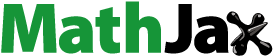
Abstract
The Wenchuan earthquake triggered many landslides, which caused many casualties and led to considerable property losses. Due to the compositionality of the hypocenter mechanism of the Wenchuan earthquake, research on the effects of a composite hypocenter on the dynamic responses of landslides is urgently necessary. Using geological field investigations, a block discrete element method simulation, and related theoretical analysis, the dynamic formation mechanism and main controlling mechanical factors of the Tangjiashan landslide were revealed. The results demonstrated the following. Firstly, the dynamic responses of the slope involved progressive deformation, critical failure, disintegration, fragmentation, collision, climb, return, and accumulation. Secondly, when the earthquake lasted for approximately 23 s, the critical failure occurred, and the sliding mass near the shoulder reached a vertical acceleration of approximately 2.8 g at this time. Thirdly, the second and third subhypocenters contributed to the progressive deformation and critical failure, and vertical seismic forces were dominant contributions to these responses. Fourthly, the disintegration, fragmentation, collision, climb, return, and accumulation were mainly controlled by the self-gravity and local topography and were slightly influenced by subhypocenters. Based on these conclusions, the study of the dynamic formation mechanism of this type of landslide has increased the level of understanding.
1. Introduction
The Longmenshan tectonic belt at the boundary between the Qinghai-Tibet Plateau and the Sichuan Basin in China is strongly active. In 2008, the Magnitude 8 Wenchuan earthquake in Sichuan Province was triggered by the tectonic belt, and its powerful destructive force attracted worldwide attention. The Wenchuan earthquake generated 30,000–50,000 landslides, rock falls and debris flows, claiming ∼20,000 lives (Yin Citation2008; Huang Citation2009; Yin et al. Citation2011). After the earthquake, over 10,000 potential geological hazards were identified, with the most significant increase in rock falls, threatening nearly one million lives (Yin Citation2008; Yin et al. Citation2011). A strong earthquake can change the landscapes of mountains and rivers and trigger significantly destructive secondary hazards (such as landslides, rock falls, and debris flows). Therefore, extensive research was carried out on distribution patterns, deformation and failure characteristics, formation mechanisms, main controlling factors, and dynamic processes of earthquake-induced slope avalanches and slides (Chigira et al. Citation2010; Dai, Tu et al. Citation2011; Dai, Xu et al. Citation2011; Huang et al. Citation2012; Luo Citation2012; Xu et al. Citation2013; Chang et al. Citation2017; Domènech et al. Citation2019; Fan et al. Citation2019; Tang et al. Citation2019). The duration of the Wenchuan earthquake was approximately 120 s (Meng and Shi Citation2011), and the strong ground motions triggered by coseismic fractures resulted in cumulative damage to a large number of slopes until failure. As a result, the dynamic responses and characteristics of slope avalanches and slides have received increasing attention. Many scholars have made advances in this field. Xu et al. (Citation2008) investigated the dynamic response laws of a slope under earthquake action and the influence of ground shaking parameters on the dynamic responses. They found that slopes exhibited vertical and surface amplification when they were subjected to the input seismic waves. Huang (Citation2009) summarized the dynamic response processes and characteristics of slope avalanches and slides in typical examples, and divided the formation mechanism of slope instability into shattering-cracking, shattering-sliding, shattering-falls, peeling, and ejection. Xu et al. (Citation2010) revealed the seismic response laws and the deformation and failure mechanisms of complex slopes under the effects of strong earthquakes. They disclosed that the responses of acceleration behaved obvious non-linearity in both vertical and horizontal directions. Zhou et al. (Citation2013) simulated the dynamic response of the Donghekou landslide and the related movement and accumulation processes. They revealed that the failure was caused by elevation-orientated amplification effect and the sliding mass triggered with a small initial velocity. Rizzitano et al. (Citation2014) analyzed the seismic responses of slopes under the actions of vertically propagating in-plane shear waves, and pointed out that topographic amplification was affected by the non-linear behavior of the soil. Gischig et al. (Citation2016) used discrete element method to simulate the deformation and failure processes of a rock mass caused by a series of repeated earthquakes. Their findings indicated that pre-existing damage had a significant impact on the damage and displacement caused by a certain earthquake. Luo et al. (Citation2021) studied and verified the unique failure process of landslide #1 induced by the Wenchuan earthquake in the Liujiawan valley, Qingchuan County, and classified it into the long-term unloading relaxation failure process of the original slope, the strong seismic dynamic failure process of the original slope, and the progressive failure process after the earthquake. Based on the discrete element method, Gao et al. (Citation2021) investigated the deformation evolution, disintegration process, and fragmentated deposition of the Wangjiayan landslide, and came to the result that under seismic loading, internal rock damage initiates, propagates, and coalesces progressively along the weak solid structure, causing the fragmentation and pulverization of the slope mass. The aforementioned results provide a practical basis for the subsequent thorough investigation conducted in this paper.
When a strong earthquake occurs, seismic waves originating from the hypocenter have a highly destructive effect on the adjacent surfaces, and slope failure is frequently triggered in mountainous areas. The hypocenter of the Wenchuan earthquake has been studied systematically by some scholars. Wang et al. (Citation2008) and Yagi et al. (Citation2012) used a more realistic double-listric finite-fault model and a simple fault model for investigating the rupture process of the Wenchuan earthquake, respectively, indicating the complexity of its rupture. The fracture, formed due to the Wenchuan earthquake, was 300 km long and distributed in a belt pattern (Li et al. Citation2008). From the start to the end of the seismogenic fault (SW to NE direction), the fracture, with the composite property (Meng and Shi Citation2011), was divided into the Guangxian (now Dujiangyan)-Jiangyou fault, Beichuan-Yingxiu fault, and Wenchuan-Maoxian fault, and its faulting mechanism gradually changed from a thrust fault to a strike-slip fault (Li et al. Citation2008; Zhang et al. Citation2009; Fielding et al. Citation2013; Cui et al. Citation2018). We refer to a hypocenter with such characteristics as a composite hypocenter. To synthesize the strong ground motion generated during the complex rupture of large earthquakes, Zeng et al. (Citation1994) proposed a composite-source model and applied it to two subduction zone earthquakes recorded on the Guerrero, Mexico, accelerograph network. Zeng and Anderson (Citation1996) studied the source process of the Northridge earthquake using a composite-source model approach. Liu et al. (Citation2007) built a composite-source model to simulate the strong ground motion of the 1679 Sanhe-Pinggu Magnitude 8 earthquake. Meng and Shi (Citation2011) established a dynamic composite-source model to simulate the strong ground motion of the Wenchuan earthquake. However, except for Cui et al. (Citation2021), no one has investigated the influence of different faulting mechanisms of a composite hypocenter on slope collapses and slides. For slopes adjacent to the Wenchuan earthquake fracture, it is more practical to study the effects of different faulting mechanisms of a composite hypocenter on their avalanches and slides. In general, relevant studies are scarce, and conducting this study is very necessary and urgent. The Tangjiashan landslide triggered by the Wenchuan earthquake was a bedding, high-speed and short-distance rock landslide with a medium steep dip, directly leading to approximately 100 deaths (Hu et al. Citation2009a, Citation2009b). The landslide received more attention due to the formation of a large-scale and hazardous barrier dam. Cui et al. (Citation2010), Cao et al. (Citation2011), Luo et al. (Citation2012), Xu et al. (Citation2013), and Zhao and Crosta (Citation2018) simulated the evolution of the Tangjiashan landslide, but they did not take the influence of the composite property of the hypocenter on its formation into account. Therefore, it is of theoretical significance to investigate the influence of different faulting mechanisms of the composite hypocenter on the dynamic responses of the Tangjiashan landslide.
Based on previous research (Cui et al. Citation2018), Cui et al. (Citation2021) divided the composite hypocenter into the first, second, third and fourth subhhypocenters and revealed the correspondence between the different faulting mechanisms of the composite hypocenter and the different dynamic responses of the Daguangbao (DGB) landslide induce by the Wenchuan earthquake for the first time: the progressive deformation and critical failure of the landslide were triggered by the second subhypocenter, its long runout stage was slightly influenced by the second, third and fourth subhypocenters, and the fourth subhypocenter affected its accumulation less. Overall, the above results provide technical support and a reference for this paper. Based on geological field investigations, a UDEC block discrete element method (DEM) (Cundall Citation1971; Itasca Citation2014) simulation and related theoretical analysis, the paper took the Tangjiashan landslide as an example and used the composite hypocenter classification of Cui et al. (Citation2021) to reveal the correspondence between the different faulting mechanisms of the composite hypocenter and the different dynamic responses of this landslide and reproduce the process of the progressive deformation and dynamic failure of the landslide, with the expectation of enhancing the study of dynamic formation mechanisms of this type of landslide and increasing the level of understanding.
2. Data and methods
2.1. Data
2.1.1. The geological setting
The Tangjiashan landslide is located in the Tongkou River (also known as Jianjiang) of Beichuan County, Sichuan Province. There is a V-shaped medium-high mountain canyon landform. The landslide, triggered by the Wenchuan earthquake, occurred on the right bank of the valley. Its sliding was obstructed by the opposite bank, and the Jiang River was dammed, forming the largest barrier dam triggered by the Wenchuan earthquake. Furthermore, it is on the hanging wall of the Central Longmenshan fault (Yingxiu-Beichuan fault), ∼2.6 km to the northwest of the fault (). depicts remote sensing images of the landslide before and after the Wenchuan earthquake and an overview of the landslide in August 2020.
Figure 1. The distribution of the different faulting mechanisms of the composite hypocenter (modified from Cui et al. (Citation2021)) (based on Google Earth).

Figure 2. Remote sensing images of the Tangjiashan landslide: (a) before the earthquake (based on Google Earth); (b) after the earthquake (based on LocaSpace viewer). (c) an overview of the Tangjiashan landslide in August 2020 (taken by Cui, F P).

Based on detailed geological field investigations and previous research (Hu et al. Citation2009a; Luo Citation2012), the main sliding direction of the landslide was approximately 345°. The landslide dam had a length of ∼800 m along the main sliding direction, a width of ∼600 m along the river, a height of ∼70 m, and a volume of ∼2.037 × 107 m3. According to the volume, it was designated a giant-sized landslide. The top elevation of the landslide was approximately 1400 m, the difference in the elevations between the front and rear edges was approximately 700 m, and the sliding displacement was approximately 800 m ( and ). The original slope, with an inclination of approximately 40°, was a bedding slope. The Quaternary deposits mainly consist of alluvial and slope residual deposits. The Changjianggou Formation of the Lower Cambrian system serves as the subterrane, comprising grayish-black thin-medium thick bedding silicalite, sandstone, marl, and mudstone, with interbedded soft and hard rock layers. The inclination direction and dip angle of the rocks are 340°–350° and 50°, respectively.
Figure 3. Profile of the Tangjiashan landslide and distribution of monitoring points in the numerical modeling (Hu et al. Citation2009a; Luo et al. Citation2012).

2.1.2. Constitutive models and boundary conditions
The discrete element numerical model built in the UDEC software (Itasca Citation2014), shown in , was created according to the profile of the landslide. The elevations of its top left and top right boundaries are approximately 960 m and 1375 m above sea level, respectively. The elevation and length of its bottom are 500 m a.s.l. and 1500 m, respectively. According to detailed geological field investigations, the barrier dam, from the top to the bottom, consisted of slope residual gravel soil, a fragmented gravel layer originating from the broken original strong weathered rocks, and similar laminated fractured rock originating from the disintegrated original slightly weathered rocks (). Therefore, the sliding mass, from the top to the bottom, was divided into completely weathered, strongly weathered, and slightly weathered layers, and the slightly weathered layer comprised the sliding bed.
The discrete element method is classified into the particulate discrete element and the block discrete element. Circular elements in the particulate DEM model are unable to characterize the rock structure of the Tangjiashan slope. However, the layered rock mass of this slope can be described using the quadrangular element in the block DEM model. Therefore, it is appropriate for revealing the dynamic responses of the Tangjiashan landslide to use the quadrangular element in the block DEM model.
In the numerical model, a rigid constitutive model was adopted as the constitutive model of the sliding mass, that is, the sliding mass was assumed as rigid blocks, because the self-deformation of elements composing the sliding mass was much smaller than their movement distance. Completely weathered rocks were highly fragmented, and the shape of Vronoi polygon grids closely resembled that of such rocks. The rock structure of strongly weathered and slightly weathered rocks was layered, making the use of quadrangular elements more appropriate for their description. Therefore, in the slide mass, Voronoi polygon grids were used to simulate the completely weathered layer, and quadrangular grids were used to simulate the strongly weathered and slightly weathered layers (the yellow grids in ). The sliding bed in the model underwent elastic-plastic deformation without obvious fracture and movement under the action of an earthquake (Cui et al. Citation2010, Citation2018). Therefore, an elastic-plastic constitutive model was applied to the sliding bed, and the Mohr–Coulomb yield criterion was employed. Triangular elements were used to simulate the sliding bed (the blue grids in ). In the static analysis, the input boundary conditions of the left and right sides of the model were x-direction fixed and y-direction freed, and the boundary condition of the bottom was y-direction fixed and x-direction freed. In the dynamic analysis, the input boundary conditions of the model were viscous (inner) and free-field (outer) along the left and right sides and viscous along the bottom in order to keep seismic waves propagating outward from being reflecting back into the model and to allow the necessary energy dispersion. First, we calculated the model to reach static equilibrium. Second, monitoring points were set to record the output values, including displacement, velocity, and acceleration. The paper focused on monitoring points 1–6 near the sliding surface, in which monitoring points 1, 3, and 5 were located in the slide mass, and the rest was located in the sliding bed (). The dynamic loads were input into the bottom of the model to simulate the dynamic responses of the landslide for 80 s. Finally, the landslide accumulated due to self-gravity.
2.1.3. Recommended parameters
For the convenience of the subsequent numerical calculation, the lithology of the sliding mass and sliding bed was generalized to siltstone. The sliding mass consisted of completely weathered, strongly weathered, and slightly weathered layers, and the slightly weathered layer comprised the sliding bed. The structural plane was divided into joint planes and rock planes. Based on previous research (Hu et al. Citation2009a; Cui et al. Citation2010; Cao et al. Citation2011; Luo Citation2012; Luo et al. Citation2012), the physical and mechanical parameters of the rock masses and structural planes were obtained ( and ).
Table 1. Physical and mechanical parameters of the rock masses.
Table 2. Mechanical parameters of the structural planes in the slope.
2.1.4. Dynamic conditions
The Qingping seismic station in Mianzhu County is the closest to the Tangjiashan landslide, at approximately 44 km away. Therefore, the acceleration records in the NS, EW, and vertical directions, as monitored by the station, were employed as the basis of the dynamic input. The discrete element model was built based on the profile along the main sliding direction of the landslide. Hence, the horizontal acceleration records in the N-S and E-W directions should be projected to the main sliding direction (NW15°). The acceleration in the NW15° direction was calculated by EquationEquation (1)(1)
(1) .
(1)
(1)
where ACCNW15° is the calculated value of the acceleration along the NW15° direction, ACCNS is the monitored value of the acceleration along the NS direction, and ACCEW is the monitored value of the acceleration along the EW direction. According to the formula, the time–history curve of ACCNW15° was obtained. By adjusting the values of acceleration input during trial numerical calculations, it was observed that when the slope did not slide, the values of acceleration input on the bottom of the model, after being amplified on the surface, were almost equal to the in situ monitored values of the acceleration on the ground. Based on this, the desired values of acceleration input were obtained for the subsequent numerical simulation. Therefore, the values of in situ monitored accelerations were modified, that is, the horizontal and vertical peak ground accelerations were modified to 2.804 m/s2 and 2.022 m/s2, respectively (). The duration of the in situ monitored ground motion accelerations was 160 s. The acceleration data of the front 20 s in the records of 160 s basically originated from ambient vibrations, and their values were relatively small after baseline correction and a low-pass filter. In this paper, the acceleration data of 20–100 s in the records of 160 s (after baseline correction and a low-pass filter), with the phenomenon of obvious ground motion, was used as the earthquake dynamic conditions in the subsequent numerical simulation.
2.1.5. Composite hypocenter classification
According to the main direction of the seismogenic fault evolution, Cui et al. (Citation2021) divided the composite hypocenter into four subhypocenters, i.e. the first, second, third and fourth subhypocenters, triggered by the seismogenic reverse fault, the first seismogenic oblique-slip fault (the reverse component of the fault is much larger than its strike-slip component), the second seismogenic oblique-slip fault (the reverse component of the fault is nearly equal to its strike-slip component) and the seismogenic strike-slip fault, respectively. The above faults are also known as the first, second, third and fourth subfaults. The segments are marked A, B, C and D in . Segment A is from southwestern Yingxiu town to Wenchuan County with a length of ∼40 km, with 7% of the total seismic moment after it fractured, segment B is from Wenchuan County to Maoxian County with a length of ∼90 km, with 61% of the total seismic moment after it fractured, segment C is from Beichuan County to Pingwu County with a length of ∼70 km, with 9% of the total seismic moment after it fractured, and segment D is from Pingwu County to Qingchuan County with a length of ∼110 km, with 23% of the total seismic moment after it fractured (Cui et al. Citation2021). The above hypocenter classification was used to investigate the correspondence of the different faulting mechanism of the composite hypocenter and the dynamic responses of the Tangjiashan landslide.
2.2. Methods
Firstly, a desk study and geological field investigations were conducted to explore the geological conditions of the study area. Secondly, the UDEC software based on a block DEM method was employed to reproduce the process of the progressive deformation and dynamic failure of the landslide and obtain the time of critical failure of the landslide. Thirdly, to validate the model, the critical failure time, duration, and dynamic response process of the Tangjiashan landslide were compared with the results obtained from field interviews and previous research to verify whether they were comparatively consistent. If the comparison showed comparatively consistency, the model’s calculation was considered to be valid. Finally, based on the above comprehensive results, the correspondence between the different faulting mechanisms of the composite hypocenter and the different dynamic responses of this landslide was revealed.
3. Results and analysis
3.1. Results of the numerical modeling
3.1.1. Acceleration and velocity amplification effect
Monitoring points 6 and 7 on the slope were on the same vertical section (). The former lay near the slope surface, and the latter lay in the model bottom. The time–history curves of their accelerations and velocities were obtained in this numerical simulation. illustrates the different amplification effects of their accelerations and velocities. Based on comparison with the input time–history curves of accelerations and velocities, shows the acceleration and velocity amplification factors of the section where monitoring points 6 and 7 were located. The term h/H represents the ratio of the vertical distance h from the micro-element in the slope body to the bottom of the slope body, to the overall height H of the slope body. The amplification factors are the ratio of absolute values of the peak accelerations or velocities from the monitored values of all monitoring points in the section where monitoring points 6 and 7 are located to the absolute value of the peak acceleration or velocity from the monitored point 7 in the model bottom.
Figure 6. Acceleration and velocity time–history curves of monitoring points 6 and 7: (a) horizontal acceleration; (b) vertical acceleration; (c) horizontal velocity; (d) vertical velocity.

Figure 7. Amplification factors of ground motion parameters of the section where monitoring points 6 and 7 were located.

The peak horizontal acceleration near the slope surface (h/H ≈ 1) occurred when the ground motion lasted for ∼34.32 s (point a in ), its absolute value reached approximately 8.58 g, and its amplification factor was ∼1.83 (). The peak vertical acceleration near the slope surface occurred when the ground motion lasted for ∼29.21 s (point b in ), its absolute value reached approximately 8.20 g, and its amplification factor was ∼2.42 (). As shown at point c in , it was observed that the peak horizontal velocity near the slope surface occurred when the ground motion lasted for ∼29.23 s, its absolute value was approximately 0.97 m/s, and its amplification factor was ∼1.20 (). When the ground motion lasted for ∼29.23 s (point d in ), the peak vertical velocity near the slope surface occurred, its absolute value was approximately 0.88 m/s, and its amplification factor was ∼1.14 (). shows that the amplification effect of the vertical acceleration of the section where monitoring points 6 and 7 were located was the most obvious, indicating that the vertical acceleration produced by the earthquake contributed more to the slope failure.
3.1.2. The whole process of slope failure
According to the vertical displacements of monitoring points 1–6 near the sliding surface (), the bifurcation of the curves of the displacements were observed at points A, B, and C, indicating the separation of the sliding mass and sliding bed. That is, the critical failure occurred, and the original slope, subjected to the earthquake lasting for approximately 23 s, began to slide as a whole. From the curves, point A showed the earliest separation of the sliding mass and sliding bed at the slope toe (the front edge of the potential sliding surface), then point B showed their separation at the middle of the potential sliding surface, and finally point C showed their separation at the slope shoulder (the rear edge of the potential sliding surface), demonstrating that the sliding surface gradually formed from the toe to the middle and rear edge. In other words, the slope started to damage at the toe and gradually pulled the failure of the middle and rear edge of the slope, resulting in the overall failure. Therefore, the landslide was a retrogressive landslide.
Figure 8. Vertical displacement time–history curves of monitoring points 1–6 (the slide body and slide bed) near the sliding surface.

As shown in , the slope was subjected to the stage of progressive deformation when ground shaking affected the model from 0 to 23 s. The sliding of the slope toe occurred, but the slide mass did not slide as a whole because of the existence of a locking section at the trailing edge of the potential sliding surface. As shown in , between 23 and 48 s in the modeling, the slide mass moved as a whole and slid down at high speed, along with its disintegration, fragmentation and collision and blocks thrown up because of the vibration. At the time of ground motion lasting for approximately 25 s, the slide mass collided with the opposite riverbank and climbed up (point D in ). shows that between 48 and 64 s in the modeling, the slide mass ceased climbing up (point E in ), its rear continued to disintegrate further, and rocks were thrown up. As shown in , between 64 and 80 s in the modeling, the sliding mass gradually returned and stabilized, and it stopped moving as a whole (point F in ). Some of the blocks were still tossed. Eventually, fragmented rock masses blocked the Tongkou River, forming the landslide dam. shows that between 80 and 100 s in the modeling, the sliding mass exhibited self-stabilizing accumulation behaviour under no earthquake action, and local blocks stopped moving. The results of the numerical modeling showed that the dynamic responses of the Tangjiashan landslide involved progressive deformation, critical failure, disintegration, fragmentation, collision, climb, return, and accumulation.
3.2. Numerical model verification
The numerical results indicated that the duration of the landslide was approximately 64 s, which was comparatively consistent with the duration of approximately 1 min investigated by Xu et al. (Citation2013). The whole dynamic response process of the Tangjiashan landslide were comparatively consistent with the field investigations of Hu et al. (Citation2009a) and Luo (Citation2012).
According to field interviews, the critical failure of the DGB landslide occurred when the Wenchuan earthquake lasted for ∼40 s (Huang et al. Citation2014). With the epicenter near Beichuan (one of epicenters of the Wenchuan earthquake) (Li et al. Citation2008) () as a center, the surrounding zone of 40 km was the scope of the epicenter (Wang et al. Citation2008). Considering the dynamic propagation of the rupture, the DGB landslide, which was ∼34 km away from the epicenter, was affected by the epicenter first, and the Tangjiashan landslide was affected by the epicenter later. Furthermore, both the DGB and Tangjiashan landslides were located in the rupture zone influenced by the Beichuan epicenter, the time of the critical failure of the Tangjiashan landslide should be comparatively consistent with that of the DGB landslide. In this numerical modeling, the in situ monitored acceleration records of 20–100 s were adopted after the baseline correction was executed, and the front 20 s were ignored. The critical failure would occur at the time of an earthquake duration of 43 s if the 20 s were considered, which was comparatively consistent with the result of field investigations.
Based on the above verification, the numerical model’s calculation was valid.
3.3. Response mechanism
The entire seismic ground motion started with the initial rupture of the composite hypocenter and ended with its terminative rupture. When the initial rupture of the composite hypocenter formed at Yingxiu town, the Tangjiashan slope, which was ∼122 km away from the initial rupture, was not subjected to seismic action (). The velocity of the primary wave was ∼6 km/s calculated by Cui et al. (Citation2021). It took 122/6 = 20 s to propagate northeastward from Yingxiu town to the Tangjiashan slope. The surface rupture of the Wenchuan earthquake propagated northeastward from Yingxiu town at an average speed of ∼3.0 km/s (Zhang et al. Citation2009). The numerical results show that the sliding surface formed; meanwhile, the original slope began to slide as a whole when the slope was subjected to the earthquake lasting for approximately 23 s. At that point, the distance between the ongoing rupture front and the initial rupture was 3 km/s*(20 + 23)=129 km.
Based on the aforementioned composite hypocenter classification, the second and third subhypocenters triggered the progressive deformation and critical failure of the slope, with the former playing a dominant role and the latter playing a secondary role. Based on the aforementioned average speed of the surface rupture, the hypocenter classification of Cui et al. (Citation2021), and , the observations reported in were obtained. shows in detail the correspondence between the different faulting mechanisms of the composite hypocenter and the different dynamic responses of the Tangjiashan landslide. According to and , between 23 and 64 s in the model, the slope was during the stages of disintegration, fragmentation, collision, and climb. At this stage, the elements of the slide mass collided and broke up. Because the sliding mass was separated from the sliding bed, the seismic forces had little influence on it and did not affect all elements of the slide mass. Consequently, in this phase, the third and fourth subhypocenters played a minor role, whereas the self-gravity and local topography played a major role. Moreover, the seismic inertial force promoted the disintegration and fragmentation of the slide mass. Between 64 and 80 s in the modeling, the sliding mass gradually returned and accumulated due to the self-gravity and local topography. The third and fourth subhypocenters slightly affected it.
Table 3. Dynamic formation mechanisms of the Tangjiashan landslide induced by different subhypocenters.
3.4. Main controlling mechanical factors
Monitoring points 5 and 6 located at the rear edge of the slope experienced the entire process of progressive deformation. Therefore, these monitored results of the points served as the basis for analysing the main controlling mechanical factors. In , it can be observed that at the stage of progressive deformation and critical failure of the slope (between 0 and 23 s, where point A represented 23 s), the absolute value of overall vertical displacement was larger than the absolute value of overall horizontal displacement. Furthermore, the separation of vertical displacement curves at point A was earlier than that of horizontal displacement curves at point B. These observations indicated that vertical seismic forces posed a main contribution to this stage. By taking the second-order derivative of the vertical displacement curve of monitoring point 5 in , it was found that the sliding body near the shoulder of the slope underwent a vertical acceleration of approximately 2.8 g when the slope was subjected to the earthquake lasting for approximately 23 s.
Figure 10. Displacement time–history curves of monitoring points 5 and 6 (the slide body and slide bed).

When mechanical factors were considered, the vertical seismic forces were produced by the second and third subhypocenters that triggered the progressive deformation and critical failure of the slope, in which the vertical seismic force produced by the second subhypocenter made a key contribution to this stage. The main controlling mechanical factors corresponding to different responses of the Tangjiashan landslide are shown in .
Table 4. The main-control mechanical factors corresponding to different response stages of the Tangjiashan landslide.
shows that the horizontal and vertical displacements increased significantly in the negative direction between 23 and 64 s (the time at point C was 64 s) in the modeling. Due to a large inclination, a great difference in height between the front and rear edges of the slope, and a large gravitational potential energy, the slide mass moved at a large speed and disintegrated. Because the slide mass was separated from the sliding bed, the coupling horizontal and vertical seismic forces, generated by the third and fourth subhypocenters, had little control on its disintegration, fragmentation, collision, and climb, whereas the self-gravity and local topography had main control on them. Furthermore, the disintegration and fragmentation of the sliding mass were controlled by instantaneous seismic inertia forces. Between 64 and 80 s in the modeling, the return and accumulation of the slide mass were mainly controlled by its self-gravity and local topography, and the coupling horizontal and vertical seismic forces generated by the fourth subhypocenter contributed to them to a minor degree.
4. Discussion
Based on the numerical results, the influence of the different faulting mechanisms of the composite hypocenter of the Wenchuan earthquake on the dynamic responses of the Tangjiashan landslide was explored. According to the subhypocenter classification, the progressive deformation and critical failure of the slope were mainly triggered by the second hypocenter. The seismic forces produced by the second subhypocenter posed a key contribution to the responses with consideration of mechanical factors. In addition to the local topography, the self-gravity played a dominant role in the disintegration, fragmentation, collision, and climb of the slide mass, and the third and fourth hypocenters played a minor role in the responses. The instantaneous inertial seismic forces contributed to the disintegration and fragmentation of the slide mass. The self-gravity and local topography controlled the return and accumulation of the slide mass, and the coupling horizontal and vertical seismic forces generated by the fourth subhypocenter influenced them less.
The peak ground accelerations monitored by the Qingping station located near a valley were ∼8 m/s2. With respect to the amplification of accelerations in the slope with increasing elevation, the acceleration monitored near the slope shoulder reached ∼80 m/s2 in this simulation. These large accelerations have been found in DEM studies (Cui Citation2009). Therefore, it is feasible based on the amplification effect.
The hanging wall of the Yingxiu-Beichuan fault (the reverse component was dominant) was a concentrated area of landslides induced by the Wenchuan earthquake (Chigira et al. Citation2010; Fan et al. Citation2019). Because of the hanging wall effect, the hanging wall had the strongest ground shaking from a reverse faulting earthquake. Therefore, there were most landslides there. Whereas on the strike slip segments, the ground shaking was more concentrated around the narrow fault zone, there were typically less landslides, but they were more concentrated at the fault. The reverse component of the third subfault was nearly equal to its strike-slip component. Furthermore, the reverse component of the second subfault was much larger than its strike-slip component, and played a key role in the initiation of the Tangjiashan landslide. The seismic moment inset in demonstrates that the second seismogenic oblique-slip fault (the third subfault) released less energy of this earthquake, whereas the first seismogenic oblique-slip fault (the second subfault) released most of the energy of this earthquake. Therefore, it is plausible that the second hypocenter played a dominant role and the third hypocenter played a secondary role in inducing the progressive deformation and critical failure of this landslide.
At present, previous studies on the formation of the Tangjiashan landslide have been preliminarily investigated, focusing on the effect of factors including geological conditions and earthquakes on its formation (Cui et al. Citation2010; Cao et al. Citation2011; Luo Citation2012; Luo et al. Citation2012; Zhao and Crosta Citation2018). Concerning previous studies on composite sources, scholars mainly use a composite source model to simulate strong ground motion (Zeng et al. Citation1994; Zeng and Anderson Citation1996; Liu et al. Citation2007; Meng and Shi Citation2011). These scholars used machine learning methods for the prediction, prevention, and mitigation of this type of landslide (Merghadi et al. Citation2020; Dou et al. Citation2023), providing some technical support for the prevention and control of such events. The paper advances the study of the influence of a composite hypocenter on slope avalanches and slides, provides technical support and a reference for further research on this type of landslide, and has theoretical significance for the study of the correspondence between different faulting mechanisms of composite hypocenters of other earthquakes and dynamic responses of landslides. The dynamic responses of the landslide serve as a basis for analysing the failure mechanism of the landslide under the earthquake action. It is vital for revealing dynamic failure mechanisms of landslides to investigate the dynamic responses of landslides. Cui et al. (Citation2021) explored the influence of different faulting mechanisms of the composite hypocenter of the Wenchuan earthquake on the dynamic responses of the DGB landslide. Based on this study, to obtain more comprehensive results on the instability mechanism of a large number of typical landslides induced by composite hypocenters, this paper makes a certain contribution. It is important to note that the influences of a composite hypocenter on the failures of different slopes are various. Studying the triggering mechanisms of coseismic landslides at different distances from the initial rupture of the composite hypocenter is conducive to revealing the evolution of their triggering mechanism. This is the novelty of the work. However, there are still shortcomings, i.e. few case studies. Therefore, more case studies are needed to further refine and improve our theory.
5. Conclusion
Based on a DEM simulation, the correspondence between the different faulting mechanisms of the composite hypocenter and the dynamic response of the Tangjiashan landslide was investigated, and the dynamic responses and main controlling mechanical factors of this landslide were revealed. The main conclusions are as follows.
The acceleration and velocity of the section, where the monitoring points near the slope surface and at the bottom of the model were located, had an amplification effect, among which the amplification effect of vertical acceleration was the most obvious, followed by horizontal acceleration, vertical velocity, and then horizontal velocity. That is, the vertical acceleration generated by the earthquake contributed more to the slope failure.
According to the numerical results, the Tangjiashan slope experienced dynamic responses, including progressive deformation, critical failure, disintegration, fragmentation, collision, climb, return, and accumulation.
The second and third subhypocenters induced the progressive deformation and critical failure of the Tangjiashan landslide, with the former playing a dominant role and the latter playing a secondary role. The disintegration, fragmentation, collision, and climb of the slide mass were dominated by the self-gravity and local topography, and the third and fourth subhypocenters played a minor role in them. The fourth subhypocenter slightly influenced the return and accumulation of the slide mass, but the self-gravity and local topography played a key role.
The vertical seismic forces produced by the second and third subhypocenters promoted the progressive deformation and critical failure of the landslide. In particular, the vertical seismic forces produced by the second subhypocenter made a key contribution. The self-gravity and local topography controlled the disintegration, fragmentation, collision, and climb of the slide mass, and the coupling horizontal and vertical seismic forces generated by the third and fourth subhypocenters controlled them to a small degree. The instantaneous inertial seismic forces contributed to the disintegration and fragmentation of the slide mass. The self-gravity and local topography controlled the return and accumulation of the slide mass, and the coupling horizontal and vertical seismic forces generated by the fourth subhypocenter slightly affected them.
Author contributions
C.X., Y.Y., F.C., W.L. and H.L. performed the investigation; C.X. performed the simulation; C.X. and F.C. wrote the manuscript. All authors reviewed and edited the manuscript. Y.Y. supervised the manuscript.
Data availability statement
The raw point data in were derived from the China Earthquake Networks Center at https://www.cenc.ac.cn/. The data (including the code used for DEM simulation) that support the findings of this study are available from the corresponding authors upon reasonable request.
Disclosure statement
No potential conflict of interest was reported by the authors.
Additional information
Funding
References
- Cao YB, Dai FC, Xu C, Tu XB, Min H, Cui FP. 2011. Discrete element simulation of deformation and movement mechanism for Tangjiashan landslide. Chin J Rock Mech Eng. 30(S1):2878–2887.
- Chang M, Tang C, Van Asch TWJ, Cai F. 2017. Hazard assessment of debris flows in the Wenchuan earthquake-stricken area, South West China. Landslides. 14(5):1783–1792. doi: 10.1007/s10346-017-0824-9.
- Chigira M, Wu XY, Inokuchi T, Wang GH. 2010. Landslides induced by the 2008 Wenchuan earthquake, Sichuan, China. Geomorphology. 118(3–4):225–238. doi: 10.1016/j.geomorph.2010.01.003.
- Cui FP, Hu RL, Yin YP, Xu Q, Zhang M. 2010. Discrete element analysis of collapsing and sliding response of slope triggered by time difference coupling effects of P and S seismic waves——taking Tangjiashan landslide in Beichuan County for example. Chin J Rock Mech Eng. 29(02):319–327.
- Cui FP, Xiong C, Wu Q, Xu C, Li N, Wu NA, Cui L. 2021. Dynamic response of the Daguangbao landslide triggered by the Wenchuan earthquake with a composite hypocenter. Geomatics Nat Hazards Risk. 12(1):2170–2193. doi: 10.1080/19475705.2021.1944916.
- Cui FP, Xu Q, Yin YP, Hu RL, Chen ZJ, Liu W. 2018. Dynamic response of slope based on fracture mechanisms of strip-shape hypocenter. Rock Soil Mech. 39(01):320–330.
- Cui FP. 2009. Study of collapsing and sliding response of slope triggered by single and combined action with time difference of P and S seismic waves. Beijing: Institute of Geology and Geophysics, Chinese Academy of Sciences.
- Cundall PA. 1971. A computer model for simulating progressively large scale moments in blocky rock system. In: Proceedings of the International Symposium on Rock Mechanics.
- Dai FC, Tu XB, Xu C, Gong QM, Yao X. 2011. Rock avalanches triggered by oblique-thrusting during the 12 May 2008 Ms 8.0 Wenchuan earthquake, China. Geomorphology. 132(3–4):300–318. doi: 10.1016/j.geomorph.2011.05.016.
- Dai FC, Xu C, Yao X, Xu L, Tu XB, Gong QM. 2011. Spatial distribution of landslides triggered by the 2008 Ms 8.0 Wenchuan earthquake, China. J Asian Earth Sci. 40(4):883–895. doi: 10.1016/j.jseaes.2010.04.010.
- Domènech G, Fan XM, Scaringi G, van Asch TWJ, Xu Q, Huang RQ, Hales TC. 2019. Modelling the role of material depletion, grain coarsening and revegetation in debris flow occurrences after the 2008 Wenchuan earthquake. Eng Geol. 250:34–44. doi: 10.1016/j.enggeo.2019.01.010.
- Dou J,Xiang ZL,Xu Q,Zheng PL,Wang XK,Su AJ,Liu JQ,Luo WQ. 2023. Application and development trend of machine learning in landslide intelligent disaster prevention and mitigation. Eart Sci. 48(05):1657–1674. doi: 10.3799/dqkx.2022.419.
- Fan XM, Scaringi G, Korup O, West AJ, van Westen CJ, Tanyas H, Hovius N, Hales TC, Jibson RW, Allstadt KE, et al. 2019. Earthquake-induced chains of geologic hazards: patterns, mechanisms, and impacts. Rev Geophys. 57(2):421–503. doi: 10.1029/2018RG000626.
- Fielding EJ, Sladen A, Li Z, Avouac JP, Bürgmann R, Ryder I. 2013. Kinematic fault slip evolution source models of the 2008 M7.9 Wenchuan earthquake in China from SAR interferometry, GPS and teleseismic analysis and implications for Longmen Shan tectonics. Geophys J Int. 194(2):1138–1166. doi: 10.1093/gji/ggt155.
- Gao G, Meguid MA, Chouinard LE, Zhan WW. 2021. Dynamic disintegration processes accompanying transport of an earthquake-induced landslide. Landslides. 18(3):909–933. doi: 10.1007/s10346-020-01508-1.
- Gischig V, Preisig G, Eberhardt E. 2016. Numerical investigation of seismically induced rock mass fatigue as a mechanism contributing to the progressive failure of deep-seated landslides. Rock Mech Rock Eng. 49(6):2457–2478. doi: 10.1007/s00603-015-0821-z.
- Hu XW, Huang RQ, Shi YB, Lu XP, Zhu HY, Wang XR. 2009a. Analysis of blocking river mechanism of Tangjiashan landslide and dam-breaking mode of its barrier dam. Chin J Rock Mech Eng. 28(01):181–189.
- Hu XW, Luo G, Huang RQ, Zheng Y, Lu XP, Wang XR, Chen SY, Shi YB. 2009b. Study of stability of remnant mountain body in back scarp of Tangjiashan landslide after “5.12” Wenchuan earthquake. Chin J Rock Mech Eng. 28(11):2349–2359.
- Huang RQ, Pei XJ, Fan XM, Zhang WF, Li SG, Li BL. 2012. The characteristics and failure mechanism of the largest landslide triggered by the Wenchuan earthquake, May 12, 2008, China. Landslides. 9(1):131–142. doi: 10.1007/s10346-011-0276-6.
- Huang RQ, Zhang WF, Pei XJ. 2014. Engineering geological study on Daguangbao landslide. J Eng Geol. 22(04):557–585.
- Huang RQ. 2009. Mechanism and geomechanical modes of landslide hazards triggered by wenchuan 8.0 earthquake. Chin J Rock Mech Eng. 28(06):1239–1249.
- Itasca CGI. 2014. Universal distinct element code (version 6.0) user’s manual. Minnesota.
- Li HB, Wang ZX, Fu XF, Hou LW, Si JL, Qiu ZL, Li N, Wu FY. 2008. The surface rupture zone distribution of the Wenchuan earthquake (Ms 8.0) happened on May 12th, 2008. Geol China. 35(05):803–813.
- Li ZQ, Yuan YF, Li XL, He P. 2008. Some insights into the macro-epicenter and meizoseismal region of Wenchuan earthquake. Seismol Geol. 30(03):768–777.
- Liu BY, Shi BP, Zhang J. 2007. Strong motion simulation by the composite source modeling: a case study of 1679 M8.0 Sanhe-Pinggu earthquake. Acta Seimol Sin. 20(3):319–331. doi: 10.1007/s11589-007-0319-1.
- Luo G, Hu XW, Gu CZ, Wang Y. 2012. Numerical simulations of kinetic formation mechanism of Tangjiashan landslide. J Rock Mech Geotech Eng. 4(2):149–159. doi: 10.3724/SP.J.1235.2012.00149.
- Luo G. 2012. Analysis of blocking mechanism of Tangjiashan high-speed short-run landslide and dam-braeking mode of Tangjiashan barrier dam. Chengdu: Southwest Jiaotong University.
- Luo YH, Zhang Y, Wang YS, He Y, Zhang YY, Cao H. 2021. A unique failure model for a landslide induced by the Wenchuan earthquake in the Liujiawan area, Qingchuan County, China. Eng Geol. 295:106412. doi: 10.1016/j.enggeo.2021.106412.
- Meng LY, Shi BP. 2011. Near-fault strong ground motion simulation of the May 12, 2008, Mw 7.9 Wenchuan Earthquake by dynamical composite source model. Chin J Geophys. 54(04):1010–1027.
- Merghadi A,Yunus AP,Dou J,Whiteley J,ThaiPham B,Bui DT,Avtar R,Abderrahmane B. 2020. Machine learning methods for landslide susceptibility studies: a comparative overview of algorithm performance. Earth Sci Rev. 207:103225.doi: 10.1016/j.earscirev.2020.103225.
- Rizzitano S, Cascone E, Biondi G. 2014. Coupling of topographic and stratigraphic effects on seismic response of slopes through 2D linear and equivalent linear analyses. Soil Dyn Earthquake Eng. 67:66–84. doi: 10.1016/j.soildyn.2014.09.003.
- Tang R, Fan XM, Scaringi G, Xu Q, van Westen CJ, Ren J, Havenith H-B. 2019. Distinctive controls on the distribution of river-damming and non-damming landslides induced by the 2008 Wenchuan earthquake. Bull Eng Geol Environ. 78(6):4075–4093. doi: 10.1007/s10064-018-1381-8.
- Wang WM, Zhao LF, Li J, Yao ZX. 2008. Rupture process of the M-s 8.0 Wenchuan earthquake of Sichuan, China. Chin J Geophys. 51(5):1403–1410.
- Xu GX, Yao LK, Li ZH, Gao ZN. 2008. Dynamic response of slopes under earthquakes and influence of ground motion parameters. Chin J Geotech Eng. 30(06):918–923.
- Xu Q, Liu HX, Zhou W, Fang XM, Chen JJ. 2010. Large-scale shaking table test study of acceleration dynamic responses characteristics of slopes. Chin J Rock Mech Eng. 29(12):2420–2428.
- Xu WJ, Xu Q, Wang YJ. 2013. The mechanism of high-speed motion and damming of the Tangjiashan landslide. Eng Geol. 157:8–20. doi: 10.1016/j.enggeo.2013.01.020.
- Yagi Y, Nishimura N, Kasahara A. 2012. Source process of the 12 May 2008 Wenchuan, China, earthquake determined by waveform inversion of teleseismic body waves with a data covariance matrix. Earth Planet Sp. 64(7):E13–E16. doi: 10.5047/eps.2012.05.006.
- Yin YP, Zheng WM, Li XC, Sun P, Li B. 2011. Catastrophic landslides associated with the M8.0 Wenchuan earthquake. Bull Eng Geol Environ. 70(1):15–32. doi: 10.1007/s10064-010-0334-7.
- Yin YP. 2008. Researches on the geo-hazards triggered by Wenchuan earthquake, Sichuan. J Eng Geol. 16(04):433–444.
- Zeng YH, Anderson JG, Yu G. 1994. A composite source model for computing realistic synthetic strong ground motions. Geophys Res Lett. 21(8):725–728. doi: 10.1029/94GL00367.
- Zeng YH, Anderson JG. 1996. A composite source modeling of the 1994 Northridge earthquake using genetic algorithm. Bull Seismol Soc Am. 86(1B):S71–S83. doi: 10.1785/BSSA08601B0S71.
- Zhang Y, Xu LS, Chen YT. 2009. Spatio-temporal variation of the source mechanism of the 2008 great Wenchuan earthquake. Chin J Geophys. 52(02):379–389.
- Zhao T, Crosta GB. 2018. On the dynamic fragmentation and lubrication of coseismic landslides. J Geophys Res Solid Earth. 123(11):9914–9932. doi: 10.1029/2018JB016378.
- Zhou JW, Cui P, Yang XG. 2013. Dynamic process analysis for the initiation and movement of the Donghekou landslide-debris flow triggered by the Wenchuan earthquake. J Asian Earth Sci. 76:70–84. doi: 10.1016/j.jseaes.2013.08.007.