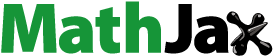
Abstract
In multi-hole blasting, the design of the initiation pattern is critical for the blasting effectiveness, but its effects on rock damage and blasting seismic are still unclear. This research focused on investigating the effect of initiation patterns under multi-hole blasting using numerical simulations and in-situ blasting experiments. First, the rock damage mode and blasting seismic characteristics under four initiation patterns were numerically studied. The rock damage degree was 19.26%, 13.42%, 17.06%, and 9.17% for the four initiation patterns, respectively. Correspondingly, the peak particle velocity (PPV) at monitoring point A reached 2.90 m/s in Case 1, but only 1.56–1.69 m/s in the other three cases. The results indicated the simultaneous initiation pattern causes more severe damage to the rock between blastholes and enhances blasting seismic effect. Furthermore, when the blasthole with a big explosive charge was preferentially initiated, the outcome of rock damage was improved. In-situ blasting experiments with various initiation patterns were conducted in a coal mine, and the blasting seismic was measured. The blasting seismic intensity is closely related to the blastholes number, explosive charge and spacing of blastholes. The simultaneous initiation pattern enhances both the PPV and source energy of blasting seismic, potentially causing rock bursts in the underground roadway. Finally, the practicable initiation pattern of deep-hole blasting in the coal mine was suggested.
1. Introduction
Deep-hole blasting was widely used in roof rock masses to mitigate the stress concentration, and then reduce the potential of rock bursts or strong underground pressure behaviours in underground coal mines (Konicek et al. Citation2013; Konicek and Waclawik Citation2018; Dou et al. Citation2022). According to engineering practices, a group of blastholes presents the fan-shaped, consisting of two to four cylindrical holes with different incline angles and lengths (Qi et al. Citation2007; Dou et al. Citation2020). The design of the initiation pattern, such as sequential or simultaneous initiation, is critical in multi-hole blasting. It has been a challenge for technicians in engineering applications. The challenge was primarily driven by the contradiction between the desired rock blasting quality, undesired blasting seismic, as well as the efficiency and cost of blasting. To be specific, when a group of blastholes were initiated simultaneously, more adequate rock blasting damage may be achieved, so as to mitigate the stress concentration to a greater extent. Moreover, it can avoid some repetitive blasting procedures, which improves blasting efficiency and lowers operation costs. However, the existing research revealed that part of the explosive energy was converted into seismic waves, which may cause damage to the underground roadway and its support structure (Sanchidrián et al. Citation2007; Lurka et al. Citation2021). As a result, the adverse effect of blasting seismic should be minimized as much as possible. The blasting damage is usually positively correlated with blasting seismic intensity, which means that the simultaneous initiation pattern is not conducive to blasting seismic control. Conversely, the sequential initiation pattern may effectively reduce the seismic intensity, but the rock damage may be limited. Moreover, the blasting efficiency decreases and the operation cost increases. For these reasons, the technicians must make a good compromise between them and design a practicable initiation pattern for deep-hole blasting.
Many fruitful studies on rock damage and vibration effect subjected to blasting load have been performed. Numerical simulation has been an efficient tool to explore the blasting failure mechanism of rock. Zhu et al. (Citation2007) investigated the dynamic fracture mechanism and crack propagation of rock using the AUTODYN 2D code. Then, they discussed the effect of the boundary condition, coupling medium, borehole diameter, decoupling and joint on rock dynamic fracture. Ning et al. (Citation2011) used discontinuous deformation analysis (DDA) to simulate the blast-induced rock mass failures and reproduce the whole blasting process. Ma and An (Citation2008) implemented the Johnson-Holmquist (J-H) material model into the LS-DYNA software to study the blasting-induced rock fractures, and then discussed the effect of some factors on the rock fracture pattern. Moreover, many researchers studied the effect of in-situ stress on rock failure under blasting load (Tao et al. Citation2020; Yi et al. Citation2018; Yilmaz and Unlu Citation2013). Their findings suggested that in-situ stress limits rock crack propagation, and the anisotropy of in-situ stress also greatly influences crack propagation. Xie et al. (Citation2016, Citation2017) studied the influence of in-situ stress on the cut blasting results using numerical calculations. The results indicated that in-situ stress and anisotropy impose resistance on rock damage. Then, they proposed an optimization method for cutting blasting designs to solve the difficulties in cutting blasting. Further, Wang et al. (Citation2021) discussed the influence of in-situ stress on cumulative rock damage under repeated blasting. They pointed out that pre-split blasting can solve the under-breaking phenomenon caused by in-situ stress. In addition to the influence factor of in-situ stress, the charge structure and the initiation location also greatly change the rock damage mode. Pan et al. (Citation2022), for example, used numerical simulations to investigate the rock damage mode in blasting with an eccentric decoupled charge. They found a distinct pressure eccentricity effect in the eccentric charge blasting, and then the blasting parameters were optimized to obtain a better blasting effect. Gao et al. (Citation2019) focused on the influence of initiation location on explosion energy transmission. The results indicated that the priority transmission direction of explosion energy is consistent with the orientation of the detonation wave. Leng et al. (Citation2016) studied the rock damage results under different initiation patterns, and the results showed that side initiation was more effective in soft and fissured rocks. Li et al. (Citation2021) studied the influence of coupling mediums on explosion energy transmission. They concluded that, compared with air-coupling blasting, a higher explosion energy transmission efficiency can be obtained in water-coupling blasting. Hu et al. (Citation2014) compared the spatial distribution of the rock damage zone under smooth blasting and pre-split blasting. They found that compared to presplit blasting, two classes of damage zone could be found in smooth blasting excavation. Zhang et al. (Citation2023) studied the distribution of blasting vibration velocity in the side walls of underground cavities and deduced a predictive formula for the PPV considering the in-situ stress.
For multi-hole blasting, more factors may influence the blasting damage and vibration effects of rock mass, such as the number of blastholes, blasthole arrangement, delay time, and blasthole spacing. Many researchers aimed at these issues and obtained many fruitful results. For example, Yue et al. (Citation2022) investigated the effect of three factors on the damage and vibration of rock in multi-hole blasting, including the blasthole arrangement, delay time, and charge structure. Zhao (Citation2021) and Zuo et al. (Citation2019) investigated the rock damage zone distribution under three blastholes layouts. The results show ‘deep-shallow-fractal’ blasthole layout causes more developed plastic damage zones and more uniform rock fragmentation. Tang et al. (Citation2022) carried out eight experiments on bench-blasting models with double holes to investigate the influence of delay time on rock fragmentation. Kan, Dou, Li, Cao, et al. (Citation2022) studied the influence of charge distribution design, spacing of blastholes and propagation path on rock vibration attenuation. Chen et al. (Citation2015) discussed the effect of millisecond time, charge length and detonation velocity on rock vibration in multi-hole blasting. The results indicated that blasting vibration superposition will occur due to the difference in propagation velocity of explosion stress wave, which can enlarge the PPV of rock vibration. Zhang et al. (Citation2022) explored the effect of different delay times on blast-induced PPV by multi-hole blasting experiments, and the PPV first decreases then increases and finally attenuates to the single-hole PPV as the initiation delay time increases. Moreover, engineering practices suggested that the number of simultaneous initiation holes and the initiation sequence between blastholes also have a considerable influence on rock blasting damage and blasting seismic effects. However, there is little literature focusing on the effect of initiation patterns in multi-hole blasting.
In this research, the effect of initiation pattern on rock damage and blasting seismic under multi-hole blasting was investigated using numerical simulations and in-situ blasting experiments. First, the rock blasting damage and blasting seismic characteristics under four initiation patterns were numerically investigated by LS-DYNA software. The influence mechanisms of initiation pattern on rock blasting damage and blasting seismic were obtained. Based on the numerical results, in-situ blasting experiments with different initiation patterns were practiced in a coal mine to further verify the effects of initiation patterns on blasting seismic intensity. Then, considering the optimal blasting damage effect and acceptable blasting seismic intensity, practicable initiation patterns for multi-hole blasting were suggested in the coal mine. The research findings can provide a reference for the design of the initiation pattern of deep-hole blasting in coal mines with similar conditions.
2. Constitutive model parameters and validation for blasting simulation
2.1. RHT model and its parameters for rock
The LS-DYNA software has been widely used to simulate rock blasting. In general, the numerical model of rock blasting mainly consists of rock medium, explosive, air and stemming medium. Three constitutive models, including the HJC model, the JH series model, and the RHT model in LS-DYNA were used to describe the rock damage subjected to blast loading (Borrvall and Riedel Citation2011; Johnson and Holmquist Citation1994; Bi Citation2018). Among which, the RHT model, proposed by Riedel et al. was verified to perform better in rock blasting simulation than other constitutive models (Riedel et al.Citation1999). Therefore, the RHT model was selected as the constitutive model for rock masses in this research.
In the RHT model, three limit surfaces are embedded to describe the strength of the material, as shown in . It includes the yield surface, the failure surface and the residual surface. First, the material begins to deform plastically when the stress is beyond the yield surface. As the stress reaches the failure surface, the damage to the material starts to develop. The material is fully damaged when the stress reaches the residual surface. The damage degree Da of the material was described by the accumulated plastic strain
(1)
(1)
where
is failure strain of the material.
For more details on the RHT model, please refer to Borrvall and Riedel (Citation2011).
In the RHT model, a total of 37 necessary parameters need to be determined. Among these, three basic parameters can be obtained directly from physical and mechanical experiments on rock samples, including rock density, elastic shear modulus and uniaxial compressive strength. Then, 10 parameters were determined based on the basic experiment data, including the strain rate dependence exponent, the Hugoniot polynomial coefficient, the parameter for polynomial EOS, and the reference compressive/tensile strain rate. For the detailed determination method of the above ten parameters, please refer to Wang et al. (Citation2021) and Huo et al. (Citation2021). The remaining parameters were difficult to determine directly. Those parameters sensitive to the simulation results, including three limit surface parameters and relative shear strength, were determined by trial and error. Then, those parameters insensitive to the result were directly taken from Borrvall’s literature. The determined parameters for the RHT model are listed in .
Table 1. Determined parameters of RHT model.
2.2. Material and parameters of explosive, air and stemming
The explosive was simulated by the high explosive burn material with the Jones-Wilkins-Lee (JWL) equation of state in LS-DYNA. The JWL equation of state was expressed as follows (Tawadrous Citation2010):
(2)
(2)
where PJ is the pressure, A, B, R1, R2, and ω are the JWL parameters, E is the internal energy, and V is the relative volume of the detonation product. The parameters of explosive and JWL EOS were cited from Sun et al. (Citation2017), as presented in .
Table 2. Parameters for the emulsion explosive.
The air material and stemming material were modelled by MAT_NULL and MAT_SOIL_AND_FOAM in LS-DYNA, respectively. The parameters of stemming material were selected from Wang (Citation2015), as listed in .
Table 3. Parameters for the stemming material.
2.3. Validation of the RHT model
To investigate the accuracy and applicability of the RHT model and its parameters, a numerical model for rock samples was developed (see ). A cubic rock model with a length of 100 mm was established, and a cylindrical hole with 3.0 mm in radius and 40 mm in length was drilled in the centre of the rock model. The charge length and stemming length are 10 mm and 30 mm, respectively. An uncoupled charge structure with a 5 mm explosive diameter was used. Then, the numerical simulation results were compared with the experimental results of (Banadaki and Mohanty Citation2012). As seen in , after the detonation of the explosive, the compression crushing zone, radial cracks and circumferential cracks can be distinctly observed in the radius direction of the blasthole. The numerical results match well with the experimental results of Banadaki, as shown in . Moreover, presents the damage results in the section plane along the columnar blasting hole, and the rock damage around the blasting hole shows a funnel shape. Therefore, overall, the determined RHT model parameters in this research are feasible.
3. Influence of initiation pattern on rock damage and blasting seismic under multi-hole blasting
3.1. Design of numerical simulation cases
In coal mines, a group of deep-hole blasting for mitigating rock bursts was composed of two to four inclined cylindrical blastholes with different incline angles and lengths. A typical blastholes group layout of deep-hole blasting is shown in . As mentioned earlier, there is always a conflict between ideal rock blasting damage, undesirable blasting seismic and blasting efficiency and cost. Then, technicians must choose a practicable initiation pattern, such as sequential or simultaneous initiation, when detonating explosives in multi-hole blasting. Therefore, a simplified physical model was built and four simulation cases were designed to investigate the effects of initiation patterns. Considering the numerical computing capability and accuracy, the model was built as a 3D 1/2 model with 14.0 m in length, 10.0 m in height and 5.0 m in width (see ). Three blastholes with diameters and lengths of 120 mm and 6.0 m, respectively, were drilled in this model, and the blasthole includes the charge section and the stemming section. The charge section length of each blasthole is 3.0 m (1# blasthole), 2.0 m (2# blasthole) and 1.0 m (3# blasthole), respectively. The spacing of each blasthole is 3.0 m. Boundary conditions were applied on the model surface to eliminate boundary effects.
Based on this physical model, four cases were studied as follows:
Case 1: three blastholes were initiated simultaneously.
Case 2: three blastholes were initiated individually, and according to the sequence of 1#, 2# and 3# blasthole. In this case, the full restart method in LS-DYNA will be applied to investigate the rock cumulative blasting damage.
Case 3: the 1# blasthole was first initiated individually, and then two blastholes (2# and 3#) were initiated simultaneously.
Case 4: switching the order of two rounds of blasting in Case 3, which means two blastholes (2# and 3#) were first initiated simultaneously, and then the 1# blasthole was initiated individually.
3.2. Influence of initiation pattern on rock damage under multi-hole blasting
The core purpose of deep-hole blasting is to change the stress environment of stope space by destroying rock structures and then mitigate the risk of coal bursts. As a result, the most critical criterion in determining whether deep-hole blasting was successful is the outcome of the rock blasting damage. illustrates the rock damage results under four initiation patterns. In Case 1, the compressive damage zones were formed around each blasthole wall subjected to blasting shock waves, the damage zones’ size was only three to five times the blasthole’s diameter. Following that, tensile damage zones were created outside the compressive damage zone under the action of tensile stress. Since the explosives were initiated simultaneously in this case, three independent blasting stress waves were generated and superimposed on each other during their propagation. The rock in the areas between the three blastholes suffered the most intense blasting stress wave, resulting in severe rock damage. Therefore, the tensile damage in rock mainly occurs in the areas between the blastholes in this case. Moreover, as the increase of charge length, the rock damage zones will expand.
In Case 2, the rock damage zone was symmetrically distributed along the blasthole after the 1# blasthole was initiated (see ). Then, after 2# blasthole was detonated, the damage zone expanded as a result of the accumulation of rock damage (see ). However, the damage zone was primarily formed in the rock on the left side of 2# blasthole. In essence, the rock in this zone was already damaged after the first blasting. When detonating the 2# blasthole, the stress waves were reflected in the damaged rock and it causes further cumulative damage to the rock. As a result, an obvious damage zone was formed in the vicinity of 2# blasthole. Finally, the 3# blasthole was detonated, and the final damage mode of the rock is shown in . The damage zone developed in the rock next to 3# blasthole, and the damage zones around 1# and 2# blastholes were further extended. However, due to the smaller charge length of 3# blasthole, the rock damage resulting from the initiation of 3# blasthole was limited. Compared to the final damage mode of rock in Case 1, overall, the dimension of the rock damage zone was smaller in Case 2 and the spatial distribution of rock damage was also different. In Case 2, the momentous damage zone focused on the left side of the 2# blasthole, while the rock damage was uniformly distributed in the areas between every two blastholes in Case 1.
In Case 3, the 1# blasthole was initiated first, and the results were the same as in Case 2. Then, the 2# and 3# blastholes were initiated simultaneously, the results are shown in . Compared to the final damage mode of the rock in Case 2, similar damage zone distribution was presented in the rock around the 2# blasthole, and there is a slight increase in the dimension of the rock damage zone. However, new damage zones were formed in the rock between the 2# and 3# blasthole in Case 3, which were caused by the superposition of blasting stress waves generated by the initiation of 2# and 3# blasthole.
In Case 4, the 2# and 3# blastholes were initiated first, and the numerical results are shown in . The results indicated the first rock damage was formed in the vicinity of 2# and 3# blastholes. When the 1# blasthole was initiated, a new damage zone was formed around 1# blasthole, and the first damage zone around 2# and 3# blastholes was further accumulated and extended. The final rock damage mode is presented in . However, compared to Case 3, the results illustrated that the rock damage was significantly reduced, and the damage zones focus on the rock around 1# blasthole.
To quantitative measure the blasting effect, the parameter of rock damage degree (η) was proposed. The index was defined as:
(3)
(3)
where Sd and ST are the areas of damaged element (damage threshold Da = 0.5) and total area of rock element, respectively.
shows the damage degree at the symmetry plane in four cases. There is a distinct difference in the rock damage degree under different initiation patterns. The best rock damage effect can be obtained when the three blastholes were initiated simultaneously. However, when three blastholes were initiated individually, the rock damage quality was not the worst. Instead, the initiation pattern in Case 4 achieved the worst blasting quality. The differences between Case 3 and Case 4 in the rock damage results also illustrate that the initiation sequence in the multi-hole blasting with unequal explosive charge influences the blasting quality. Among them, the initiation pattern in Case 3 achieves a better blasting quality, second only to Case 1. When the blasthole with a higher explosive charge was initiated first, a more developed blast damage zone was generated. The first damage zone is easy to be expanded subjected to the subsequent blasting, resulting in the higher damage quality of rock.
3.3. Influence of initiation pattern on blasting seismic under multi-hole blasting
Since there are other factors to consider when designing deep-hole blasting in underground coal mines, as was noted in the introduction, it is important to keep the undesired blasting seismic effect within acceptable limits. In order to investigate the influence of the initiation pattern on the blasting seismic effect under multi-hole blasting, four monitoring points were selected to extract the rock vibration velocity during the blasting process (see ).
presents the Z-velocity distribution of four monitoring points under different initiation patterns. The PPV value at monitoring point A reached 2.90 m/s in Case 1, compared to 1.56–1.69 m/s in the other three cases, as shown in . Since monitoring point A was located in the middle of hole 1# and hole 2#, when the two blastholes are initiated simultaneously, the rock vibration will be superimposed and enhanced. Similarly, the PPV value of monitoring point B in Case 2 was 1.65 m/s, which was less than that of the other three cases (2# and 3# blastholes are initiated simultaneously). The results indicated that the rock between the blastholes suffered from the superimposed explosion stress under the simultaneous initiation pattern, and the rock vibration was significantly enhanced. This also means that the rocks in these areas suffered stronger damage, which can be verified in . However, the Z-velocity distribution trend of monitoring point C, which is located on the left side of 1# blasthole, was distinct from that of the aforementioned monitoring points. To be specific, the PPV values at monitoring point C in Cases 1–3 were all 0.88 m/s. The main reason is that there is a distance difference between the measuring point C and each blasthole, which leads to a time difference in pressure wave propagation. In essence, the PPV of blasting vibration was caused by the blasting pressure wave generated by the nearest 1# blasthole. Therefore, in these cases, the Z-velocity curves are approximately coincident before the vibration propagation for 1 ms. After 1 ms, when the blasting pressure waves from the farther blastholes reach this location, the stress waves begin to superimpose, which increases the effect of rock vibration. At monitoring point D, a similar vibration velocity distribution also can be observed. Although the PPV value of rock vibration was not improved by the simultaneous initiation pattern, the vibration intensity after the peak vibration was enhanced in the duration. Zhang (Citation2006) pointed out two damage modes of rock subjected to blasting seismic: first overtaking damage and cumulative damage. Among them, the parameter of PPV was generally used to judge whether the first overtaking damage occurs, and total vibration velocity within its duration was used to assess the rock cumulative damage. Therefore, the simultaneous initiation pattern will enhance the cumulative damage effect on the rock at one side of the blasthole. Moreover, the results also show that Case 4 had a higher PPV value at monitoring point C (1.26 m/s) than the other three cases (0.88 m/s). Particularly, only the initiation sequence was reversed in Cases 3 and 4, a greater PPV was obtained in Case 4. Similarly, the PPV value at monitoring point D in Case 3 is 0.77 m/s, which is higher than that in Case 4. This result also indicated that the rock vibration is significantly influenced by the initiation sequence. When the rock was damaged by the first blasting, the rock vibration effect induced by subsequent blasting will be enhanced.
4. In-situ measurement of blasting seismic under different initiation patterns
4.1. Site characterization
Deep-hole blasting experiments with various initiation patterns were carried out in an underground coal mine to verify the effect of initiation patterns obtained from numerical simulations. In this coal mine, the 3–3 coal seam with an average 500 m depth and 4.5 m height was mined. A layer of 25 m thick conglomerate strata covers the coal seam. By the end of 2019, five longwall panels had been mined or planned to be mined, as seen in . Among them, longwall panels (LW) 1101 had been mined, LW 1102 has been suspended after a serious coal burst accident, and LW 1103 was being mined. During the mining process of LW 1103, the expected risk of coal burst exists due to the influence of conglomerate strata. Therefore, roof deep-hole blasting was practiced in the conglomerate strata to mitigate the coal bursts. A group of roof deep-hole blasting in this coal mine is composed of four inclined cylindrical holes. Additionally, a microseismic system was installed and used to pick up the seismic waves induced by various production activities. For more details on this microseismic system, please refer to Cai et al. (Citation2018). In this research, this microseismic system was used to monitor and record the blasting seismic waveforms, and then the software enables data processing so as to provide information about source energy and spatial location of blasting seismic events. Further, the distance between the microseismic sensors and the source position of blasting seismic is the vibration propagation distance. The maximum vibration velocity in the blasting seismic waveform is the PPV parameter.
4.2. Different initiation patterns of deep-hole blasting
A total of five cases were designed to study the blasting seismic effect under different initiation patterns of deep-hole blasting, and the parameters of blastholes and explosives in these five cases are listed in . In Cases 1 and 2, only one blasthole was detonated and the explosives charge was 132 and 240 kg, respectively. Then, simultaneous initiation of multiple blastholes was adopted in other cases. In these cases, the explosive charge of a single hole ranges from 132 to 240 kg, and the total charge is 282, 450 and 732 kg, respectively. Moreover, the explosive used in roof deep-hole blasting is the permissible emulsion explosive for coal mines, and it was initiated by the millisecond delay electric detonator connected to detonating cord.
Table 4. Parameters of blastholes and explosives.
4.3. Attenuation of blasting seismic under different initiation patterns
shows the blasting seismic waveform received by each sensor and the attenuation of the PPV with propagation distance in different blasting cases. These results indicated that the waveforms of blasting seismic events are clear and regular, and the PPV of each waveform can be easily captured. A nonlinear relationship exists between the PPV and propagation distance, which can be described by the following equation:
(4)
(4)
where C and α are the constant coefficients; Di is the propagation distance.
Figure 10. Blasting seismic waveform received by each sensor and attenuation of peak velocity with distance in different blasting cases.
shows the PPV of Case 2 was greater than that of Case 1. As mentioned earlier, both Cases 1 and 2 were initiated with one blasthole, but the explosive charge in Case 2 was 108 kg more than that in Case 1. This indicated that the PPV increases with the explosive charge of the single blasthole. Then, in Case 3, the explosive charge of two blastholes was 132 and 150 kg, respectively. The explosive of a single blasthole was approximately consistent with that in Case 1 and less than that in Case 2. However, the PPV in Case 3 was overall higher than that of the above two cases. The results indicated that the blasting seismic wave generated by each blasthole will be superimposed and enhanced in multi-hole blasting. Particularly, when the spacing between the two blastholes is small, the total explosive charge of all blastholes will become the contributing factor to the PPV. Similarly, the comparison of the results of Cases 3 and 4 also presented an increasing trend as the increase of blasthole number and total charge. However, the PPV in Case 5 was not significantly higher than that in Case 4 and remained approximately consistent. The possible reason is that the charging position of the blasthole with 98 m length was far away from the other three blastholes, and its initiation contributes little to the vibration superposition effect. The results showed that except for the intensity of each independent blasting source, the superposition effect was also influenced by the distance between the independent blasting sources.
The above analysis aimed at the effect of initiation patterns on the PPV of blasting seismic. However, the numerical simulation results in Chapter 3 illustrated that initiation patterns also have a non-negligible impact on the vibration intensity after the peak velocity. As shown in , the blasting seismic waveforms received by the S2 sensor in Cases 4 and 5 were given. The distances between the explosive source and the S2 sensor in two cases are approximate, which are 195 m (Case 4) and 223 m (Case 5), respectively. The PPV values under the two cases are the same, but the vibration intensity after peak vibration is distinctly different. To be specific, the overall vibration velocity after the peak velocity in Case 5 is higher than that in Case 4, and the duration of vibration also presented a similar law. The results indicated that the in-situ measurement results and numerical results have a high correlation. In order to quantify blasting seismic vibration intensity, the source energy of blasting seismic was calculated as follows (Sanchidrián et al. Citation2007).
(5)
(5)
where r is the propagation distance, ρ is the rock density, c is propagation velocity, v is vibration velocity, t1 and t2 are the start and end times of vibration, respectively.
The results of the source energy of blasting seismic in five cases are shown in . The source energy of blasting seismic increases with the explosive charge and blastholes number. As source energy increases (from 3.1 × 104 J to 1.1 × 106 J), the blasting seismic damage to coal and rock will gradually increase.
5. Discussion
According to the results of numerical simulations and in-situ blasting experiments, as the number of blastholes increases, both the rock blasting damage effect and blasting seismic intensity increase. Moreover, Wojtecki et al. (Citation2022), and Kan, Dou, Li, Li, et al. (Citation2022)pointed out that the blasting seismic source energy can be used to evaluate the destress effect, to be specific, the higher the source energy, the better the destressing effect. However, an excessively strong seismic may cause roadway failure or support structure damage. Therefore, the design of the initiation pattern needs to keep the blasting seismic intensity within the safety threshold. Cai (Citation2022) proposed to determine the critical safety value of seismic source energy by establishing the Gutenberg-Richter frequency-magnitude relationship of seismic events in underground coal mines. Then, a total of 26,705 mining-induced seismic events were collected during the mining of LW1103. The Gutenberg–Richter frequency-magnitude relationship of these seismic events was established, as shown in . The upper limit of source energy is 1 × 106.04 J, which can be considered the critical safety value. Particularly, the seismic source energy in Case 5 can reach 1.1 × 106 J, which is close to the critical safety value. This means that the initiation pattern in Case 5 has a high probability to cause the failure of coal and rock, and even inducing coal bursts. Therefore, a good compromise between blasting damage and seismic effect should be made. Although the initiation pattern in Case 5 may achieve a better damage effect, it is not recommended to be selected due to the excessive blasting seismic effect. Based on numerical results and in-situ measurement results, and considering the blasting damage and seismic effect control, two initiation patterns can be considered better choices. The first is that the three blastholes (35 m, 39 m and 56 m in length) can be initiated simultaneously, and then the blasthole with 98 m length was initiated individually. The second is that the two blastholes (35 m and 39 m in length) can be initiated simultaneously, and then the remaining blastholes (56 m and 98 m in length) were initiated simultaneously.
6. Conclusion
In this research, the effects of initiation pattern on the rock damage and blasting seismic in multi-hole blasting were studied using numerical simulations and in-situ blasting experiments. The following conclusions were drawn.
The determined parameters of the RHT model can well describe the rock damage behaviour subjected to blast loading. The initiation pattern greatly influences the rock damage mode in multi-hole blasting. Due to the superposition of blasting stress waves, the best rock damage effect can be achieved when all the blastholes were initiated simultaneously. Moreover, when the sequential initiation pattern was adopted, the initiation order also significantly changes the rock damage mode. A better rock damage result may be obtained when the blasthole with a large explosive charge was preferentially initiated.
The PPV of rock vibration between the blastholes was significantly improved due to the superposition of blasting vibration when the blastholes were initiated simultaneously. However, the PPV of rock vibration at one side of blastholes does not change with the increase of the number of blastholes, which is more dependent on the vibration induced by the nearest blastholes. At the moment, the superposition of blasting vibration occurred in a certain segment after the peak under the simultaneous initiation pattern, and it will lead to an increase in blasting seismic energy.
The in-situ measurement results indicated that except for the intensity of each independent blasting source, the superposition effect was also influenced by the distance between the independent blasting sources. Only when the blasthole spacing is small, the PPV of rock vibration will increase when the blastholes are initiated simultaneously. However, the source energy of blasting seismic increases with the explosive charge and blastholes number. The main reason is that the source energy of blasting seismic was calculated based on full vibration intensity in the time domain, rather than just PPV. Based on the numerical and in-situ measurement results, reasonable designs of the initiation pattern of deep-hole blasting in the coal mine were given.
Due to the limitation of numerical calculation capability, the numerical model in this study was simplified, such as reducing the model size and simplifying the blasthole shape, which could not be fully matched with the engineering scale. Moreover, the in-situ blasting experiments in this study were carried out under a specific coal mine condition and can only provide a reference for the coal mines with similar conditions. Therefore, in future studies, with the improvement of numerical calculation capability, the numerical model should be improved to better match the engineering scale. Also, more in-situ blasting data should be collected and analysed, and develop methods, such as machine learning for intelligent design of initiation patterns under different mine conditions.
Acknowledgements
We gratefully acknowledge the financial support for this work provided by National Key R&D Program of China (Grant No. 2022YFC3004603), National Natural Science Foundation of China (Grant No. 52227901, 51934007), the Open Research Fund of The State Key Laboratory of Coal Resources and safe Mining, CUMT (SKLCRSM22KF008) and the Postgraduate Research and Practice Innovation Program of Jiangsu Province (Grant No. KYCX21_2378).
Disclosure statement
The authors report no conflict of interest.
Data availability statement
The data presented in this study are available on request from the corresponding author.
Additional information
Funding
References
- Banadaki MMD, Mohanty B. 2012. Numerical simulation of stress wave induced fractures in rock. Int J Impact Eng. 40–41:16–25.
- Bi CC. 2018. Calibration of HJC constitutive parameters of Huashan granite and its blasting damage numerical simulation. Hefei, China: Hefei University of Technology.
- Borrvall T, Riedel W. 2011. The RHT concrete model in LS-DYNA. Proceedings of the 8th European LS-DYNA Users Conference; May; Strasbourg, France.
- Cai W. 2022. Laboratory-scale insight into fractal dimension indicators for seismic hazard assessment associated with rock failures. Shock Vib. 2022:1–8.
- Cai W, Dou L, Zhang M, Cao W, Shi JQ, Feng L. 2018. A fuzzy comprehensive evaluation methodology for rock burst forecasting using microseismic monitoring. Tunnell Underground Space Technol. 80:232–245.
- Chen S, Wu J, Zhang Z. 2015. Influence of millisecond time, charge length and detonation velocity on blasting vibration. J Cent South Univ. 22(12):4787–4796.
- Dou LM, Kan JL, Li XW, Qi YJ, Bai JZ, Liu MH. 2020. Study on prevention technology of rock burst by break-tip blasting and its effect estimation. Coal Sci Technol. 48(1):4–32.
- Dou LM, Tian XY, Cao AY, et al. 2022. Present situation and problems of coal mine rock burst prevention and control in China. J Chin Coal Soc. 47(1):152–171.
- Gao Q, Lu W, Yan P, Hu H, Yang Z, Chen M., 2019. Effect of initiation location on distribution and utilization of explosion energy during rock blasting. Bull Eng Geol Environ. 78(5):3433–3447.
- Hu YG, Lu WB, Chen M, Yan P, Yang, J, H. 2014. Comparison of blast-induced damage between presplit and smooth blasting of high rock slope. Rock Mech Rock Eng. 47(4):1307–1320.
- Huo X, Shi X, Qiu X, Chen H, Zhou J, Zhang S, Rao D. 2021. Study on rock damage mechanism for lateral blasting under high in situ stresses. Appl. Sci. 11(11):4992.
- Johnson GR, Holmquist TJ. 1994. An improved computational constitutive model for brittle materials. AIP Conf Proc. 309:981–984.
- Kan JL, Dou LM, Li JZ, Li XW, Bai JZ, Wang M. 2022. Characteristics of microseismic waveforms induced by underground destress blasting: comparison with those induced by ground blasting and coal ining. Front Earth Sci. 10:797358.
- Kan J, Dou L, Li X, Cao J, Bai J, Chai Y. 2022. Study on influencing factors and prediction of peak particle velocity induced by roof pre-split blasting in underground. Underground Space. 7(6):1068–1085.
- Konicek P, Soucek K, Stas L, Singh R., 2013. Long-hole destress blasting for rockburst control during deep underground coal mining. Int J Rock Mech Min Sci. 61:141–153.
- Konicek P, Waclawik P. 2018. Stress changes and seismicity monitoring of hard coal longwall mining in high rockburst risk areas. Tunnelling Underground Space Technol. 81:237–251.
- Leng ZD, Lu WB, Chen M, et al. 2016. Explosion energy transmission under side initiation and its effect on rock fragmentation. Int J Rock Mech Min Sci. 86:245–254.
- Li T, Chen M, Ye ZW, Lu WB, Wer D. 2021. Study on the energy transfer efficiency of explosive blasting with different coupling medium. Explos Shock Waves. 41(6):062201.
- Lurka A, Mutke G, Małkowski P. 2021. Dynamic influence of near-source seismic ground motion on underground mine working stability using time-frequency analysis. Shock Vib. 2021:1–16.
- Ma GW, An XM. 2008. Numerical simulation of blasting-induced rock fractures. Int J Rock Mech Min Sci. 45(6):966–975.
- Ning Y, Yang J, An X, Ma G., 2011. Modelling rock fracturing and blast-induced rock mass failure via advanced discretisation within the discontinuous deformation analysis framework. Comput Geotech. 38(1):40–49.
- Pan C, Xie LX, Li X, Liu K, Gao PF, Tian LG. 2022. Numerical investigation of effect of eccentric decoupled charge structure on blasting-induced rock damage. J Cent South Univ. 29(2):663–679.
- Qi Q, Li H, Ji Z, Liu J, Pan J. 2007. Theory and application of prevention of rock burst by break-tip blast in deep hole. Chin J Rock Mech Eng. 26:3524–3527.
- Riedel W, Thoma K, Hiermaier S, Schmolinske E. 1999. Penetration of reinforced concrete by BETA-B-500 Numerical analysis using a new macroscopic concrete model for hydrocodes. Proceedings of the 9th international symposium on interaction of the effects of munitions with structures; Berlin, Germany.
- Sanchidrián JA, Segarra P, López LM. 2007. Energy components in rock blasting, International. J Rock Mech Min Sci. 44(1):130–147.
- Sun CH, Qu YD, Kong XQ, Zhang LJ. 2017. Numerical simulation of explosion effects of double-hole blasting in rock medium. Blasting. 34:37–46.
- Tang HL, Liu X, Yang J, Yu Q. 2022. Experimental study on the influence of delay time on rock fragmentation in bench blasting. Appl Sci. 13(1):85.
- Tao J, Yang XG, Li HT, Zhou JW, Fan G, Lu GD. 2020. Effects of in-situ stresses on dynamic rock responses under blast loading. Mech Mater. 145:103374.
- Tawadrous A. 2010. Hard rocks under high strain-rate loading. Ontario, Canada: Queen’s University.
- Wang H, Wang Z, Wang J, Wang S, Wang H, Yin Y, Li F., 2021. Effect of confining pressure on damage accumulation of rock under repeated blast loading. Int J Impact Eng. 156:103961.
- Wang IT. 2015. Numerical simulation and experimental validation of the Mach reflection effect of shock wave under ground surface blast. J Vibroeng. 17(3):1413–1423.
- Wojtecki Ł, Konicek P, Mendecki MJ, Zuberek WM. 2022. Evaluation of destress blasting effectiveness using the seismic moment tensor inversion and seismic effect methods. Int J Geomech. 22(4):04022010.
- Xie LX, Lu WB, Zhang QB, Jiang QH, Chen M, Zhao J. 2017. Analysis of damage mechanisms and optimization of cut blasting design under high in-situ stresses. Tunnel Underground Space Technol. 66:19–33.
- Xie LX, Lu WB, Zhang QB, Jiang QH, Wang GH, Zhao J. 2016. Damage evolution mechanisms of rock in deep tunnels induced by cut blasting. Tunnel Underground Space Technol. 58:257–270.
- Yi C, Johansson D, Greberg J. 2018. Effects of in-situ stresses on the fracturing of rock by blasting. Comput Geotech. 104:321–330.
- Yilmaz O, Unlu T. 2013. Three dimensional numerical rock damage analysis under blasting load. Tunn Undergr Space Technol. 38:266–278.
- Yue HZ, Yu C, Li HB, Zhou CB, Chen SH, Shao ZS. 2022. The effect of blast-hole arrangement, delay time, and decoupling charge on rock damage and vibration attenuation in multihole blasting. Shock Vib. 2022:1–18.,
- Zhang T, Huang J, Li X, Liu T, Bian X, Luo Y., 2023. Characteristics of the elevation amplification effect of vibration velocity in rock surrounding underground cavities under different stress conditions. Soil Dyn Earthquake Eng. 165:107704.
- Zhang X, Yan P, He H, Lu W, Liu B, Zhu J, Wang X, Cheng Y., 2022. Experimental investigations of the effect of millisecond-delay time on the blast vibration reduction with electronic detonators. J Vib Control. 107754632211136.
- Zhang YP. 2006. HHT analysis of blasting vibration and its application. Changsha, China: Central South University.
- Zhao S. 2021. Mechanism of force-structure cooperative prevention and control of deep hole roof pre-blasting for rockburst. J Chin Coal Soc. 46:3419–3432.
- Zhu Z, Mohanty B, Xie H. 2007. Numerical investigation of blasting-induced crack initiation and propagation in rocks. Int J Rock Mech Min Sci. 44(3):412–424.
- Zuo J, Li Z, Zhao S, Jiang Y, Liu H, Yao M. 2019. A study of fractal deep-hole blasting and its induced stress behavior of hard roof strata in bayangaole coal mine, China. Adv Civ Eng. 2019:1–14.