landslides, was used. The red point represents the distribution of landslides within 20 kilometers. (c) statistical distribution of large landslides at different distances from the Yingxiu-Beichuan fault.

Xu et al. (Citation2013) pointed out that 80% of the landslides triggered by the 2008 Wenchuan earthquake were within a 20 km range from the Yingxiu-Beichuan fault (). Xu et al. (Citation2013) indicated that most of the landslides triggered by the Yushu-earthquake occurred within 2.5 km of the surface fault rupture. This indicates that earthquake-induced slope instability is correlated with the earthquake surface rupture zone.
Zhang et al. (Citation2019) indicated that a distance within 20 km of fault rapture is within the near-fault range, termed 'Near fault ground motion (NFGM), and those above 20 km, also termed Far-fault ground motion (FFGM) in this study'. NFGM exhibits unique characteristics (i.e. rupture directivity, fling step, hanging wall thrusting, and vertical effect) (Somerville and Graves Citation2003; Yang et al. Citation2021). These characteristics induce the NFGM to produce longer periods, larger velocity and displacement pulse amplitudes, and higher peak ground acceleration (PGA), especially in the fault-normal (FN) direction. These attributes cause the NFGM to impose enormous demands on slopes and structures and cause severe damage compared to FFGM (Zhao and Chen Citation2015; Bao et al. Citation2021). Slope failure triggered by FFGM usually comprises sliding, whiles those of NFGM comprise both collapse and sliding (Bao et al. Citation2021). The source outcome of the NFGM causes them to arrive in a single or double coherent long-period pulse of motion, typically observed in its velocity-time history, termed Near fault pulse-like (NFPL) ground motion (Adanur et al. Citation2012). A static inventory of landslides shows that NFPL ground motion is relevant to catastrophic landslides; therefore, accurately predicting a slope’s dynamic response and failure process under NFPL ground motion is essential to seismic hazard studies. This has encouraged vast literature on dynamic response and failure process of slope response to NFPL ground motion (Zhang and Wang Citation2013; Kohrangi et al. Citation2019; Zhang et al. Citation2019; Buah et al. Citation2022).
The most widely used methods for estimating seismic-induced landslide hazards from the twentieth century include the pseudo-static analysis method (Petchkaew et al. Citation2021), the Newmark sliding block method, and numerical simulation methods (Jibson Citation2011). As a primary pseudo-static method, the limit equilibrium-based methods are limited to simple slope geometries and basic loading conditions (Gao et al. Citation2016). The Newmark sliding block method also underestimates displacement under pulse-like ground motion (Gischig et al. Citation2015).
This makes the numerical method the most favorable for studying the effect of NFPL ground motion on slope stability and failure (He et al. Citation2021, Citation2022; Qi et al. Citation2022).
Some recent numerical simulation methods in geotechnical engineering have also been explored (Nguyen et al. Citation2020; Petchkaew et al. Citation2021; Ongpaporn et al. Citation2022).
Chen and Song (Citation2021) used the numerical discrete element method to investigate the Chenhecun landslides (Gnasu china), considering fault distance, and presented that the sliding mass at the rear and bottom edges exhibits lower mobility than at the front and upper edges. Yang et al. (Citation2021) investigated the dynamic response of slopes to NFGM and reaffirmed the hazardous nature of the NFGM but did not consider the pulse effects of the seismic waves. Considering the limited literature on the topic, Buah et al. (Citation2022) used finite element software Flac 3 D to compare the effect of NFPL and non-pulselike ground motions on the dynamic response of a homogenous slope and indicated that NFPL ground motion causes significant damages compared with the non-pulselike.
It is evident from this literature that the Pulse effects of seismic waves on the dynamic response of slope are not well understood, and previous seismic slope stability analysis studies used to develop landslide disaster mitigative measures may have underestimated the pulse effect of seismic waves due to lack of a strong ground motion database, therefore there is a need for a systematic study on the topic (Zhang et al. Citation2019).
In this study, the time-domain procedure is adopted to determine the seismic response characteristics of a homogenous rock mass slope using the Flac 3 D finite difference code to perform numerical dynamic analyses on the slope structure. The wave propagation, peak ground acceleration (PGA), and peak ground velocity (PGV) amplification effect of indicated monitoring points on the slope’s surface is analyzed considering vertical seismic wave propagation direction. The relationship between the natural frequencies of the slope and its dynamic deformation characteristics is clarified using five different naturally recorded NFPL ground motions. The PGA, and PGV amplification result for NFPL ground motion obtained from the simulation is compared with results from five near-fault non-pulse-like ground motions (NFNPL) and five far-fault non-pulselike ground motions (FFNPL). The result from the possible slope displacement under NFPL ground motion is verified using the Newmark-type hazard displacement simulation. The dynamic failure mechanism of the slope is discussed based on the time-domain analyses. These results can be factored into the probabilistic seismic stability analysis.
1.1. Background of pulselike ground motion
Structural response to the NFPL ground motion is primarily different and more dangerous than non-pulselike ground motions (Mavroeidis and Papageorgiou Citation2003; Adanur et al. Citation2012). NFPL ground motions usually have PGA higher than 1.0 g, PGV higher than 2.0 m/s, and higher PGD (peak ground displacement) due to the presence of velocity pulses (Kalkan and Kunnath Citation2006; Adanur et al. Citation2012). The velocity pulse is influenced by the directivity, fling step, hanging wall, and vertical effects characteristics of the ground motion. The generating principle of the velocity pulse can be explained using a strike-slip fault; for example, the energy generated by each rupture will be superimposed when the near-fault site is at the end of the fault along the fracture strike. As a result, a velocity pulse will be formed produced by the rupture directivity pulse and fling step of the propagating wave in a direction perpendicular to the fault strike (). The NFPL ground motion contains visible pulses, single or double. It forms a significant part of the total energy that destroys slopes and structures during an earthquake. Therefore, the response of a slope to different pulse-like ground motions is analyzed in this study.
2. Method and materials
Numerical modeling of slope dynamic analysis is performed using the Fast Lagrangian Analysis of Continua in 3 Dimensions continuum code, Flac 3 D (Gischig et al. Citation2015; Itasca Consulting Group Citation2017). Flac 3 D is a numerical code for advanced geotechnical analysis of soil, rock, and structures in 3 Dimensions. The code permits the user to define a numerical model to provide explicit solutions to soil and rock mechanic problems. The codes also permit similar algorithms to be used for static and dynamic analysis with the differences in damping and soil densities (Itasca Consulting Group Citation2017). Flac 3 D codes are based on spatial discretization that operates on the principles of finite difference methods. The steps in using the Flac 3 D code for simulation include defining a numerical model, discretizing it with user-defined meshing, assigning constitutive, material properties, and examining the model’s response using finite difference principles to complete the project (Itasca Consulting Group Citation2017). Flac 3 D code can simulate wave propagation in x, y, and z directions. Damping due to the loss in frictional energy is considered. The numerical model in this study is assigned elastic properties; therefore, although it is deformable, but not permitted.
2.1. Numerical simulation
The numerical simulation model is undertaken using the Flac 3 D software based on the finite difference method (FDM) principles. The model is simplified and analyzed using 2 D analysis of Flac 3 D. The size of the numerical model is adopted considering computation accuracy. Based on Li et al. (Citation2019); Ma et al.(Citation2021), the proposed distance from the slope toe to the left boundary (L2, in ) is 1.5H, and the distance from the slope crest to the right boundary (L1 in ) is 2H. The model height, H (the difference between the slope toe and crest), is 20 m with a 45° inclination angle. The simulation results show that the numerical model size has minimal influence on the dynamic response and failure of the slope. Itasca Consulting Group (Citation2017) states that Mesh size influences dynamic analysis accuracy. The numerical model mesh size (in this study) is adopted based on the geotechnical materials’ shear wave velocity and the incident wave’s maximum frequency. (Assimaki et al. Citation2005) pointed out that, for an efficient wave transmission representation through a model, the mesh size (Δl) in the wave propagation direction should be less than at least one-tenth to one-eighth of the shortest wavelength (λ) associated with the frequency component of the input wave (EquationEq. (1)(1)
(1) ).
(1)
(1)
Figure 3. Numerical Model of the homogenous rock slope showing the layout of the monitoring points, mesh, boundary, and wave input (vertical direction).

Considering boundary conditions of a semi-infinite space body (i.e. slope) is essential in the dynamic response analysis because wave refraction at the boundary may influence the simulation results (Khanbabazadeh et al. Citation2022). The numerical model for this study is a rock slope with an extensive foundation; therefore, a static/viscous boundary condition is selected at the bottom. Free field boundaries are applied at both the slope’s left and right side boundaries to prevent wave reflection into the model. To analyze the seismic response of the slope, Seven monitoring points (P1–P7), are arranged on the free surface, with three other points (P8, P9, P10) fixed within the slope to measure the accuracy of the model boundary and the input wave (). Local damping with a coefficient of 0.156 is adopted (Chowdhury and Dasgupta Citation2003). When a viscous boundary is used, the seismic input must be transformed from acceleration history to stress (force) history before application (EquationEq. (2)(2)
(2) ) to avoid the effect of rock gravity (Song et al. Citation2021).
(2)
(2)
where σs(t) is the time-dependent shear stress at the bottom boundary of the slope, Vs is the sine-wave velocity, and vh is the instantaneous horizontal velocity of the input wave. Ρ is the material density.
2.2. Materials properties
The rock mass selected is assumed to be isotropic, linear, and elastic in numerical modeling. The rock is selected according to the rock classification scheme in the Standard for Engineering Classification of Rock Masses of China GB-50218-94(1994) which considers rock mass hardness and intactness based on its qualitative characteristics and basic quality which ranges from 550–451. The mechanical and physical properties of the rock materials used in the simulations are listed in and . The properties in are used for the dynamic analysis, whiles those in are used for failure analysis. About 30,456 surface strain elements and 17,970 grid nodes were generated in the model.
Table 1. Rock properties for dynamic response.
Table 2. Rock properties for slope failure analysis.
2.3. Input seismic wave selection
This study aims to accurately determine the dynamic response of slope to pulselike ground motions. Therefore, selecting the horizontal seismic records must be done carefully. Randomly selected seismic records may have different acceleration amplitudes, and the dynamic response results cannot truly reflect the actual situation. In this case, the ideal situation was to use ground motion records with the same PGA which was quite impossible to obtain because we needed to select ground motions from a wide range of seismic events to enhance accuracy. Fifteen horizontal directional loadings from six earthquake events are selected and loaded at the fixed boundary (bottom) of the model (Vertical-direction) (; Song et al. Citation2021). They include the seismogram from the Mw = 7.62 September 21, 1999, Chi-Chi earthquake (Tsai et al. Citation2000; Adanur et al. Citation2012; Tsai et al. Citation2019), the Mw =6.53 October 15, 1979, imperial valley earthquake (Adanur et al. Citation2012), the Mw = 6.69 January 17, 1994, Northridge earthquake (Champion and Liel Citation2012; Zhang et al. Citation2013; Bao et al. Citation2021), the Mw = 6.63 October 23, 2004, Niigata earthquake (Wu et al. Citation2020; Yang et al. Citation2021)), and the Mw =6.54 November 24, 1987, Superstition Hills earthquakes (Zhang et al. Citation2020). The acceleration amplitudes for all selected ground motions were modulated (Baker Citation2007) to have the same PGA before being used (). However, their PGV remained unchanged. The dominant frequency wave is 7.5 Hz, t = 60 s, and Δt = 0.005 s. The input wave is filtered using the undecimated wavelet transform method at a low-frequency bandpass to minimize noise (Chanerley and Alexander Citation2010) and baseline corrected as required (Chanerley et al. Citation2013). The dynamic amplitudes obtained show good correspondence with the input acceleration time history, which indicates the appropriateness of the earthquake loading input for the numerical simulation. These records were taken from Pacific Earthquake Engineering Research (PEER) ground motion data (Chiou et al. Citation2008). Based on their properties, the records are grouped into NFPL, NFNPL, and FFNPL earthquake ground motion
Figure 4. Acceleration time histories for the scaled NFPL, NFNPL, and FFNPL earthquake ground motions ().

Table 3. Properties of ground motion records selected.
2.3.1. Pulse extraction
Zou et al. (Citation2017) pointed out that when the ratio of the PGV to PGA of a ground motion is above 2.0 (i.e. PGV/PGA > 2.0), there is the presence of velocity pulses. Studies involving pulse-like ground motion can be grouped into three categories. First is identifying the pulse inherent in a near-fault ground motion. The second is developing an empirical predictive relationship of pulse-like ground motion characteristics. The third is assessing the potential damage of pulse-like ground motion to various structures and natural slopes (Zhang et al. Citation2015). This study belongs to the third category; however, to analyze the response and damage of slope under pulse-like ground motion, the velocity pulse of the wave must first be extracted. The energy, wavelet analysis, and Mavroeidis and Papageorgiou (M&P) wavelet methods (Somerville et al. Citation1997; Baker Citation2007) can identify and extract the velocity pulse of near-fault ground motion. This study adopts the M&P wavelet method by Mavroeidis and Papageorgiou (Mavroeidis and Papageorgiou Citation2010) as a proper function (EquationEq. (3)(3)
(3) ) to extract the largest velocity pulse. The method’s advantage is removing the influence of high-frequency content in the extracted pulse. The mathematical representation of the pulse using the M&P wavelet method depends on five physical parameters. These parameters were obtained based on observers’ experiences in the past. Therefore the extracted pulses suffered significant subjectivity and low efficiency. The M&P wavelet is derived from the Gabor wavelet (Velmurugan et al. Citation2019) by replacing the Gaussian envelope with another symmetric bell-shaped function with a simpler analytical expression. Five parameters used to define the pulse are determined using Zhang et al.(Citation2020) and represent the pulse extracted in this study (). The five pulse parameters are as follows,
Figure 5. Extracted pulse portion of ground motion (NFPL 2) (‘Recorded’ indicates the natural ground motion and ‘pulse’ represent the extracted pulse).

Peak velocity A, which controls the amplitude of the wavelet;
Pulse period Tp of the harmonic oscillation of the wavelet;
Pulse duration γ of the wavelet, which measures the number of oscillations;
Phase ϕ of the amplitude–modulate harmonic (i.e. it can either be a symmetric or antisymmetric signal through setting ϕ = 0 and ϕ =
π/2, respectively).
An epoch of the envelope’s peak T0.
(3)
(3)
3. Test result analysis
The dynamic response of rock mass slopes exposed to NFPL seismic waves is a major scientific problem (Buah et al. Citation2022) The time-domain procedure can be used to reveal the dynamic response characteristics and failure mechanism of slope from multiple perspectives. Therefore, it is adopted alongside Flac 3 D to explore the influence of different ground motions on the dynamic response characteristics of a homogenous rock mass slope in this study. SeismoSignal. (Citation2021) is used for baseline correction and band band-pass filtering before the time domain analysis.
3.1. Wave propagation characteristics of the layered slope
illustrates the acceleration distribution from the bottom to the crest of a rock mass slope exposed to seismic wave excitation. This is done to demonstrate the impact of slope on wave propagation characteristics. The NFPL 2 input wave (vertical direction) is used as an example. The acceleration result from the calculation considers the initial stress due to gravity. There is a layered wave propagation characteristic through the rock mass (), where an acceleration phase shift from the bottom of the slope to the crest can be identified. This acceleration phase shift within the slope symbolizes an amplification effect ().
3.2. Amplification factors
The PGA and PGV at each monitoring point on the slope surface must first be determined from the dynamic analysis acceleration and velocity time history, respectively (Zhang et al. Citation2013; Zhou et al. Citation2019; Song et al. Citation2021). The PGA and PGV obtained at various points on the slope surface may significantly differ. The PGA and PGV at the slope toe (P1) are slightly smaller than the PGA at all other points (P2–P7) (). This indicates that the PGA and PGV for slopes under seismic waves positively correlate with elevation, confirming Sun et al. Citation2021). The PGA and PGV amplification factors (MPGA) and (MPGV) at each point on the slope surface are determined by the ratio of the PGA and PGV at that point under consideration (using P2, for example) to the selected reference point (usually the point at the slope toe, P1). This is expressed mathematically as in EquationEqs. (4)(4)
(4) and Equation(5)
(5)
(5) .
(4)
(4)
(5)
(5)
Figure 7. Acceleration and velocity-time history of a natural pulse-like earthquake loading (NFPL 2) indicating PGA and PGV amplification effect.

represents the acceleration and velocity distribution after dynamic analysis. There is a clear difference between the PGA and PGV at P1 (lowest point) and P7 (highest point) on the slope. The PGA and PGV values at P7 are much higher than their correspondence at other lower points (P6 to P1). confirms that structural planes exhibit an amplification effect on wave propagation characteristics when exposed to seismic waves. The amplification effect is usually proportional to elevation, although the propagation wave’s characteristics usually show an uneven characteristic depending on the material composition of the structural plane.
3.3. Amplification magnification
The magnification change rule must be exploited in determining the dynamic acceleration response of a slope under seismic waves. The acceleration magnification change rule is a plot of the MPGA of any monitoring point (P1–P7) to its corresponding relative height (h/H) on the slope surface. The relative height (h/H) is defined as the ratio of the height of the monitoring point (h, measured from the toe of the slope model) to the total height (H) of the slope model.
The MPGA change rule to relative height is determined for NFPL ground motion (), NFNPL ground motion (), FFNPL ground motion (), and () the average for the selected ground motions. It can be noticed from that MPGA of the NFPL seismic waves increases gradually and steadily with the h/H until it reaches halfway through the total height (h/H > 0.5). After that, the increase becomes rapid until the maximum point (MPGAmax) is reached. Compared to the non-pulse-like ground motions, the NFPL ground motion exhibits higher magnification effects (). However, the PGA for all the ground motions was modulated (have the same PGA, ) prior to the numerical simulation.
Figure 8. Variations in PGA amplification factor for each monitoring point on the slope surface under seismic loading (a) Five NFPL (b) Five NFNPL (c) Five FFNPL (d) Average NFPL, NFNPL, and FFNPL.

The magnification change rule is exploited in determining the dynamic velocity response of the model slope under seismic waves. A plot of the MPGV at any monitoring point (P1–P7) to its corresponding relative height (h/H) on the slope surface is used to establish the velocity amplification magnification change rule (). The MPGV increases with the relative elevation of the slope up to the maximum point (MPGVmax), indicating that the PGV of the slope under seismic wave also has an elevation amplification effect. The MPGV change rule to h/H is determined for NFPL ground motion (), NFNPL ground motion (), FFNPL ground motion (), () the average for the selected ground motions, and CH01 (0.1 g used for validation). The average NFPL ground motion has a Maximum amplification Factor (MPGVmax) of 2.45 (representing 42%), the MPGVmax of NFNPL ground motion is 1.95 (representing 32.7%), and the MPGVmax of the FFNPL ground motion is 1.55, (representing 26%). This indicates that the structural response to NFPL ground motion is 9.3%, stronger than the NFNPL and 16% higher than the FFNPL.
3.4. Slope surface displacement
The Slope surface displacement for the slope subjected to NFPL, NFNPL, and FFNPL ground motions is shown in . The displacement plot is made from residual post-earthquake values for the fifteen ground motions records used for this study. The displacement response of the homogenous slope first increases, decreases, and increases again with the relative elevation. This is explained by the discontinuity nature of the rock mass, wave refraction, and reflection affects the slope surface during its interaction with seismic waves. However, it’s noticed from that the displacement plot under NFPL ground motion is 20% higher than NFNPL ground motions.
Figure 10. Slope surface displacement (a) Five NFPL, (b) five NFNPL, (c) Five FFNPL, (d) Average NFPL, NFNPL, and FFNPL.

Seismic-induced displacement profile of the slope is determined by Peak ground displacement (PGD) Nephograms. Two NFPL, two NFNPL, and two FFNPL horizontal seismic loads are used as examples. shows the PGD distribution Nephograms of the seismic loads obtained from computing the PGD values at all monitoring points on the slope’s surface. The PGD distribution is similar for NFPL, NFNPL, and FFNPL seismic loads, where displacement linearly increases with slope elevation, indicating that PGD is positively correlated with slope elevation.
3.5. Fourier response analyses
The influence of NFPL ground motion on catastrophic landslides is further determined using Fourier spectrum analysis. NFPL (NFPL 2) and NFNPL (NFNPL 10) ground motions are used to analyze the amplification effect of the slope model. The predominant frequencies of the ground motions are determined (). NFPL 2 had f2, f4, and f5 as predominant Frequencies (). The Peak Fourier Spectra Amplitudes (PFSA) progressively increased with the relative slope elevation. This shows that Fourier Spectrums are closely related to the amplification magnification of a slope exposed to seismic waves.
Figure 12. Fourier amplitude of different measuring points on the slope surface (a) NFPL 2 (b) NFPL10, PFSA dynamic response for (c) NFPL 2, (d) NFNPL 10.

The dynamic response of the slope under both seismic loads is represented with a PFSA and h/H change rule (). The PFSA of the NFNPL ground motions has an irregular progressive increase with the h/H of the slope up to the maximum point (slope crest).
Under the NFNPL ground motion change rule, a rapid increase is witnessed from the start point (P1 on the slope surface) up to h/H > 0.5. The increase then becomes gradual up to h/H > 0.82 before it decreases and increases again ().
Under the NFPL ground motion, the increase was much more regular from the starting point up to the slope crest (highest point) without any decrease ().
The difference in the amplification magnification plot for the NFPL and NFNPL ground motions is because NFNPL seismic loads are not destructive compared to the NFPL seismic loads. Therefore, NFNPL seismic load cannot reach the highest point of the slope causing a more vigorous dynamic response and subsequent damage to the structural plane (slope) compared to the NFPL seismic loads.
3.6. Influence of input motion frequency
From numerical simulation results, a simultaneously generated SV and P wave get reflected when SV waves are incident on homogenous slopes (Citation2022). The influence of the frequency of the input seismic wave on the dynamic response of the model slope is analyzed. The model slope in (using ten monitoring points) is evaluated using material properties in under assumed seismic frequencies 5 Hz, 10 Hz, and 15 Hz (). The MPGA for 5 Hz reaches 3.1 at the slope crest. This makes 5 Hz, the natural frequency of the slope structure. The MPGA for 10 Hz increases first, then decreases and increases again. The increase under 15 Hz frequency is almost identical to the 10 Hz (but relatively lower). This shows that under the same condition (geometry and material), different input seismic frequencies exhibit different characteristics, as in . Because different seismic input characteristics present different seismic responses, the lower the frequency, the higher the response. This shows the effect of seismic frequency and must be factored into dynamic response analysis.
Figure 13. Frequency (a) Incoming Sv wave propagation on the slope Surface and the generated SV waves, P waves, and Rayleigh waves (Qi et al. Citation2022). (b) MPGA along slope surface with different input frequencies.

4. Slope failure process
Practical methods to evaluate the seismic stability of slopes include the dynamic time history, dynamic strength reduction, and pseudo-static methods. The strength reduction method is used to evaluate the failure surface of the slope under static conditions using earthquake ground motion (CH01) in this study. The strength reduction method is applied in progressively calculating the Factor of safety (Fs) by reducing the material’s strength to a limit equilibrium state (Qi et al. Citation2022). This method is employed in Flac 3 D using the model slope () and the material properties (). written mathematically as
(6)
(6)
where c is the cohesion, ϕ is the friction angle, and σt is the tensile strength. c′, ϕ′, and σt are the reductions of the cohesion, friction angle, and tensile strength, respectively. k′ is the reduction coefficient. The value of k′ indicates the Fs of the slope, where under a certain reduction condition k′ reaches a state of failure.
The potential slip surface of the model slope is determined by applying natural earthquake waves at the bottom (). The slope’s static and dynamic failure is determined using the CH01 seismic wave, the model slope in , and the material properties in . The result shows that the static and dynamic failure are nearly consistent, the slope failure occurs at the surface, where the materials are sometimes exposed and loose (). The dynamic failure surface is deeper than the static and occurs progressively with time. The surface failure starts with strain formation at the slope toe (because wave propagation starts from the bottom), strain accumulation, and concentration at the middle of the slope. Formation of tensile strains at the crest of the slope where sliding failure finally initiates depending on the slope’s topography, material properties, seismic wave, and time (). is an illustration of the cloud diagram obtained from the dynamic failure of the model slope determined under NFPL, NFNPL and FFNPL ground motions. The cloud diagrams show deeper failure under NFPL ground motions compared with the NFNPL and FFNPL ground motions.
Figure 14. Dynamic and static Failure surface of slope under CH01 earthquake ground motion in Flac 3 D at time (t) (a) t = 0.8 s, (b) t = 5.7 s, (c) t = 10 s, (d) t = 20 s.

Figure 15. Permanent displacement cloud diagram of natural earthquake loadings (a) NFPL, (b) NFNPL, and (c) FFNPL obtained from Flac 3 D.

4.1. Comparing dynamic failure in Flac 3 D and Newmark-type displacement
The dynamic displacement of the rock mass () under five NFPL ground motions obtained using the time-domain procedure is compared with displacement obtained using the Newmark-type sliding block methods (Newmark Citation1965; Jibson Citation2011). SLAMMER (Seismic Landslide Movement Modeled using Earthquake Records) is used to compute the Sliding block displacements (Jibson et al. Citation2013). They range from the most straightforward approach of the original rigid block method of Newmark (Citation1965) to the more sophisticated sliding block method by Zhang et al. (Citation2019)
shows the surface displacement at all monitoring points (P1–P7). The average displacement for the monitoring points is represented by bars in . The orange colored bars represent the failure obtained using the numerical simulation method, and the green ones represent the displacements obtained from the Newmark-type sliding block method. The error margin in the results obtained for Flac 3 D and Newmark’s sliding block method ranges between 1–8%. O the error margin is due to s the difference in ground motions application. The rigid-block approach applies ground motion tangentially to the sliding plane (Newmark Citation1965; Jibson Citation2011); thus, only the tangential acceleration varies. In Flac 3 D, ground motion is applied at the bottom of the model, and variations of both the tangential and normal components of stress occur as the shear wave propagates upward. According to a simple scoping calculation by Song, et al. (Citation2021) the Newmark displacements tend to increase if both the tangential and normal components are considered
Figure 16. Comparison of Flac 3 D displacements with Newmark-type methods (i.e. rigid block) (a) NFPL1-NFPL7 ground motion displacement for all monitoring points on the slope surface from Flac 3 D against Newmark type displacement at each point on the slope surface (b) Average displacement for each NFPL ground motion in Flac 3 D against average Newmark-displacement for each NFPL ground motion.

5. Conclusion
The seismic response of a homogenous rock mass slope subjected to vertically propagating seismic wave is investigated in this study. The model slope is numerically simulated with different seismic waves using the finite difference code Flac 3 D to determine its dynamic response and failure properties. The MPGA, MPGV, surface displacement, and dynamic failure are analyzed, and some significant findings are concluded according to the results as follows;
Structural plains profoundly influence wave propagation characteristics through them. This makes material properties, mesh size, and model slope boundary essential factors for consideration in dynamic analysis.
The slope surface’s MPGA, MPGV, and displacement increase with an elevation under all ground motions (i.e. NFPL, NFNPL, and FFNPL). The increase is gradual and nonlinear until the maximum point of the slope is reached.
The increase in the MPGA and MPGV is gradual at the lower part of the slope, whiles a rapid increase is noticed at the upper part. This is because the seismic direction of ground motion is closely related to the amplification magnification of the slope. The NFPL ground motion presents a higher dynamic response than NFNPL and FFNPL ground motions. The average MPGV for NFPL is 9.3% higher than NFNPL and 16% higher than FFNPL ground motions.
The influence of seismic waves’ natural frequencies (Hz) on the dynamic response characteristics of the slope is determined. Lower frequencies usually present higher dynamic responses. The NFNL ground motion had a peak predominant frequency of up to 10.1Hz, but the NFPL ground motion, which had a peak predominant frequency of 6.5Hz, produced the highest PFSA dynamic response.
The dynamic failure of a homogenous slope is mainly represented by sliding displacement. The failure happens due to strains built up on the slope surface from the impact of the seismic wave to which it is subjected. The accumulated strain forces the slope crest to lose strength, leading to its subsequent failure. The test result analysis shows that NFPL ground motion is a high inducer of slope failure compared to NFNPL and FFNPL ground motions and presented an average 20% surface displacement higher than non-pulse ground motions.
The result of this study can be factored into reinforcing seismic engineering design and probabilistic stability analysis. However, there are also some limitations. The time–domain analysis method only considers the acceleration time-history characteristics and ground motion amplitude, therefore appropriate for simple structures. The dynamic response characteristics of complex geological structures need to be studied using the frequency-domain method.
Data availability statement
The authors confirm that the data supporting the findings of this study are available within the article [and/or] its supplementary materials.
Disclosure statement
The authors have no relevant financial or non-financial interests to disclose.
Additional information
Funding
References
- Adanur S, Altunişik AC, Bayraktar A, Akköse M. 2012. Comparison of near-fault and far-fault ground motion effects on geometrically nonlinear earthquake behavior of suspension bridges. Nat Hazards. 64(1):593–614.
- Assimaki D, Gazetas G, Kausel E. 2005. Effects of local soil conditions on the topographic aggravation of seismic motion: parametric investigation and recorded field evidence from the 1999 Athens earthquake. Bull Seismol Soc Am. 95(3):1059–1089.
- Baker JW. 2007. Quantitative classification of near-fault ground motions using wavelet analysis. Bull Seismol Soc Am. 97(5):1486–1501.
- Bao Y, Huang Y, Zhu C. 2021. Effects of near-fault ground motions on dynamic response of slopes based on shaking table model tests. Soil Dyn Earthq Eng. 149(May 2020):106869.
- Buah PA, Zhang Y, He J, Xiang C, Bakah DAY. 2022. Study on the seismic response of slope under pulse-like ground motion. Int Geotech J Eng. 16(9):105–109.
- Champion C, Liel A. 2012. The effect of near-fault directivity on building seismic collapse risk. Earthq Engng Struct Dyn. 41(10):1391–1409.
- Chanerley AA, Alexander NA. 2010. Obtaining estimates of the low-frequency “fling”, instrument tilts and displacement timeseries using wavelet decomposition. Bull Earthq Eng. 8(2):231–255.
- Chanerley AA, Alexander NA, Berrill J, Avery H, Halldorsson B, Sigbjornsson R. 2013. Concerning baseline errors in the form of acceleration transients when recovering displacements from strong motion records using the undecimated wavelet transform. Bull Seismol Soc Am. 103(1):283–295.
- Chen Z, Song D. 2021. Numerical investigation of the recent Chenhecun landslide (Gansu, China) using the discrete element method. Nat Hazards. 105(1):717–733.
- Chiou B, Darragh R, Gregor N, Silva W. 2008. NGA project strong-motion database. Earthq Spectra. 24(1):23–44.
- Chowdhury I, Dasgupta SP. 2003. Computation of Rayleigh damping coefficients for large systems. Electron J Geotech Eng. vol. 8C:2–10.
- Gao W, Wang X, Dai S, Chen D. 2016. Numerical study on stability of rock slope based on energy method. Adv Mater Sci Eng. 2016:1–10.
- Gischig VS, Eberhardt E, Moore JR, Hungr O. 2015. On the seismic response of deep-seated rock slope instabilities - insights from numerical modeling. Eng Geol. 193:1–18.
- Zhang Y, Yu P, Zhao L, Cheng Q, Yuan R. 2015. A new energy-based pulse index for quantitative identification of near-fault pulse-like ground motions. Msc Thesis SWJTU - Chengdu (1), 3949.
- He J, Fu H, Zhang Y, Wan A. 2022. The effect of surficial soil on the seismic response characteristics and failure pattern of step-like slopes. Soil Dyn Earthq Eng. 161(December 2021):107441.
- He J, Qi S, Zhan Z, Guo S, Li C, Zheng B, Huang X, Zou Y, Yang G, Liang N. 2021. Seismic response characteristics and deformation evolution of the bedding rock slope using a large-scale shaking table. Landslides. 18(8):2835–2853.
- Itasca Consulting Group. 2017. FLAC3D 6.0 Modelling. 405.
- Jibson RW. 2011. Methods for assessing the stability of slopes during earthquakes - a retrospective. Eng Geol. 122(1–2):43–50.
- Jibson RW, Rathje EM, Jibson MW, Lee YW. 2013. SLAMMER: Seismic LAndslide Movement Modeled using Earthquake Records. November 2014.
- Kalkan E, Kunnath SK. 2006. Effects of fling step and forward directivity on seismic response of buildings. Earthq Spectra. 22(2):367–390.
- Keeper DK. 1984. Geological Society of America Bulletin Landslides caused by earthquakes. Geol Soc Am. 4:406–421.
- Khanbabazadeh H, Iyisan R, Ozaslan B. 2022. 2D seismic response of shallow sandy basins subjected to obliquely incident waves. Soil Dyn Earthq Eng. 153(November 2021):107080.
- Kohrangi M, Vamvatsikos D, Bazzurro P. 2019. Pulse-like versus non-pulse-like ground motion records: spectral shape comparisons and record selection strategies. Earthqu Eng Struct Dyn. 48(1):46–64.
- Li H, Liu Y, Liu L, Liu B, Xia X. 2019. Numerical evaluation of topographic effects on seismic response of single-faced rock slopes. Bull Eng Geol Environ. 78(3):1873–1891.
- Ma Z, Liao H, Dang F, Cheng Y. 2021. Seismic slope stability and failure process analysis using explicit finite element method. Bull Eng Geol Environ. 80(2):1287–1301.
- Mase LZ, Likitlersuang S, Tobita T. 2018. Non-linear site response analysis of soil sites in northern Thailand during the Mw 6.8 tarlay Earthquake. EJ. 22(3):291–303.
- Mase LZ, Likitlersuang S, Tobita T. 2022. Verification of liquefaction potential during the strong earthquake at the border of Thailand-Myanmar. J Earthq Eng. 26(4):2023–2050.
- Mase LZ, Likitlersuang S, Tobita T, Chaiprakaikeow S, Soralump S. 2020. Local site investigation of liquefied soils caused by earthquake in Northern Thailand. J Earthq Eng. 24(7):1181–1204.
- Mavroeidis GP, Papageorgiou AS. 2010. Effect of fault rupture characteristics on near-fault strong ground motions. Bull Seismol Soc Am. 100(1):37–58.
- Mavroeidis GP, Papageorgiou AS. 2003. A mathematical representation of near-fault ground motions. Bull Seismol Soc Am. 93(3):1099–1131.
- Newmark NM. 1965. Effects of earthquakes on dams and embankments. Geotechnique. 15(2):139–160.
- Nguyen TS, Likitlersuang S, Jotisankasa A. 2020. Stability analysis of vegetated residual soil slope in Thailand under rainfall conditions. Environ Geotech. 7(5):338–349.
- Ongpaporn P, Jotisankasa A, Likitlersuang S. 2022. Geotechnical investigation and stability analysis of bio-engineered slope at Surat Thani Province in Southern Thailand. Bull Eng Geol Environ. 81:84.
- Petchkaew P, Keawsawasvong S, Tanapalungkorn W, Likitlersuang S. 2021. 3D stability analysis of unsupported rectangular excavation under pseudo-static seismic body force. Geomech Geoeng. 0(0):1–18.
- Qi S, He J, Zhan Z. 2022. A single surface slope effects on seismic response based on shaking table test and numerical simulation. Eng Geol. 306(June):106762.
- Seismosoft. 2021. Seismosignal Software. Pavia, Italy. https://seismosoft.com/product/seismosignal/.
- Somerville PG, Graves RW. 2003. Characterization of earthquake strong ground motion. Pure Appl Geophys. 160(10–11):1811–1828.
- Somerville PG, Smith NF, Graves RW, Abrahamson NA. 1997. Modification of empirical strong ground motion attenuation relations to include the amplitude and duration effects of rupture directivity. Seismol Res Lett. 68(1):199–222.
- Song D, Chen Z, Dong L, Zhu W. 2021. Numerical investigation on dynamic response characteristics and deformation mechanism of a bedded rock mass slope subject to earthquake excitation. Appl Sci (Switzerland). 11(15):7068.
- Standard for Engineering Classification of Rock Masses (GB 50218-94). 1994. General administration of quality supervision, inspection, and quarantine of the People’s Republic of China. (In Chinese).
- Sun W, Yan S, Ma Q, Liang Q, Ou E, Cao X, Wang J, Luo X. 2021. Dynamic response characteristics and failure mode of a bias loess tunnel using a shaking table model test. Transp Geotech. 31(September):100659.
- Tsai HY, Tsai CC, Chang WC. 2019. Slope unit-based approach for assessing regional seismic landslide displacement for deep and shallow failure. Eng Geol. 248(January 2018):124–139.
- Tsai KC, Hsiao CP, Bruneau M. 2000. Overview of building damages in 921 Chi-Chi earthquake. Earthq Eng Eng Seismol. 2(1):93–108.
- Velmurugan S, Mahabub Basha A, Vijayakumar M. 2019. Gabor wavelet multi-linear discriminant analysis for data extraction in ECG signals. Cluster Comput. 22(s6):14219–14229.
- Wu Y, Ke Y, Chen Z, Liang S, Zhao H, Hong H. 2020. Application of alternating decision tree with AdaBoost and bagging ensembles for landslide susceptibility mapping. Catena. 187(November 2019):104396.
- Xu C, Xu X, Yu G. 2013. Landslides triggered by slipping-fault-generated earthquake on a plateau: an example of the 14 April 2010, Ms 7.1, Yushu, China earthquake. Landslides. 10(4):421–431.
- Yang B, Hou J, Liu Y, Zhou Z. 2021. Dynamic response and failure characteristics of slope with weak interlayer under action of near-fault ground motion. Shock Vib 2021:1–18.
- Yin Y, Wang F, Sun P. 2009. Landslide hazards triggered by the 2008 Wenchuan earthquake, Sichuan, China. Landslides. 6(2):139–152.
- Zhang J, Zhang M, Li M, Min Q, Shi B, Song L. 2020. Nonlinear dynamic response of a CC-RCC combined dam structure under oblique incidence of near-fault ground motions. Appl Sci (Switzerland). 10:3–15.
- Zhang S, Wang G. 2013. Effects of near-fault and far-fault ground motions on nonlinear dynamic response and seismic damage of concrete gravity dams. Soil Dyn Earthq Eng. 53:217–229.
- Zhang S, Wang G, Sa W. 2013. Damage evaluation of concrete gravity dams under mainshock-aftershock seismic sequences. Soil Dyn Earthq Eng. 50:16–27.
- Zhang Yb, Xiang Cl, Chen Yl, Cheng Qg, Xiao L, Yu Pc, Chang Zw. 2019. Permanent displacement models of earthquake-induced landslides considering near-fault pulse-like ground motions. J Mt Sci. 16(6):1244–1257.
- Zhao WS, Chen WZ. 2015. Effect of near-fault ground motions with long-period pulses on the tunnel. J Vibroeng. 17(2):841–858.
- Zhou Z, Ren C, Xu G, Zhan H, Liu T. 2019. Dynamic failure mode and dynamic response of high slope using shaking table test. Shock Vib. 2019:1–19.
- Zou D, Han H, Liu J, Yang D, Kong X. 2017. Seismic failure analysis for a high concrete face rockfill dam subjected to near-fault pulse-like ground motions. Soil Dyn Earthq Eng. 98(October 2016):235–243.