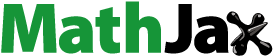
Abstract
Based on the true triaxial dynamic anisotropic permeability model of coal and rock, a multi field coupling mathematical model of structurally anisotropic coal seams under true triaxial stress was established, and a numerical simulation experiment of porous dry gas drainage in anisotropic coal seams was conducted using the COMSOL Multiphysics numerical simulation software. It was found that with an increase in the permeability anisotropy ratio, the greater the ratio of the long and short axes of the elliptical pressure isoline was, the denser was the gas streamline in the dominant seepage direction. As the permeability anisotropy ratio increased, the gas extraction volume and rate decreased. When the permeability was isotropic, the gas drainage volume of the uniformly arranged rectangular boreholes was the largest. However, when the permeability was anisotropic, the gas extraction volume and rate were the largest along the y-direction layout (perpendicular to the dominant seepage direction), followed by the rhombus layout, and the smallest along the x-direction layout. Based on the test results, an optimal arrangement principle of multi holes pumping boreholes along the vertical dominant seepage direction was proposed.
1. Introduction
Gas accidents are critical power disasters in coal mines, and gas drainage is an important measure to prevent gas disasters (Yuan Citation2016; Xie et al. Citation2022). The layout of drainage boreholes has a significant impact on gas drainage. If the spacing between the drainage boreholes is too large or too small, it will crucially affect the coordination between gas drainage efficiency and mining (Lin et al. Citation2019; Fan et al. Citation2021). Therefore, according to the gas permeability characteristics of coal seams, it is important to analyse the effective distance of coal seam gas drainage, and then optimize the layout of the drainage holes to improve the efficiency of gas drainage.
Permeability is an important physical parameter reflecting the seepage characteristics and migration laws of coal seam gas (Chen et al. Citation2012). Coal is a dual-porosity medium, with many matrix pores and fractures, as well as obvious structural anisotropy characteristics. The anisotropy of the internal structure and external stress in coal results in anisotropic seepage (Wang et al. Citation2014; Citation2018; Duan et al. Citation2020). In particular, the anisotropy of the overlying strata seepage in the goaf was more evident (see ). For the anisotropic seepage model, Lou et al. (Citation2021) established a mathematical model that couples the orthotropic permeability and gas flow in coal seams. Li and Li (Citation2021) and Li et al. (Citation2022) proposed an anisotropic coal permeability model incorporating the effects of stress and gas sorption and analysed the evolution mechanism of anisotropic coal under the effects of stress and gas sorption. Zhou et al. (Citation2020) developed a new anisotropic coal permeability model based on the directional fracture strain under the influence of stress, gas sorption and temperature. Various anisotropic permeability models have been established, considering the anisotropy of the internal structure (Gu and Chalaturnyk Citation2010; Pan and Connell Citation2011; Zhang et al. Citation2019), mining-induced stress evolution (Zhang et al. Citation2017), microfracture propagation, and gas sorption desorption effects (Chen et al. Citation2022). Yang et al. (Citation2016) developed an improved anisotropic permeability evolution model for coal, which can replicate the coupling effect between effective stress and matrix shrinkage during coal bed methane (CBM) production. Zeng et al. (Citation2023) established a fully anisotropic coal permeability model incorporating stress sensitivity, anisotropic internal swelling/shrinkage, and gas rarefaction effects. These anisotropic permeability models consider various factors, however, they are static anisotropies, and there has been little research on dynamic anisotropic permeability models.
Figure 2. Schematic illustration of coal cleat system and its geometry. (a) Cleat trace pattern in a plane view; (b) coal cleat system and matrix block; and (c) raw coal.

For coal anisotropic seepage test, Yan et al. (Citation2019) studied the anisotropy of coal permeability and its stress sensitivity, and found that the coal seam permeability is different along the strike, dip, and vertical direction. Lin et al. (Citation2019) investigated the evolution of the permeability of anthracite coal under true triaxial stress conditions and structural anisotropy. Xiao et al. (Citation2021) studied the effective porosity and permeability in three orthogonal directions under different stress conditions and analysed the anisotropic evolution law of the stress sensitivity of permeability. Koenig and Stubbs (Citation1986) conducted field experiments by using CBM production wells and noted that the ratio of the permeability of face cleats to that of butt cleats was as high as 17:1. The anisotropy of permeability is mainly due to the distribution of natural complex pores and fractures, in situ stress and excavation disturbances in the coal seam (Connell et al. Citation2016; Zha et al. Citation2021).
For the optimized layout of coal seam gas drainage boreholes, Lin et al. (Citation2016) studied the relationship between the adsorption constants and permeability, gas pressure, and effective drainage radius of coal seams, as well as applied the approach to the layout of pre-drainage gas drillings in coal seams. Based on the coupling relationship between the seepage field and the deformation equation of coal seams, some scholars have numerically analysed the effective drainage radius of coal seam gas under different geological conditions, investigated the spatio-temporal evolution of gas anisotropy flow fields around boreholes, and discussed the effective area of gas drainage as well as the layout of boreholes (Chen et al. Citation2017; Lin et al. Citation2019; Yan et al. Citation2019; Zhang et al. Citation2019; Xu et al. Citation2022). In the field, the pressure drop and gas flow methods have been used to test the effective drainage radius of coal seam gas (Yu et al. Citation2012; Ji et al. Citation2013). The above studies achieved beneficial results, but all considered the average value or initial anisotropy of coal seams. The anisotropy of coal is a dynamic change, and the dynamic anisotropy of permeability causes differences in the effective radius of gas drainage in different directions. Duan et al. (Citation2020) established a dynamic anisotropic permeability model and performed numerical simulation of single hole gas drainage. However, they did not discuss the optimal layout of multiple holes in anisotropic coal seams.
To reveal the influence of anisotropic permeability on the effective radius of gas drainage and layout of boreholes, a multi-field coupling mathematical model of structural coal seams under true triaxial stress was established based on a dynamic anisotropic permeability model derived from previous research (Duan et al. Citation2020). A numerical simulation experiment of gas drainage in a multi borehole of anisotropic coal seams was conducted using the COMSOL Multiphysics software. The influence of different initial permeability anisotropy ratios and hole layout methods on gas drainage was studied, and an optimal layout method for multiple drainage holes was proposed. This layout method provides scientific guidance for selecting appropriate hole arrangement shapes, spacing, depth, and orientation for the on-site gas drainage holes arrangement process.
2. Multifield coupling model in gas drainage process considering permeability anisotropy
2.1. Basic assumptions of model
To establish a solvable multifield coupling mathematical model based on previous studies, the following basic assumptions were made (Liu et al. Citation2010; An et al. Citation2015; Liu et al. Citation2017; Fan et al. Citation2018).
① Coal is a type of dual porous elastic medium with a single permeability, which is mainly composed of coal skeleton, matrix pores, and fractures, as shown in .
② The elastic deformation of the coal skeleton is small and follows Hooke’s law.
③ Coal seam gas is regarded as an ideal gas, and only the saturated state of a single-phase and single gas component in coal is considered.
④ The flow of gas in the fracture follows Darcy’s law, and the diffusion in the matrix pore follows Fick’s law, as shown in .
⑤ Gas migration and adsorption–desorption are carried out in a constant temperature process, following the Langmuir isothermal adsorption equation.
⑥ The influence of the slippage effect (or Klinkenberg effect) is not considered.
⑦ The matrix permeability of the model is far less than the fracture permeability, therefore, only the fracture permeability is considered and the coal matrix permeability is ignored.
⑧ Fracture permeability anisotropy considers the anisotropy only in the orthogonal direction.
⑨ The gas density is related to the gas pressure, and the inertial force and volume stress of free gas seepage and coal deformation are ignored.
2.2. Governing equation of stress field in gas bearing coal seam
① Stress balance equation
The effects of pore pressure and adsorption stress on the effective stress of gas-bearing coal under a dual-pore system can be obtained as follows:
(1)
(1)
where σeij is the effective stress of gas-bearing coal (MPa), pm,i is the pore pressure in the coal matrix (MPa), pf,i is the pore pressure in the coal fracture (MPa), σab is the adsorption stress of the coal matrix (MPa), a and β are the Biot coefficients of the coal matrix and fracture, respectively, which are expressed as follows (Wu et al. Citation2011):
(2)
(2)
where Km is the bulk modulus of the coal matrix (GPa), K is the bulk modulus of coal, (GPa), and Kf is the bulk modulus of the coal fracture (GPa).
According to the Langmuir adsorption principle, the expansion stress generated by adsorption can be expressed as follows (Liu et al. Citation2010)
(3)
(3)
where εm and pL are the isothermal adsorption deformation constants, εm is the ultimate adsorption strain (%), pL is the corresponding gas pressure when the adsorption strain is half of the limit adsorption strain (MPa), and pm is the gas pressure in the coal matrix (MPa).
In conclusion, the stress balance equation for coal expressed by the effective stress can be obtained as follows:
(4)
(4)
where Fi is the component of volume stress (MPa).
② Constitutive equation of gas-bearing coal
According to the basic assumption ②, the elastic constitutive equation of coal satisfies the generalized Hooke’s law
(5)
(5)
where G is the shear modulus of coal (GPa), λ is the Lame constants (GPa), εij is the strain tensor component of coal. δij is kronecker delta. εkk is volumetric strain.
③ Control equation of stress field in gas-bearing coal
Considering the influence of the pore pressure of the double pore system and the adsorption of coal matrix on the effective stress of coal, the control equation of the stress field of gas-bearing coal can be obtained by combining EquationEquations (4)(4)
(4) and Equation(5)
(5)
(5) (Wu et al. Citation2010):
(6)
(6)
where ui,jj is the displacement component of coal.
2.3. Governing equation of gas migration field in coal matrix
① Control equation of free gas in pores of coal matrix
The pore volume of the coal matrix can be characterized by its porosity, and the gas content of the matrix pores in the unit coal volume can be calculated using the following formula (Lin et al. Citation2019):
(7)
(7)
where mmfree is the mass of free gas in the pores of the coal matrix (kg/m3), φm is the porosity of coal matrix (%), ρgm is the density of free methane in the pores of coal matrix (kg/m3), R is a constant of the ideal state of the gas, M is the molar mass of methane gas molecule (kg/mol), and T is temperature (K). According to the basic assumption ③ that the gas is ideal and single-phase saturated gas, the density of free gas in the pores of coal matrix can be obtained from the ideal gas state equation.
② Control equation of adsorbed gas in coal rock matrix
The adsorbed gas satisfies the Langmuir isothermal adsorption equation, therefore, the mass of the adsorbed gas in the coal matrix can be expressed as follows (Wang et al. Citation2013):
(8)
(8)
where mmab is the mass of adsorbed gas per unit volume of coal matrix (kg/m3), mmsor is the mass of gas desorbed by coal matrix per unit volume, when the adsorption is balanced, the amount of adsorbed gas is equal to the amount of desorbed gas (kg/m3), ρga is the gas density under standard atmospheric pressure (kg/m3), ρc is the density of coal (kg/m3), pL represents the Langmuir pressure constant (MPa), and Vl represents the Langmuir volume constant (m3/kg).
③ Control equation of gas diffusion field between coal matrix and fracture
The gas seepage in the coal matrix was very small and could be ignored relative to the fracture seepage. Therefore, only the diffusion effect was considered for gas migration in the matrix. The gas diffusion in the coal matrix follows Fick’s law (Liu et al. Citation2017):
(9)
(9)
where Qd is the gas exchange flux of coal matrix diffusing to the fracture and the mass source of fracture seepage [kg/(m3·s)], Di is the dynamic diffusion coefficient (m2/s), ς is the shape factor, reflecting the geometric shape of the matrix or fracture (ς = 3π2/A2, m−2), A is the spacing of cracks (m), and ▽C is the diffusion gradient or concentration difference (kg/m3).
Liu et al. (Citation2017) found that the diffusion coefficient of gas in the matrix is not a constant, but a variable that changes with the matrix gas pressure. The fitting function of the dynamic diffusion coefficient is obtained as follows:
(10)
(10)
where Di0 is the initial diffusion coefficient (m2/s), and η is the fitting coefficient (s−1).
④ Control equation of gas migration in coal matrix
According to the principle of mass conservation, the gas change in the matrix is equal to the gas flux diffused to the fracture; thus, the gas mass conservation equation in the matrix can be obtained as follows (Liu et al. Citation2017):
(11)
(11)
where mm is the total gas content in the coal matrix (kg/m3).
The control equations for gas migration in a coal matrix can be obtained using EquationEquations (12)(12)
(12) , Equation(13)
(13)
(13) , and Equation(14)
(14)
(14) simultaneously.
(12)
(12)
2.4. Control equation of gas seepage field in coal fracture
① Control equation of free gas in coal fracture
The expression method of the free gas quality in coal fractures is the same as that of the free gas quality in matrix pores; therefore, the equation of free gas in fractures per unit volume can be used in the same manner:
(13)
(13)
where mf is mass of gas in coal fractures (kg/m3), φf is the fracture rate of coal (%), and ρgf is the density of free gas in coal fractures (kg/m3). The fracture rate of coal φf can be obtained as follows (Duan et al. Citation2020):
(14)
(14)
where φf0 is the initial fracture porosity (%), γi is the modulus reduction ratio defined by Liu et al. (Citation2010), (γi=Ei/Eim), Δεs is the adsorption volume strain increment (%), and Δεi is the strain increment of coal (%).
② Control equation of gas flow in coal fracture
The gas flow in coal fracture follows Darcy’s law, and the gas seepage velocity in the fracture can be expressed as follows:
(15)
(15)
where v is the seepage velocity of the gas in coal fissure (m/s), k is the permeability of coal (m2), and v and k are vector matrices that consider only orthogonal directions:
(16)
(16)
According to a previous study (Duan et al. Citation2020), a gas seepage experiments was conducted with different true triaxial stresses and different seepage directions, and a dynamic anisotropic seepage model based on the experimental results was established and validated.
(17)
(17)
where ξ is fracture width anisotropy.
③ Control equation of gas migration in coal fracture
The gas in the coal fracture is generated through the gas desorption and diffusion in the matrix. According to the law of mass conservation of gas in the fracture, the control equation of gas seepage in the fracture can be obtained as follows (Liu et al. Citation2017):
(18)
(18)
Substituting EquationEquations (12)(12)
(12) and Equation(16)-(17) into Equation (20), the control equation for gas migration in the coal fracture can be obtained by derivation and simplification as follows:
(19)
(19)
2.5. Control equations of multifield coupling mathematical model in coal seam
We assembled governing EquationEquations (6)(6)
(6) , Equation(12)
(12)
(12) , Equation(17)
(17)
(17) and Equation(19)
(19)
(19) to establish the multifield coupling mathematical model of coal considering permeability anisotropy. The coupling relationships are depicted in . The bidirectional interactions among the coal deformation, gas flow in the coal fracture and gas diffusion in the coal matrix illustrate the full coupling of the proposed model. This model has been validated in a previous study (Duan et al. Citation2020). These governing equations are nonlinear second-order partial differential equations (PDEs) in both the space and time domain and are difficult to solve theoretically because of nonlinearity (Li et al. Citation2016; Fan et al. Citation2017). COMSOL Multiphysics provides a powerful PDE-based modelling environment that was used to obtain a numerical solution using the finite element (FE) method (Fan et al. Citation2019). The solid mechanics module was applied to evaluate coal deformation, and two PDE modules were applied to calculate the gas seepage and diffusion fields. The model considers the effective stress, dynamic permeability anisotropy, dynamic diffusion coefficient and other factors, and can more accurately simulate and reproduce the changes in the stress seepage field during coal and gas mining. Next, the established mathematical model was imported into the COMSOL software to perform a numerical simulation of gas drainage based on dynamic permeability anisotropy.
3. Numerical simulation of gas drainage based on dynamic permeability anisotropy
3.1. Model establishment and boundary condition setting
① Establishment of physical model
The relevant physical parameters for the gas drainage numerical simulation are listed in . The geometric physical model of the porous dry gas drainage and different arrangements of the boreholes are shown in . The drilling depth was 50 m, and the drilling hole diameter was 0.5 m.
Table 1. Basic parameters of the numerical simulation (Zhao et al. Citation2018; Duan et al. Citation2020).
② Boundary condition setting
As shown in , the top of the model is subjected to the stress of the overlying strata and is set to a 20 MPa boundary load. The bottom of the model is set as a fixed constraint boundary, implying that the displacement in all directions is zero. The front, rear, left, and right sides of the model are provided with roller supports, implying that only the normal displacement is zero, and displacement can occur in other directions. The six outer faces of the model are gas-impermeable boundary surfaces that are zero-flux surfaces. Therefore, when calculating the seepage or diffusion fields, non-flow boundary conditions were applied to the six outer faces of the model. In addition, a constant pressure of 0.88 MPa was set at the boundary of the inner wall of the bore hole for gas extraction. The gas pressure in the fracture and matrix in the initial state of the coal was set to 3 MPa. Owing to the large number of three-dimensional simulation calculations, the time step set for the simulation calculation in this experiment was large, the time step was 1 day, and the total simulated extraction time was 500 days.
③ Simulation process monitoring settings
To monitor the accumulated gas drainage volume at different time points during the numerical simulation process, domain probe 1 was used to integrate the gas volume over the entire domain. The gas content of each time node in the coal seam can be obtained using the domain probe integral function, and the amount of gas extracted can then be obtained according to the change in gas in the coal seam.
3.2. Design of multi-borehole layout scheme for gas drainage
To explore the influence of permeability anisotropy on the gas extraction range and drilling hole layout, the influence of permeability anisotropy and optimized drilling hole layout on CBM production was evaluated according to the anisotropy ratio of permeability in different directions measured by Wang et al. (Citation2018) (). Twelve gas extraction numerical calculations were performed under different conditions (i.e. three different permeability anisotropy ratios and four different drilling hole layout combinations, as shown in and ). (Note: For effective comparison between different permeability anisotropy ratios, the equivalent permeability of the three different permeability anisotropy ratios is the same, that is, the equivalent permeability is Ke=(kx0×ky0×kz0)/3 = 4.16 × 10−17 m2. The initial permeability value and permeability anisotropy ratios set in the simulation test was shown in ).
Figure 6. Permeability measurements in different directions are obtained by Wang et al. (Citation2018).

Figure 7. The gas pressure of coal seams with different permeability anisotropy ratio and borehole layout after 500 days of gas drainage.

Table 2. Initial directional permeability values of the three simulation cases.
3.3. Analysis of gas drainage in multi-borehole
shows the gas pressure contour map and ABC section pressure distribution map of the coal seams with different permeability anisotropy ratios and borehole arrangements after 500 days of gas drainage. With an increase in the permeability anisotropy ratio, the shape of the gas–pressure isosurface becomes narrower and flatter. When the permeability is isotropic, the gas pressure isosurface exhibits a circular funnel shape centred on the borehole. When the permeability is anisotropic, the gas isosurface presents an elliptical shape with the borehole as the centre. The greater the permeability anisotropy ratio, the greater the ratio of the long and short axes of the elliptical pressure isoline and the denser the gas streamline in the dominant seepage direction. There were significant differences in the contour surface and contour map of the gas drainage under different borehole arrangements. When the permeability was isotropic, the gas boreholes were evenly arranged and the overall gas pressure decreased rapidly. When the permeability was anisotropic, the different arrangements of the drainage holes had a significant impact on the overall decline in gas pressure in the coal seam. The linear arrangement along the dominant seepage direction had the smallest effective gas drainage range, whereas the linear arrangement perpendicular to the dominant seepage direction had the largest effective gas drainage range.
The gas pressure cloud diagram shown in only reflects the spatial pattern of the gas pressure change of the gas drainage species under different permeability anisotropy ratios and borehole arrangements. To more intuitively and accurately reflect the impact of different permeability anisotropy ratios and borehole arrangement formed on gas drainage volume and gas drainage efficiency, show the spatial pattern for gas pressure change of different permeability anisotropy ratios and the variation in gas extraction volume and extraction efficiency with extraction time under different borehole layouts.
① Influence of permeability anisotropy ratio on gas extraction
The gas drainage volume increases with time. The rate of increase gradually slows, and the curve change trend is parabolic. This is because the gas pressure difference is large and the gas migration distance is short in the initial stage. As the extraction time increased, the gas pressure or concentration difference decreased, the gas migration power decreased, the extraction range increased, and the gas flow distance increased. For the same borehole arrangement, the gas drainage volume decreased with increasing permeability anisotropy ratio. This may be because the larger the permeability anisotropy ratio, the greater is the gas seepage velocity along a certain dominant direction and the smaller is the seepage velocity in the inferior direction in the gas drainage process. The distribution of the seepage velocity is uneven, the gas convergence flow is more obvious, and the gas in the inferior direction cannot flow straight to the extraction borehole, but detours in the superior direction. As the seepage distance increased, the total amount of extraction decreased for the same extraction time. However, for boreholes arranged linearly along the Y-direction (along the dominant vertical seepage direction), the gas drainage volume changed little with an increase in the permeability anisotropy ratio. This shows that the decrease in the gas extraction volume caused by permeability anisotropy can be compensated for by optimizing the drilling holes. When the drilled holes were arranged perpendicular to the dominant direction, the permeability anisotropy ratio had less influence on the gas extraction volume. The amount of gas drainage is a result of the comprehensive influence of the gas borehole arrangement and permeability anisotropy.
② Influence of borehole layout on gas extraction
It can be seen from the enlarged drawing in that the borehole forms at the initial stage of extraction (10 days) has little impact on the extraction volume, and the extraction is almost the same. After 10 days of extraction, different drainage borehole forms have a significant impact on the gas drainage volume (see ). When the permeability is isotropic, the gas drainage volume of the rectangular-layout boreholes is the largest, followed by that of the rhombic layout. The layout along the X- and Y-directions is equivalent, and the gas drainage volume is the same and smallest. However, when the permeability of coal is anisotropic, the greater is the permeability anisotropy ratio, the greater is the difference in the gas extraction volume among the different layout modes. The linear layout in the Y-direction (perpendicular to the dominant seepage flow direction) has the largest gas extraction volume, followed by the rhombic layout. The gas extraction volume of the rectangular layout was slightly smaller than that of the rhombic layout, and the linear layout in the X-direction had the smallest gas extraction volume. Through the analysis of gas drainage simulation experiments, the principle of optimising the layout of drainage boreholes was to lay boreholes along the vertical dominant seepage direction as much as possible.
③ Influence of permeability anisotropy ratio and borehole layout on gas extraction rate
It can be seen from and its local enlarged view that with an increase in the extraction time, the gas extraction rate generally increases rapidly, then decreases rapidly, and subsequently decreases slowly. Different permeability anisotropies and borehole layout forms significantly affect the gas extraction rate. Taking a permeability anisotropy ratio of 1:20 as an example, the linear layout in the Y-direction has the largest gas extraction rate, followed by the rhombic layout, and the smallest gas extraction rate was observed in the linear layout in the X-direction. Using a rhombus layout as a column, the gas extraction rate decreased with an increase in the permeability anisotropy ratio.
4. Discussion
shows the comparison of effective extraction areas between isotropic and anisotropic coal bodies. From the comparison of simulation results of isotropic and anisotropic coal bodies (), the effective extraction areas of isotropic and anisotropic coal bodies are circular and elliptical, respectively. The effective extraction area range in the parallel bedding direction is basically the same, while the effective extraction area range in the vertical bedding direction is significantly different. Therefore, as shown in , isotropic coal bodies have effective extraction radii R, however, anisotropic coal bodies have effective extraction radii R and r (r less than R) in parallel and vertical bedding directions, respectively. The original coal seam structure exhibits anisotropic seepage characteristics (Wang et al. Citation2023). If the drilling layout on site is guided by the theory and simulation results of previous isotropic coal seams, there will be significant errors and blind spots for gas extraction (), which will cause high residual gas content in some areas of the coal seam and threaten the safety production of the coal mine underground. Therefore, in order to eliminate this potential risk factor, when arranging boreholes in coal seams, the anisotropic characteristics of the coal seams should be taken into account. The effective extraction radius r in the vertical bedding direction should be used for borehole layout, and the number of boreholes in the vertical bedding direction should be appropriately increased. The borehole layout should be reasonably arranged to reduce the ineffective extraction area.
Figure 11. Schematic Diagrams of zones affected by drainage from boreholes in isotropic and anisotropic coal masses: (a) simulation results of isotropic and anisotropic coal bodies; (b) effective extraction radius of isotropic and anisotropic coal bodies; (c) comparison of effective extraction ranges between isotropic and anisotropic coal bodies; and (d) layout of anisotropic coal body drilling.

5. Conclusions
To reveal the effect of seepage anisotropy on the effective radius of gas drainage and the coupling between seepage anisotropy and borehole arrangement, a multi-field coupling mathematical model of structurally anisotropic coal seams under true triaxial stress was established based on the true triaxial dynamic anisotropic permeability model of coal. A numerical simulation experiment of porous dry gas drainage in anisotropic coal seams was conducted using the COMSOL Multiphysics software. The results are as follows.
With an increase in the permeability anisotropy ratio, the greater the ratio of the long and short axes of the elliptical pressure isoline, the denser the gas streamline in the dominant seepage direction.
The gas drainage volume increased with time in a parabolic manner. However, the gas extraction rate first increased rapidly, then decreased rapidly over time, and subsequently decreased slowly. As the permeability anisotropy ratio increased, the gas extraction volume and rate decreased. However, for boreholes arranged linearly along the Y-direction (along the dominant vertical seepage direction), the gas drainage volume changed little with an increase in the anisotropy ratio.
When the permeability was isotropic, the gas drainage volume of the uniformly arranged rectangular boreholes was the largest. However, when the permeability was anisotropic, the gas extraction volume and rate were the largest along the y-direction layout (along the direction perpendicular to the dominant seepage direction), followed by the rhombus layout, which was the smallest along the x-direction layout.
Based on the test results, an optimal arrangement principle for multi pumping boreholes along the vertical dominant seepage direction was proposed. It provides scientific guidance for the selection of the appropriate hole arrangement form, spacing, depth, and orientation in the process of on-site gas drainage drilling.
Nomenclature | ||
σij | = | the stress of gas bearing coal |
σeij | = | the effective stress of gas bearing coal |
pm,i | = | the pore pressure in the coal matrix |
pf,i | = | the pore pressure in the coal fracture |
σab | = | the adsorption stress of coal matrix |
a and β | = | the Biot coefficients of coal matrix and fracture |
K | = | the bulk modulus of coal |
Km | = | the bulk modulus of coal matrix |
Kf | = | the bulk modulus of coal fracture |
εm and pL | = | isothermal adsorption deformation constant |
Fi | = | the component of volume stress |
G | = | the shear modulus of coal |
λ | = | Lame constants |
εij | = | the strain tensor component of coal |
δij | = | kronecker delta |
εkk | = | volumetric strain |
ui,jj | = | the displacement component of coal |
mmfree | = | the mass of free gas in the pores of coal matrix |
φm | = | the porosity of coal matrix |
ρgm | = | the density of free methane in the pores of coal matrix |
R | = | a constant of the ideal state of the gas |
M | = | the molar mass of methane gas molecule |
T | = | temperature |
mmab | = | the mass of adsorbed gas per unit volume of coal matrix |
mmsor | = | the mass of gas desorbed by coal matrix per unit volume |
ρga | = | the gas density under standard atmospheric pressure |
ρc | = | the density of coal |
pL and Vl | = | the Langmuir pressure constant |
Qd | = | the gas exchange flux of coal matrix diffusing to the fracture |
Di | = | the dynamic diffusion coefficient |
ς | = | a shape factor |
A | = | the spacing of cracks |
▽C | = | diffusion gradient or concentration difference |
Di0 | = | the initial diffusion coefficient |
η | = | the fitting coefficient |
mm | = | the total gas content in the coal matrix |
mf | = | mass of gas in coal fractures |
φf | = | the fracture rate of coal |
ρgf | = | the density of free gas in coal fractures |
φf0 | = | the initial fracture porosity |
γi | = | the modulus reduction ratio |
Δεs | = | the adsorption volume strain increment |
Δεi | = | the strain increment of coal |
v | = | the seepage velocity of the gas in coal fissure |
k | = | the permeability of coal |
ξ | = | fracture width anisotropy |
Data availability statement
The data used to support the findings of the study are available from the corresponding author upon request.
Disclosure statement
No potential conflict of interest was reported by the author(s).
Additional information
Funding
References
- An H, Wei XR, Wang GX, Massarotto P, Wang FY, Rudolph V, Golding SD. 2015. Modeling anisotropic permeability of coal and its effects on CO2 sequestration and enhanced coalbed methane recovery. Int J Coal Geol. 152(B):15–24. doi: 10.1016/j.coal.2015.09.013.
- Chen D, Pan ZP, Liu JS, Connell LD. 2012. Characteristic of anisotropic coal permeability and its impact on optimal design of multi-lateral well for coalbed methane production. J Pet Sci Eng. 88-89:13–28. doi: 10.1016/j.petrol.2012.04.003.
- Chen D, Ye Z, Pan Z, Zhou Y, Zhang J. 2017. A permeability model for the hydraulic fracture filled with proppant packs under combined effect of compaction and embedment. J Petrol Sci Eng. 149:428–435. doi: 10.1016/j.petrol.2016.10.045.
- Chen M, Masum S, Sadasivam S, Thomas H. 2022. Modelling anisotropic adsorption-induced coal swelling and stress-dependent anisotropic permeability. Int J Rock Mech Min Sci. 153:105107. doi: 10.1016/j.ijrmms.2022.105107.
- Connell LD, Mazumder S, Sander R, Camilleri M, Pan Z, Heryanto D. 2016. Laboratory characterisation of coal matrix shrinkage cleat compressibility and the geomechanical properties determining reservoir permeability. Fuel. 165:499–512. doi: 10.1016/j.fuel.2015.10.055.
- Duan MK, Jiang CB, Gan Q, Zhao HB, Yang Y, Li ZK. 2020. Study on permeability anisotropy of bedded coal under true triaxial stress and its application. Transp Porous Med. 131(3):1007–1035. doi: 10.1007/s11242-019-01375-y.
- Fan CJ, Elsworth D, Li S, Zhou LJ, Yang ZH, Song Y. 2019. Thermo-hydro-mechanical-chemical couplings controlling CH4 production and CO2 sequestration in enhanced coalbed methane recovery. Energy. 173:1054–1077. doi: 10.1016/j.energy.2019.02.126.
- Fan CJ, Li S, Luo MK, Du WZ, Yang ZH. 2017. Coal and gas outburst dynamic system. Int J Min Sci Technol. 27(1):49–55. doi: 10.1016/j.ijmst.2016.11.003.
- Fan YP, Deng CB, Zhang X, Li FQ, Wang XY, Qiao L. 2018. Numerical study of CO2-enhanced coalbed methane recovery. Int J Greenh Gas Con. 76:12–23. doi: 10.1016/j.ijggc.2018.06.016.
- Fan ZL, Fan GW, Zhang DS. 2021. Representation of mining permeability and borehole layout optimization for efficient methane drainage. Energy Rep. 7:3911–3921. doi: 10.1016/j.egyr.2021.06.069.
- Gu FG, Chalaturnyk R. 2010. Permeability and porosity models considering anisotropy and discontinuity of coalbeds and application in coupled simulation. J Petrol Sci Eng. 74(3-4):113–131. doi: 10.1016/j.petrol.2010.09.002.
- Ji H, Li ZH, Yang YL, Liu Z, Yang JC. 2013. Drainage radius measurement based on gas flow field. J Min Safety Eng. 30(6):917–921.
- Koenig RA, Stubbs PB. 1986. Interference testing of a coalbed methane reservoir. In: SPE Unconventional Gas Technology Symposium. Society of Petroleum Engineers, Louisville, Kentucky. p. 12. doi: 10.2118/15225-MS.
- Li JH, Li BB. 2021. Modeling of anisotropic coal permeability under the effects of matrix-fracture interaction. J Nat Gas Sci Eng. 93:104022. doi: 10.1016/j.jngse.2021.104022.
- Li JH, Li BB, Cheng QY, Gao Z. 2022. Characterization of anisotropic coal permeability with the effect of sorption-induced deformation and stress. Fuel. 309:122089. doi: 10.1016/j.fuel.2021.122089.
- Li S, Fan C, Han J, Luo M, Yang Z, Bi H. 2016. A fully coupled thermal-hydraulic mechanical model with two-phase flow for coalbed methane extraction. J Nat Gas Sci Eng. 33:324–336. doi: 10.1016/j.jngse.2016.05.032.
- Lin B, Song H, Zhao Y, Liu T, Kong J, Huang Z. 2019. Significance of gas flow in anisotropic coal seams to underground gas drainage. J Petrol Sci Eng. 180:808–819. doi: 10.1016/j.petrol.2019.06.023.
- Lin H, Huang M, Li S, Zhang C, Cheng L. 2016. Numerical simulation of influence of Langmuir adsorption constant on gas drainage radius of drilling in coal seam. Int J Min Sci Technol. 26(3):377–382. doi: 10.1016/j.ijmst.2016.02.002.
- Liu JS, Chen ZW, Elsworth D, Miao XX, Mao XB. 2010. Linking gas-sorption induced changes in coal permeability to directional strains through a modulus reduction ratio. Int J Coal Geol. 83(1):21–30. doi: 10.1016/j.coal.2010.04.006.
- Liu T, Lin BQ, Yang W, Liu T, Kong J, Huang ZB, Wang R, Zhao Y. 2017. Dynamic diffusion-based multifield coupling model for gas drainage. J Nat Gas Sci Eng. 44:233–249. doi: 10.1016/j.jngse.2017.04.026.
- Lou Z, Wang K, Zang J, Zhao W, Qin BB, Kan T. 2021. Effects of permeability anisotropy on coal mine methane drainage performance. J Nat Gas Sci Eng. 86:103733. doi: 10.1016/j.jngse.2020.103733.
- Pan Z, Connell LD. 2011. Modelling of anisotropic coal swelling and its impact on permeability behaviour for primary and enhanced coalbed methane recovery. Int J Coal Geol. 85(3-4):257–267. doi: 10.1016/j.coal.2010.12.003.
- Wang DK, Lv RH, Wei JP, Zhang C, Yu C, Yao BH. 2018. An experimental study of the anisotropic permeability rule of coal containing gas. J Nat Gas Sci Eng. 53:67–73. doi: 10.1016/j.jngse.2018.02.026.
- Wang JG, Liu JS, Kabir A. 2013. Combined effects of directional compaction, non-Darcy flow and anisotropic swelling on coal seam gas extraction. Int J Coal Geol. 109-110:1–14. doi: 10.1016/j.coal.2013.01.009.
- Wang K, Wang G, Zang J, Zhou AT. 2014. Anisotropic permeability evolution of coal with effective stress variation and gas sorption: model development and analysis. Int J Coal Geol. 130:53–65. doi: 10.1016/j.coal.2014.05.006.
- Wang PT, Qi ZW, Ma C, Cai MF. 2023. Anisotropic behavior of the seepage-stress coupling mechanical model of coal pillars of underground reservoirs. Geomech Geophys Geo-Energ Geo-Resour. 9(3):1–20. doi: 10.1007/s40948-023-00549-9.
- Wu Y, Liu JS, Elsworth D, Chen ZW, Connell L, Pan ZJ. 2010. Dual poroelastic response of a coal seam to CO2 injection. Int J Greenh Gas Con. 4(4):668–678. doi: 10.1016/j.ijggc.2010.02.004.
- Wu Y, Liu JS, Elsworth D, Siriwardane H, Miao XX. 2011. Evolution of coal permeability: contribution of heterogeneous swelling processes. Int J Coal Geol. 88(2-3):152–162. doi: 10.1016/j.coal.2011.09.002.
- Xu H, Qin Y, Wu F, Zhang F, Liu W, Liu J, Guo M. 2022. Numerical modeling of gas extraction from coal seam combined with a dual-porosity model finite difference solution and multi-factor analysis. Fuel. 313:122687. doi: 10.1016/j.fuel.2021.122687.
- Xiao K, Zhang ZT, Zhang R, Gao MZ, Xie J, Zhang AL, Liu Y. 2021. Anisotropy of the effective porosity and stress sensitivity of coal permeability considering natural fractures. Energy Rep. 7:3898–3910. doi: 10.1016/j.egyr.2021.06.067.
- Xie XC, Shen SF, Fu G, Shu XM, Hu J, Jia QS, Shi Z. 2022. Accident case data–accident cause model hybrid-driven coal and gas outburst accident analysis. Evidence from 84 accidents in China during 2008–2018. Process Saf Environ Prot. 164:67–90.
- Yan Z, Wang K, Zang J, Wang C, Liu A. 2019. Anisotropic coal permeability and its stress sensitivity. Int J Min Sci Technol. 29(3):507–511. doi: 10.1016/j.ijmst.2018.10.005.
- Yang DS, Qi XY, Chen WZ, Wang SG, Dai F. 2016. Numerical investigation on the coupled gas-solid behavior of coal using an improved anisotropic permeability model. J Nat Gas Sci Eng. 34:226–235. doi: 10.1016/j.jngse.2016.06.058.
- Yu T, Lu P, Sun JH, Deng Z. 2012. Measurement of effective drainage radius based on gas flow and pressure of boreholes. J Min Sci Eng. 29(4):596–600.
- Yuan L. 2016. Control of coal and gas outbursts in Huainan mines in China. A review. J Rock Mech Geotech Eng. 8(4):559–567. doi: 10.1016/j.jrmge.2016.01.005.
- Zeng J, Guo JC, Liu JS, Li W, Zhou YF, Tian JW. 2023. Anisotropic permeability model for coal considering stress sensitivity, matrix anisotropic internal swelling/shrinkage, and gas rarefaction effects. Energy Fuels. 37(4):2811–2832. doi: 10.1021/acs.energyfuels.2c04003.
- Zha ER, Zhang ZT, Zhang R, Wu SY, Li CB, Ren L, Gao MZ, Zhou JF. 2021. Long-term mechanical and acoustic emission characteristics of creep in deeply buried jinping marble considering excavation disturbance. Int J Rock Mech Min Sci. 139:104603. doi: 10.1016/j.ijrmms.2020.104603.
- Zhang C, Xu J, Peng S, Li Q, Yan F. 2019. Experimental study of drainage radius considering borehole interaction based on 3D monitoring of gas pressure in coal. Fuel. 239:955–963. doi: 10.1016/j.fuel.2018.11.092.
- Zhang Z, Zhang R, Xie H, Gao M, Zha E, Jia Z. 2017. An anisotropic coal permeability model that considers mining-induced stress evolution, microfracture propagation and gas sorption-desorption effects. J Nat Gas Sci Eng. 46:664–679. doi: 10.1016/j.jngse.2017.08.028.
- Zhao Y, Lin BQ, Liu T, Li QZ, Kong J. 2018. Gas flow field evolution around hydraulic slotted borehole in anisotropic coal. J Nat Gas Sci Eng. 58:189–200. doi: 10.1016/j.jngse.2018.08.006.
- Zhou HW, Rong TL, Wang LJ, Mou RY, Ren WG. 2020. A new anisotropic coal permeability model under the influence of stress, gas sorption and temperature: development and verification. Int J Rock Mech Min Sci. 132:104407. doi: 10.1016/j.ijrmms.2020.104407.