ABSTRACT
Shigella spp. causing bacterial diarrhea and dysentery are human enteroinvasive bacterial pathogens that are orally transmitted through contaminated food and water and cause bacillary dysentery. Although natural Shigella infections are restricted to humans and primates, several smaller animal models are used to analyze individual steps in pathogenesis. No animal model fully duplicates the human response and sustaining the models requires expensive animals, costly maintenance of animal facilities, veterinary services and approved animal protocols. This study proposes the development of the caterpillar larvae of Galleria mellonella as a simple, inexpensive, informative, and rapid in-vivo model for evaluating virulence and the interaction of Shigella with cells of the insect innate immunity. Virulent Shigella injected through the forelegs causes larvae death. The mortality rates were dependent on the Shigella strain, the infectious dose, and the presence of the virulence plasmid. Wild-type S. flexneri 2a, persisted and replicated within the larvae, resulting in haemocyte cell death, whereas plasmid-cured mutants were rapidly cleared. Histology of the infected larvae in conjunction with fluorescence, immunofluorescence, and transmission electron microscopy indicate that S. flexneri reside within a vacuole of the insect haemocytes that ultrastructurally resembles vacuoles described in studies with mouse and human macrophage cell lines. Some of these bacteria-laden vacuoles had double-membranes characteristic of autophagosomes. These results suggest that G. mellonella larvae can be used as an easy-to-use animal model to understand Shigella pathogenesis that requires none of the time and labor-consuming procedures typical of other systems.
Introduction
Shigella spp. are human-adapted pathogens that are transmitted orally through contaminated food and water and cause an acute inflammation of the distal colon and rectum. The symptoms of infection include moderate to severe diarrhea, dysentery, fever, abdominal cramps, tenesmus, nausea and various other gastrointestinal and constitutional symptoms. As low as 10–100 virulent Shigella can cause disease in some humans. Within 10–14 hours of infection, the level of serum TNF-α, a proinflammatory cytokine rises and the strain can be detected in diarrheal stools with either symptoms of fever, diarrhea and/or dysentery appearing in the next few hours.Citation1 Primates such as rhesus monkeys are also susceptible to oral Shigella infections and become very sick, sometimes resulting in death.Citation2,3 However, the multiplicity of infections in such animals is much higher, in the range of 109 to 1010 CFU.
Shigella is an enteroinvasive gram negative pathogen with a genome backbone very similar to E. coli. There are 4 major Shigella serogroups, S. flexneri, S. sonnei, S. dysenterieae and S. boydii and within each group, there are multiple serotypes and subtypes. Only S. sonnei has one serotype. Shigella virulence is an acquired trait and is due to the presence of a large mosaic 180–220 kb plasmid, also known as the invasion plasmid or the virulence plasmid (VP), composed of multiple virulence associated genes and a type III secretion system (T3SS). During pathogenesis, the presence of the VP allows the bacteria to initially be rescued from phagocytic macrophages and then to invade epithelial cells and subsequently spread from one cell to another.Citation4-8 These 2 essential steps in pathogenesis, invasion and spread, can be assayed in the laboratory using cultured cells. Plasmid-cured strains or strains lacking critical regions of the VP are noninvasive, avirulent and unable to cause disease. Shigella mutants that are invasive but lack the ability to spread intercellularly are significantly attenuated in virulence and are being evaluated as live attenuated vaccine candidates. Since humans and primates alone are naturally susceptible to infection, several small animal models have been developed to test different facets of Shigella pathogenesis and assist in vaccine development.Citation9-16
Rhesus macaques and more recently Aotus nancymae monkeys have been used to study oral Shigella infections.Citation2,9,17,18 However, the monkey models are very expensive, technically challenging and difficult to sustain. Alternately, several small animal model are being used to understand specific steps in pathogenesis. For example, the Sereny keratoconjunctivitis reaction in guinea pig eyes is a measure of virulence of a Shigella strain and requires both bacterial invasion and intercellular spread of the bacteria.Citation16 Sereny negative strains are significantly attenuated in virulence properties .19 The mice intranasal model is used to probe the inflammatory potential of a Shigella strain and has been previously used to evaluate the endotoxicity of LPS mutants.Citation20 Oral feeding of Shigella to 3-day old gnotobiotic piglets results in diarrhea and has been used to demonstrate the enterotoxicity of some Shigella strains although the animals become quickly resistant to the bacteria.Citation10 However, most small animal models do not simulate natural infections in humans. Additionally, monkeys, mice, guinea pigs and gnotobiotic piglets are hard to maintain, expensive and labor-intensive to work with and require regulatory approval and adequate veterinary resources. Thus, most of the small animal models have some drawbacks including practicality, ethical considerations, inter-laboratory reproducibility, ease of use, specialized training, and relevance to shigellosis. Due to these limitations, alternative in vivo infection model systems are being described that are easier and cheaper to house and maintain without the need for specific approvals.Citation21-23 This study focuses on insect model organisms that are attractive as they are relatively cheap, readily available, and present limited ethical issues. Like other animal models they typically shed light on relevant aspects of pathogenesis. It is believed that the epithelial cells of the insect larval midgut have similarities to the intestinal cells of the mammalian digestive system. The human and insect innate immune systems demonstrate several similarities which in-turn enable testing hypothesis across kingdoms.Citation24 Most insect species contain specialized cells known as haemocytes that phagocytose pathogens and form aggregates which encapsulate and neutralize foreign microorganisms.Citation25 Moreover, activated haemocytes can trigger a phenoloxidase (PO) melanization cascade.Citation24 As a result of bacterial infection haemocyte-mediated responses are complemented by the production and secretion of antimicrobial peptides (AMPs) by the insect fat body, an organ similar to the mammalian liver, activation of specific signaling pathways, and generation of reactive oxygen species.Citation25
The caterpillar larva (also called wax worms) of the Lepidopteran greater wax moth (Galleria mellonella) has become a widely adopted insect model to study several human pathogens including Listeria spp,Citation26 Streptococcus,Citation27 Legionella,Citation28 Campylobacter,Citation29 Yersinia,Citation30,31 Pseudomonas,Citation32-34 Enterococcus,Citation35 Staphylococcus,Citation36 Candida,Citation37,38 AspergillusCitation39-41 and other bacterial and fungal pathogens.Citation42-45 The life cycle of this organism comprises a larval stage that transforms within 6–8 weeks into pupae and finally into moth. The larvae are fairly large in size, the last instar larva is 2 cm long and 250 mg in weight and can be reared at various temperatures (20–30°C). G. mellonella larvae tolerate prolonged incubation at elevated temperatures (up to 42°C) and do not require anesthesia. The size of the larvae allows for easy manipulation and injection. After introduction of microbial pathogens the larvae is conveniently monitored for survival and larvae death is accompanied by unresponsiveness to physical stimuli and strong melanization. However, intrinsic differences between bacterial species in mechanisms of colonization, invasion, and pathogenicity means that it is necessary to examine the suitability of this model for the study of Shigella spp. In this study, the G. mellonella larvae is evaluated as an in-vivo model for studying Shigella infections. Its utility as a quick and inexpensive tool to evaluate virulence and compare isolates, species, and mutant Shigella strains is demonstrated.
Materials and methods
Bacterial strains and preparation for larval infections
The bacterial strains used in this study are listed in and came from the WRAIR collection. Bacterial strains were routinely grown on Tryptic Soy Agar (TSA) plates containing 0.05% Congo Red (w/v) and in Tryptic Soy Broth (TSB) medium. A Congo Red positive colony usually correlates with a virulent Shigella. A Form I Moseley strain represents an invasion-plasmid containing S. sonnei isolate whereas a Form II isolate refers to a plasmid-cured strain. Where appropriate, carbenicillin (50 μg mL−1) or chloramphenicol (35 μg mL−1) was added to the growth media. Shigella strains were cultured on TSA plates for 16 hours before inoculation in TSB. After overnight growth in TSB, mid-log phase cultures were derived by inoculating fresh TSB medium with an aliquot of the overnight growth at a 1:100 dilution. Unless specified differently bacteria growth was done at 37°C. The culture at mid-log phase of growth (O.D. = 0.4, ∼2 hours) was harvested by centrifugation (5,000 X g, 5 minutes), washed and diluted in Dulbecco's phosphate-buffered saline (D-PBS) to the desired optical density at 600 nm (OD600). The overnight growth culture represents the stationary growth phase. 1 O.D. unit was estimated to correspond to 5×108 CFU/ml and the actual concentration was verified by serial dilutions and plating.
Table 1. List of bacterial isolates and plasmids used in the G. mellonella infection assays.
G. mellonella larvae and infections
Final instar G. mellonella larvae were obtained from Vanderhorst Wholesale Inc. (St. Marys, OH) and stored in darkness at room temperature. Only healthy-looking caterpillars with no melanization were used in the experiments. Randomly assigned sets of 10 G. mellonella larvae were contained in a petri dish and injected with 5 μl of bacterial suspension using a modified Hamilton syringe with a 31 gauge 8 mm long needle. The plates containing the caterpillars were incubated at 37°C in the dark unless specified differently. All injections were administered via the last left pro-leg into the haemocoel. As controls, equal numbers of larvae kept in a second Petri dish were injected with PBS alone while a third group of larvae with no treatment were included in every experiment. Larvae were individually examined for pigmentation, and the number of live larvae was recorded at designated intervals. Larvae that did not show any movement in response to touch were considered dead. Assays proceeded for 48–72 hours and monitored twice a day. At least 3 independent replicates of each experiment were performed.
Assessment of bacterial proliferation in G. mellonella
At designated time post-infection (p.i.), 4 live infected larvae were pooled and killed. After homogenization in PBS+0.1% Triton X100 and a 10 minute incubation to allow for release of the bacteria, the sample was serially diluted in PBS and plated. Plates were incubated at 37°C for 16 hours and the number of CFU was recorded.
Plasmids and standard molecular biology techniques
Construction of S. flexneri ipaB mutant: An ipaB deletion of the wild-type S.flexneri strain 2457T was made by the lambda-red recombineering method.Citation46,47 The chloramphenicol cassette/linear DNA was PCR amplified from the pKD3 plasmid with the primers ipaB KO for and ipaBC KO rev (CATTCTCCTTATTTGTATCAAGCAGTAGTTTGTTGC-AAAATTGCTTTTGCGTGTAGGCTGGAGCTGCTTCG and AAAGCACAATCATACTTGGACGCAATT-CAGGATATCAAGGAGTAATTATTCATATGAATATCCTCCTTAGT). The ipaB gene was cloned by PCR amplification into pUC18 (IpaB clone). The plasmid was transformed into 2457TΔipaB and the expression of IpaB was evaluated by Western blot analysis using monoclonal anti-IpaB antibodies. Transformation of Shigella strains with pGFPuv (Clontech) was done according to standard molecular biology method and GFP expression was checked spectroscopically.
Haemocytes quantification
Haemolymph was extracted at t = 0 and t = 24 hours p.i. Trypan blue (0.02% [vol/vol] in PBS) was added to the cells in the hemolymph and incubated at room temperature for 10 min. Viable cells were enumerated using a hemocytometer (TC-10, Bio-rad), and each sample was analyzed in triplicate. The average of 3 independent experiments are reported.
Fixation and staining of G. mellonella
G. mellonella larvae were fixed in 4% Paraformaldehyde for 3–4 weeks at room temperature, processed on Sakura Tissue-Tek VIP processor, embedded, sectioned and stained with hematoxylin and eosin (H&E) by Sakura Tissue-Tek Prisma stainer.
Transmission electron microscopy (TEM)
Haemolymph was extracted from infected G. mellonella larvae. Cells were spun down, washed once with PBS, and fixed in 1% formaldehyde and then 4% glutarladehyde buffered with sodium phosphate pH 7.2. Samples were post-fixed with 2% OsO4 and then post stained with 2% uranyl acetate for TEM. The material was sectioned on Leica microtome and viewed on 1400 Jeol electron microscope.
Fluorescence and confocal microscopy
Bacterial cells were transformed with pGFPuv to constitutively express GFP (2457T-GFP). Haemolymph was extracted from G. mellonella larvae infected with 2457T-GFP at the designated time points. Haemocytes were spun down, washed once with PBS, mounted on glass coverslips and viewed directly using a fluorescence microscope (Zeiss, AxioObserver.Z1). For confocal microscopy G. mellonella larvae were fixed and sectioned as written above, labeled with monoclonal anti-S.flexneri antibody followed by Alexa-fluor 594 conjugated secondary antibody (goat anti-mouse antibody) and detected by Olympus Fluoview FV1200.
Statistical analysis and LD50 calculations
Individual statistical analysis of data are indicated either in the text or in the Figure legends. The Spearman-Karber method (Haas 1989) was used to estimate the Lethal Dose (LD) causing 50% mortality (LD50) of the infected G. mellonella larvae.
Results
Galleria mellonella larvae are susceptible to Shigella spp. infection
G. mellonella larvae were injected with representative virulent strains of 3 major Shigella species. The larvae survival was monitored over time following injection of the bacterial suspension as shown in a representative experiment (panel A). S. flexneri 2a strain 2457T, S. sonnei strain Moseley, and S. dysenteriae 1 strain 1617 caused time-dependent larval death that was assayed by touch-induced lack of larval movement. In most cases, larval mortality was accompanied by melanization of the larvae (panel B). Compared to the other 2 species of Shigella, strain 1617 induced higher mortality at the same bacterial inoculum dose indicating an increased virulence of this strain in this model (panel A). No mortality was observed in the control PBS-injected larvae. An avirulent S. flexneri 2a strain BS103, under the same conditions behaved like PBS (panel B). Oral force feeding of larvae with 2457T did not show any specific phenotype (data not shown). These results demonstrate that, with the limited number of strains studied here, G. mellonella larvae succumb to Shigella infection and appear to show strain-specific differences.
Figure 1. Shigella spp. infection of G. mellonella induces strain dependent lethality. Panel A, Log phase cultures of S. flexneri 2a strain 2457T (––▪––), S. sonnei strain Moseley (–▴–), and S. dysenteriae 1 strain 1617 (•–▾–•) were harvested and 7 × 105 CFU bacteria were injected into the larva. Each group contained 10 larvae. PBS mock injection was used as a negative control (--•--). Survival was monitored over 54 hours p.i. Panel B, photographic images of the G. mellonella larvae injected with 7×105 CFU/larvae of 2457T, BS103 or PBS at 24 hours p.i., showing melanization of the larvae by injection of 2457T.
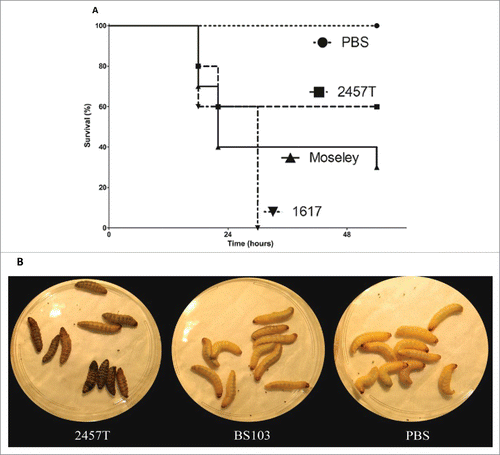
Shigella-induced larval mortality is dose dependent and requires the presence of the Shigella virulence plasmid
Infection with increasing doses of 2457T (and Moseley Form I, data not shown) resulted in increased rates of larval mortality indicating that larval death was dose dependent (panel A). Infection with 2 × 106 CFU of 2457T resulted in 100% mortality of the larvae within 24–30 hours while mortality was reduced to 10% in larvae injected with 1.8 × 104 CFU of 2457T, even with increased time of infection to more than 40 hours (panel A). VP-cured strains BS103 (and Moseley Form II, data not shown) caused lower rates of mortality, demonstrating the role of the invasion plasmid in causing early larval death (panels A-B). A similar profile was seen when G. mellonella was injected with S. flexneri 5a strain M90T-W, and an isogenic VP-cured strain M90T-55. M90T-A3, that lacks a portion of the VP, is less virulent than M90T-W (data not shown). These differences between plasmid-containing and plasmid-cured strains were reflected in the LD50 values for larval death ().
Figure 2. Dose, time and IpaB dependence of G. mellonella larvae death after infection with Shigella spp. G. mellonella larvae were injected with different concentrations of panel A, 2457T, panel B, BS103, panel C, 2457TΔipaB and panel D, 2457T ΔipaB : : ipaB. Larval death was monitored over time and scored by lack of movement after a touch. Significance was determined by the Log Rank test.
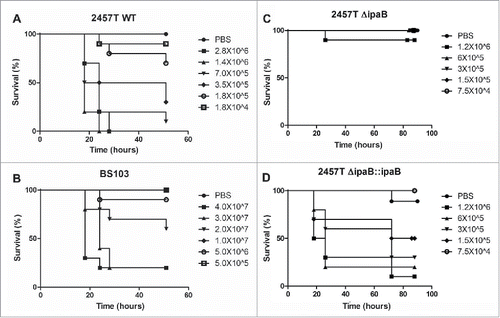
Table 2. LD50 of Shigella strains in the wax worm.
Shigella IpaB is required for G. mellonella larvae mortality
IpaB is a T3SS effector protein that is present on the VP and is critical for epithelial cell invasion and release of the bacteria from endocytic vacuoles. IpaB mutants are noninvasive and hence avirulent. G. mellonella were injected with different doses of 2457T, 2457TΔipaB, 2457T ΔipaB::ipaB and BS103 ( panels A-D). Larvae injected with 2457TΔipaB exhibited very low rates of mortality (panel C). 2457T at a dose of 1.4 × 106 CFU resulted in 100% mortality within 24–30 hours of infection. However, only 10% of the larvae died when infected with a similar dose of a 2457TΔipaB mutant over 48 hours of infection (panel C). Complementation of the ΔipaB mutant with an ipaB gene (2457T ΔipaB::ipaB ) restored larval mortality rates indicating that ipaB-mediated virulence is required for larval death (panel D). The significance of the differences in the survival rates, performed using the Log-Rank test, and the p-values derived for larvae infected with 2457T, 2457TΔipaB and 2457T ΔipaB :: ipaB at 1.2 × 106 CFU, 6 × 105 CFU, 3 × 105 CFU and 1.5 × 105 CFU were p < 0.0001, 0.0001, 0.004 and 0.026, respectively. S. sonnei ipaB mutants were also attenuated in this infection model (data not shown).
Effect of bacterial growth temperature and growth phase on Shigella virulence in G. mellonella
G. mellonella larvae have the advantage of being able to grow at a broad range of temperatures. And as with many pathogens, Shigella virulence is temperature regulated. 2457T grown at 37°C or 30°C in TSB medium was used to infect G. mellonella. After infection, the larvae were incubated at the same temperatures as the bacterial growth, at either 37°C or 30°C. The larval mortality rates infected with 1 × 106 CFU of 2457T are shown in . Infection with 2457T that was grown at 30°C did not cause larval death at 24 hours p.i. even at doses of more than 4 times the LD50 of the strain at 37°C (data not shown). At 72 hours p.i. the larval mortality at 30°C did not exceed 20% indicating that bacterial growth temperatures determined the outcome of the infection ().
Figure 3. G. mellonella larval death is dependent on bacterial growth temperature and bacterial growth phase. G. mellonella larvae were injected with log (LP) and stationary phase (SP) 2457T that were grown at 37°C and 30°C. After injection, the larvae were incubated at either 37°C or 30°C, and death was monitored over time.
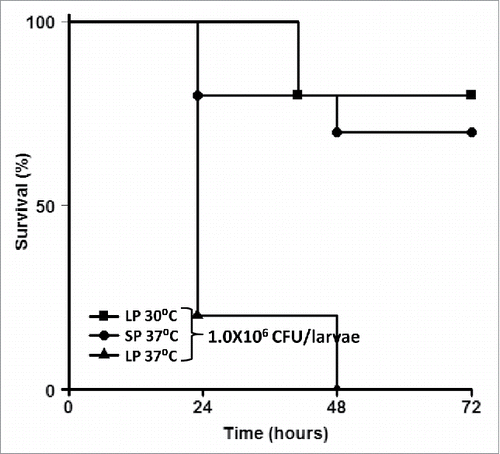
Larval mortality was also dependent upon the growth phase of the bacteria (). Overnight, stationary phase bacterial cells induced much lower mortality than bacterial cells grown to log phase (OD600 = 0.4–0.6) ().
Virulent Shigella replicates within G. mellonella and induces haemocyte cell-death
To determine the fate of the bacteria within the wax worm larvae, Shigella-infected larvae were killed and aliquots of PBS/TritonX-100 larval lysates were plated out on TSA plates. The number of 2457T bacteria recovered from the lysates increased with time by more than one log indicating that the VP-containing strain replicates within G. mellonella while at the same time resulting in larval death ( panels A-B). In contrast, the number of BS103 recovered post infection reduced by more than a log indicating that the VP-cured noninvasive strain does not replicate and persist and is cleared from the larvae (panel B). At the same time enumeration of larval haemocyte counts indicated that infection of G. mellonella with 2457T resulted in the drastic reduction of haemocyte counts in contrast to larvae infected with BS103 where the haemocyte counts remained almost unchanged (panel C).
Figure 4. Bacterial replication and haemocyte depletion after Shigella infection in Galleria larva. G. mellonella larvae were infected with PBS, 1.0 × 106 CFU of 2457T and 1.0 × 106 CFU of BS103. After 24 hours, larvae survival rate, bacterial load in larvae and haemocyte counts were recorded. 4 live larvae at t = 0 and t = 24 were killed for these assays. Panel A represents percent survival of larvae at t = 0 and t = 24. Panel B represents changes in bacterial load in whole body at the 2 time points. Panel C represents per cent haemocyte counts at the 2 time points. These experiments were repeated 4 times. Each error bar represents one standard deviation from the mean. Differences between wild-type and BS103 were assessed for statistical significance by using a 2-sided t-test and assuming unequal variances. *p<0.05. Dark-filled bars t = 0, light gray bars, t = 24.
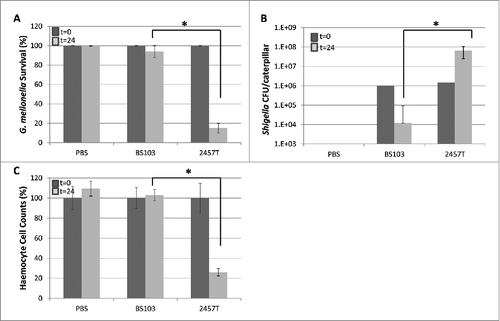
Fluorescence microscopy of infected larvae
G. mellonella larvae were infected with 2457T carrying a constitutive GFP-expressing plasmid. The introduction of the plasmid had no effect on bacterial growth inside the larvae or the LD50 values (data not shown). The hemolymph was extracted and observed under fluorescence microscopy. Soon after injection, GFP-tagged bacterial cells were observed in close association with haemocytes. At 2 hours post infection many of the haemocytes contained fluorescent 2457T could be seen ( panels A-F). Within a few hours the number of bacterial cells within the haemocytes increased, the percentage of haemocytes containing bacterial cells also increased while the number of free floating bacterial cells diminished significantly (data not shown). At 24 hours post infection very few haemocytes were seen and those that remained contained multiple GFP-tagged bacteria.
Figure 5. S. flexneri replicates within G. mellonella haemocytes. 2457T was tagged with a plasmid encoding GFP and used to infect larvae of G. mellonella at a dose of 1 × 106 CFU/larvae. The larvae were killed 2 hours post infection. Samples of the larval hemolymph were observed using panel A, bright field and panel B, fluorescent microscopy. The merged image in panel C shows GFP-tagged bacteria within the haemocytes. Panels D, E, and F represent a different field where multiple haemocytes can be seen with GFP-laden bacteria. Panel F represents the merged image of panels D and E.
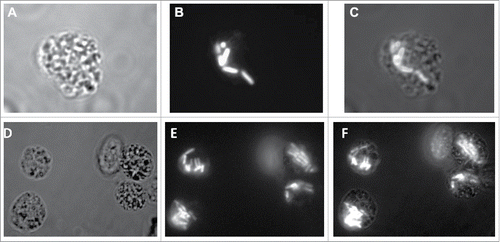
S. flexneri interactions with G. mellonella
Whole body sections of an infected G. mellonella larvae were analyzed using H&E staining accompanied by immune-staining confocal microscopy. At 2 hours post infection with 2457T, H&E stained cross sections of larvae revealed clusters of haemocytes exhibiting phagocytosis of bacteria (finely stippled blue dots throughout section) with small area of melanization around haemocyte aggregates (panel A). At 20 hours post infection no individual haemocytes were seen and those that were observed were present in nodules, some attached to organ structures with evidence of melanization (panels B-C). Tubular organs became surrounded by a load of bacteria (panel B). In contrast, cross sections of H&E-stained uninfected larva looked healthy with individually distributed haemocytes showing no noticeable aggregates or melanization (panel D). To confirm that the bacteria observed in the fixed sections of the infected G. mellonella were S. flexneri 2a, sections were stained with an 2457T-specific LPS antibody. Confocal microscopy of cross sections of the worm indicated that some 2457T bacteria localized in the proximity of the tissue surrounding tubular organs (panels A-D).
Figure 6. H&E-stained sections of G. mellonella after Shigella infection. G. mellonella were infected with 1 × 106 CFU/larvae of 2457T. After 2 hours and 20 hours of growth at 37°C the larva were fixed and stained. Panel A, 2 hours p.i. Haemocytes slightly more clustered than observed in uninfected hemolymph (panel D). Panels B and C, 20 hours p.i. Haemocytes form nodules with evidence of melanization (black arrow in panel B and inset in panel B) and a load of bacteria around tubular organelle (red arrows in panel B). Panel D, PBS injected sample. No bacteria are observed in haemocoel and no obvious aggregates of haemocytes or melanization in uninfected samples.
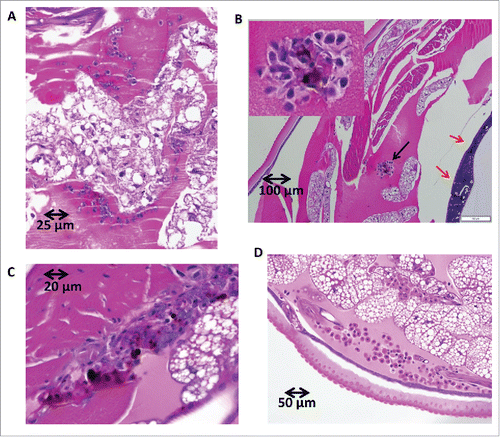
Figure 7. Immunofluorescence microscopy of 2457T-infected G. mellonella. G. mellonella larvae were infected with 1.0 × 106 CFU of 2457T/larvae. After 20 hours the larvae were fixed and stained using monoclonal anti-S. flexneri LPS antibody as primary antibody and Alexa-fluor 594 conjugated secondary antibody. Panel A, autofluorescence of uninfected G. mellonella section, panel B, autofluorescence of infected larvae section, panel C, immunofluorescence of anti S. flexneri specific antibodies and panel D, higher-magnification view of the square marked region in panel C showing red-labeled bacteria clustering around tubular organs.
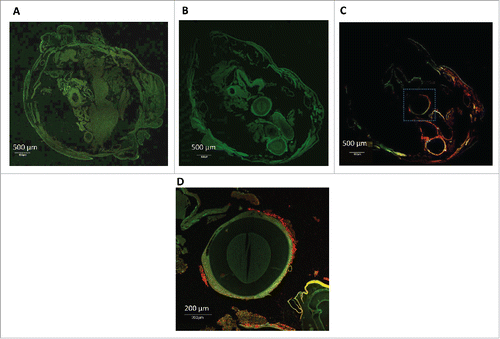
Transmission electron microscopy (TEM).
Haemocytes at different times post infection were extracted and analyzed using TEM ( panels A-H). The electron micrograph demonstrates internalization of the 2457T strain within a distinct vacuole lying within the cytoplasm of a granular haemocyte (, panels A-F). The cytoplasm shows numerous mitochondria as well as Golgi and rough endoplasmic reticulum (RER) studded with distinct ribosomes ( panels A-F). Several darker and less darker vesicular bodies are seen which in previous publications have been referred to as dense granules and structured granules.Citation25 The structured granules appear to have “microtubular” structures within the organelle. At 8 hours after infection with 2457T, the haemocyte contains multiple bacteria within enclosed vacuoles. In some cases the bacterium is seen replicating within the vacuole ( panel C, F). Most of the bacteria-laden vacuoles are encased within a distinct double membrane characteristic of autophagosomes that appears in some cases to have a fibrillar edge (see thin arrow in enlarged panels D-F). While some vacuoles within the infected haemocyte are clearly empty or have faint granular material, other darker vesicles present within individual haemocytes appear to contain multiple smaller bodies or organelles. Several of the vacuoles have whorls of filaments forming concentric rings (“onion rings”?) (panels B-C, E-F). In some cases these vacuoles with onion rings along with the structured granules appear to lie in close association with the bacteria-laden vacuoles. No intact bacteria was seen in the haemocytes of BS103 infected larva ( panels G-H). In some of these BS103-infected haemocytes there were vacuoles that appear to contain what looks like disintegrating bacteria. However, further analysis of the material within the BS103-infected haemocytes is needed ( panels G-H). Unlike the haemocyte vacuoles present within the 2457T-infected larvae, haemocytes from BS103-infected larvae looked intact after 8 hours p.i., and did not contain autophagosomal-like structures or vacuoles with “onion rings” ( panels G-H).
Figure 8. TEM of G. mellonella larval haemocytes after Shigella infection. Haemocytes of G. mellonella infected with 1.0 × 106 CFU/larva, were fixed, sectioned and observed under the TEM. Panel A-F, sections of G. mellonella larval haemocytes infected with S. flexneri 2a 2457T. The lower panels D-F represent higher magnification images of bacteria-containing regions in the corresponding upper panels A-C (wide arrows-structures appearing as onion rings, arrow heads-replicating bacteria, thin arrow-fibrillar edges). Panels G-H, larval haemocytes infected with BS103.
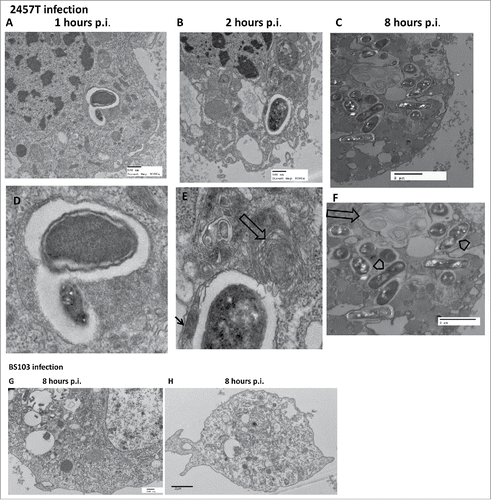
Discussion
In this study the larvae of the greater wax moth G. mellonella served as a non-vertebrate animal host model for Shigella infections. G. mellonella was chosen because it has several advantages over other non-vertebrate infection systems such as Drosophila melanogaster or Caenorhabditis elegans. The larvae are quite large in size, commercially available, can be easily stored at room temperature and do not need to be propagated in the laboratory.Citation43 G. mellonella larvae can be incubated at 37°C, a temperature that is necessary for virulence of many pathogens such as Shigella.
The larval death was dose dependent, strain dependent, and required IpaB. VP-cured Shigella required 2–3 log higher doses to cause larval death that occurred over a longer period of time. Virulent Shigella replicates within the larvae and results in haemocyte cell death, melanization and ultimately larval death within 48–72 hours. The bacteria-laden vacuoles within 2457T-infected larval haemocytes appear to be encased within a double-membraned structure that resembles autophagosomes, reminiscent of what has been recently described in Shigella-infected zebra fish.Citation21 Some of the vacuoles containing individual or replicating bacteria resemble endocytic vacuoles described previously in Shigella-infected mouse and human macrophages and in rabbit PMNs. At 8 hours after infection with 2457T the haemocytes appear necrotic concomitant with an increase in the number of bacteria. At 24 hours of infection haemocyte counts reduced drastically. Molecular and biochemical analysis is required to understand the mechanism of Shigella-mediated haemocyte cell death although a recent report describes apoptosis of Galleria haemocytes to occur upon Shigella infection.Citation48 Avirulent strains were not recovered from the haemocytes at later times of infection and were not seen as intact bacteria within the haemocyte vacuoles. Unlike 2457T-infected haemocytes, BS103 infected haemocytes larvae showed large vacuoles with disintegrating bacteria. It is not clear whether BS103 bacteria are taken up and immediately destroyed within the phagocytic vacuole similar to what has been described with Galleria larvae infected with Legionella pneumophila. In this system wild type strains replicated within the larvae while type IV secretion system mutants were rapidly cleared.Citation49-51
Unlike C. elegans, G. mellonella has a relatively complex innate immune response, with homology to mammalian innate immunity.Citation52 Circulating granular haemocytes that function as mammalian macrophages as well as plasmatocytes that can adhere to and encapsulate foreign bodies are the main drivers of the larval humoral and cellular defense responses.Citation24,25,52-58 Bacterial infections can be cleared by haemocyte-mediated aggregation and nodulation, or by phagocytosis of the bacterial strain, resulting in production and release of antimicrobial peptides, haemocyte cell death and in some cases melanization of the larval body.Citation27,28,37,59-66 Some of the physical features such as phagocytosis of the bacetrial strain, haemocyte-aggregation, nodulation, and melanization were also observed in Shigella-infected larvae; however future work will determine whether specific AMPs are increased after infection.
G. mellonella larvae has been widely adopted as a simple and inexpensive model for assessing host-pathogen interactions that includes bacterial, plant and fungal pathogens.Citation67,68 Different Shigella strains, such as S. flexneri 2a strain 2457T, S. flexneri 5 strain M90T, S. sonnei strain Moseley and S. dysenteriae 1 strain 1617 as well as mutants of these strains showed different kinetics of survival and larval mortality suggesting that this model is useful in discriminating between virulent as well as avirulent strains. Similar pattern of discrimination between strains of the same species in Galleria has been seen with other organisms such as Yersinia, Campylobacter and H. pylori.Citation29,30,31,69,70 For example, OxyR and PhoP mutants of Y.pestis are less virulent in Galleria, and H. pylori VacA, CagA, CagE, cag PAI, or urease mutants, were less virulent than the respective parental strain.Citation71
Like mammalian cells, Galleria can recognize microbe-associated molecular patterns by receptors such as Toll and peptidoglycan recognition proteins.Citation72,73 Galleria larvae also exhibit apoptosis and autophagy as cellular processes that, like in mammalian cells, could potentially be activated during microbial infection.Citation74,75 Thus, bacterial factors that enable survival in this insect host are likely to be directly relevant to human infection.Citation75,76 More recently, a cDNA encoding a caspase 1 has been cloned and sequenced from the midgut of G. mellonella larvae and shown to be similar to human ced-3 and proteins homologous to human NADPH oxidase system have also been shown to exist in G. mellonella larvae.Citation77,78 Thus, it appears that mechanisms used by mammalian neutrophils and macrophages including an oxidative burst, production of lysozyme and numerous antimicrobial peptides that kill ingested microbes are also in play in Galleria haemocytes. Future studies will be directed toward which of these defense mechanisms are utilised during Shigella infections.
Obviously there are differences since Galleria does not have an adaptive immune system and lacks antibodies and Galleria innate immune system lacks natural killer cells, dendritic cells and cytokines that play a crucial role in the human immune response.Citation67 Recently, a comprehensive transcriptome of G. mellonella genes that participate in its immune response has been identified and will help to further studies in this host.Citation66 This transcriptome will be useful since Galleria does not have a fully sequenced genome. The response of Galleria larvae to virulent strains of Shigella was sensitive to both temperature and growth phase of the bacteria before infection, 2 factors that also influence virulence in mammalian models.Citation79 However, it is known that environmental factors such as heat, nutrient deprivation and other kinds of stress also influence the cellular and humoral immune response of the larvae.Citation54,80-83 suggesting that careful interpretation of data arising from effect of specific environmental factors is needed.Citation84
Disclosure of potential conflicts of interest
No potential conflicts of interest were disclosed.
Acknowledgments
We thank Edward Asafo-Adjei for TEM imaging, Matthew C. Wise for confocal microscopy, LTC Mark Smith for wax worm related discussions, Cassandra Federman for help with wax worms and Eran Barnoy for statistical analysis and survival curves.
Funding
This work was supported by funding from The Military Infectious Diseases Research Program (MIDRP). The opinions or assertions contained herein are the private views of the author, and are not to be construed as official, or as reflecting true views of the Department of the Army or the Department of Defense.
References
- Munoz C, Baqar S, van de Verg L, Thupari J, Goldblum S, Olson JG, Taylor DN, Heresi GP, Murphy JR. Characteristics of Shigella sonnei infection of volunteers: signs, symptoms, immune responses, changes in selected cytokines and acute-phase substances. Am J Trop Med Hyg 1995; 53:47-54; PMID:7542845
- Formal SB, Oaks EV, Olsen RE, Wingfield-Eggleston M, Snoy PJ, Cogan JP. Effect of prior infection with virulent Shigella flexneri 2a on the resistance of monkeys to subsequent infection with Shigella sonnei. J Infect Dis 1991;164:533-7; PMID: 1869840; http://dx.doi.org/10.1093/infdis/164.3.533
- Dinari G, Hale TL, Austin SW, Formal SB. Local and systemic antibody responses to Shigella infection in rhesus monkeys. J Infect Dis 1987;155:1065-9; PMID: 3549918; http://dx.doi.org/10.1093/infdis/155.5.1065
- Ashida H, Ogawa M, Mimuro H, Kobayashi T, Sanada T, Sasakawa C. Shigella are versatile mucosal pathogens that circumvent the host innate immune system. Curr Opin Immunol 2011;23:448-55; PMID: 21763117; http://dx.doi.org/10.1016/j.coi.2011.06.001
- Ashida H, Mimuro H, Sasakawa C. Shigella manipulates host immune responses by delivering effector proteins with specific roles. Front Immunol 2015; 6:219; PMID: 25999954; http://dx.doi.org/10.3389/fimmu.2015.00219
- Sansonetti PJ. The bacterial weaponry: lessons from Shigella. Ann N Y Acad Sci 2006;1072: 307-12; PMID: 17057210; http://dx.doi.org/10.1196/annals.1326.025
- Phalipon A, Sansonetti PJ. Shigella's ways of manipulating the host intestinal innate and adaptive immune system: a tool box for survival? Immunol Cell Biol 2007;85:119-29
- Puhar A, Tronchere H, Payrastre B, Nhieu GT, Sansonetti PJ. A Shigella effector dampens inflammation by regulating epithelial release of danger signal ATP through production of the lipid mediator PtdIns5P. Immunity 2013; 39:1121-31; PMID: 24332032; http://dx.doi.org/10.1016/j.immuni.2013.11.013
- Gregory M, Kaminski RW, Lugo-Roman LA, Galvez Carrillo H, Tilley DH, Baldeviano C, Simons MP, Reynolds ND, Ranallo RT, Suvarnapunya AE, et al. Development of an Aotus nancymaae model for Shigella vaccine immunogenicity and efficacy studies. Infect Immun 2014;82:2027-36; PMID: 24595138; http://dx.doi.org/10.1128/IAI.01665-13
- Jeong KI, Venkatesan MM, Barnoy S, Tzipori S. Evaluation of virulent and live Shigella sonnei vaccine candidates in a gnotobiotic piglet model. Vaccine 2013;31:4039-46; PMID: 23684833; http://dx.doi.org/10.1016/j.vaccine.2013.04.076
- Levenson VJ, Mallett CP, Hale TL. Protection against local Shigella sonnei infection in mice by parenteral immunization with a nucleoprotein subcellular vaccine. Infect Immun 1995;63:2762-5; PMID: 7790095
- Ranallo RT, Fonseka CP, Cassels F, Srinivasan J, Venkatesan MM. Construction and characterization of bivalent Shigella flexneri 2a vaccine strains SC608(pCFAI) and SC608(pCFAI/LTB) that express antigens from enterotoxigenic Escherichia coli. Infect Immun 2005; 73:258-67; PMID: 15618162; http://dx.doi.org/10.1128/IAI.73.1.258-267.2005
- Ranallo RT, Thakkar S, Chen Q, Venkatesan MM. Immunogenicity and characterization of WRSF2G11: a second generation live attenuated Shigella flexneri 2a vaccine strain. Vaccine 2007; 25:2269-78; PMID: 17229494; http://dx.doi.org/10.1016/j.vaccine.2006.11.067
- Mallett CP, Hale TL, Kaminski RW, Larsen T, Orr N, Cohen D, Lowell GH. Intranasal or intragastric immunization with proteosome-Shigella lipopolysaccharide vaccines protects against lethal pneumonia in a murine model of Shigella infection. Infect Immun 1995; 63:2382-6;. PMID: 7768627
- Hartman AB, Powell CJ, Schultz CL, Oaks EV, Eckels KH. Small-animal model to measure efficacy and immunogenicity of Shigella vaccine strains. Infect Immun 1991;59:4075-83; PMID: 1937767
- Hartman AB, Van de Verg LL, Collins HH, Jr., Tang DB, Bendiuk NO, Taylor DN, Powell CJ. Local immune response and protection in the guinea pig keratoconjunctivitis model following immunization with Shigella vaccines. Infect Immun 1994; 62:412-20; PMID: 7507892
- Ranallo RT, Kaminski R, Baqar S, Dutta M, Lugo-Roman LA, Boren T, Barnoy S, Venkatesan MM. Oral administration of live Shigella vaccine candidates in rhesus monkeys show no evidence of competition for colonization and immunogenicity between different serotypes. Vaccine 2014; 32:1754-60; PMID: 24522159; http://dx.doi.org/10.1016/j.vaccine.2013.12.068
- Collins TA, Barnoy S, Baqar S, Ranallo RT, Nemelka KW, Venkatesan MM. Safety and colonization of two novel VirG(IcsA)-based live Shigella sonnei vaccine strains in rhesus macaques (Macaca mulatta). Comp Med 2008; 58:88-94;. PMID: 19793462
- Venkatesan MM, Ranallo RT. Live-attenuated Shigella vaccines. Expert Rev Vaccines 2006; 5:669-86; PMID: 17181440; http://dx.doi.org/10.1586/14760584.5.5.669
- Ranallo RT, Kaminski RW, George T, Kordis AA, Chen Q, Szabo K, Venkatesan MM. Virulence, inflammatory potential, and adaptive immunity induced by Shigella flexneri msbB mutants. Infect Immun 2010; 78:400-12; PMID: 19884336; http://dx.doi.org/10.1128/IAI.00533-09
- Mostowy S, Boucontet L, Mazon Moya MJ, Sirianni A, Boudinot P, Hollinshead M, Cossart P, Herbomel P, Levraud JP, Colucci-Guyon E. The zebrafish as a new model for the in vivo study of Shigella flexneri interaction with phagocytes and bacterial autophagy. PLoS Pathog 2013; 9:e1003588; PMID: 24039575; http://dx.doi.org/10.1371/journal.ppat.1003588
- George DT, Behm CA, Hall DH, Mathesius U, Rug M, Nguyen KC, Verma NK. Shigella flexneri infection in Caenorhabditis elegans: cytopathological examination and identification of host responses. PLoS One 2014; 9:e106085; PMID: 25187942; http://dx.doi.org/10.1371/journal.pone.0106085
- Fung CC, Octavia S, Mooney AM, Lan R. Virulence variations in Shigella and enteroinvasive Escherichia coli using the Caenorhabditis elegans model. FEMS Microbiol Lett 2015; 362:1-5; PMID: 25673655; http://dx.doi.org/10.1093/femsle/fnu045
- Lavine MD, Strand MR. Insect haemocytes and their role in immunity. Insect Biochem Mol Biol 2002; 32:1295-309; PMID: 12225920; http://dx.doi.org/10.1016/S0965-1748(02)00092-9
- Ribeiro C, Brehelin M. Insect haemocytes: what type of cell is that? J Insect Physiol 2006; 52:417-29; PMID: 16527302; http://dx.doi.org/10.1016/j.jinsphys.2006.01.005
- Browne N, Kavanagh K. Developing the potential of using Galleria mellonella larvae as models for studying brain infection by Listeria monocytogenes. Virulence 2013;4:271-2; PMID: 23552811; http://dx.doi.org/10.4161/viru.24174
- Loh JM, Adenwalla N, Wiles S, Proft T. Galleria mellonella larvae as an infection model for group A streptococcus. Virulence 2013; 4:419-28.
- Chmiel E, Palusinska-Szysz M, Zdybicka-Barabas A, Cytrynska M, Mak P. The effect of Galleria mellonella hemolymph polypeptides on Legionella gormanii. Acta Biochim Pol 2014; 61:123-7; PMID: 24649482
- Senior NJ, Bagnall MC, Champion OL, Reynolds SE, La Ragione RM, Woodward MJ, Salguero FJ, Titball RW. Galleria mellonella as an infection model for Campylobacter jejuni virulence. J Med Microbiol 2011;60:661-9; PMID: 21233296; http://dx.doi.org/10.1099/jmm.0.026658-0
- Erickson DL, Russell CW, Johnson KL, Hileman T, Stewart RM. PhoP and OxyR transcriptional regulators contribute to Yersinia pestis virulence and survival within Galleria mellonella. Microb Pathog 2011; 51:389-95; PMID: 21964409; http://dx.doi.org/10.1016/j.micpath.2011.08.008
- Hurst MR, Beattie AK, Jones SA, Hsu PC, Calder J, van Koten C. Temperature-Dependent Galleria mellonella Mortality as a Result of Yersinia entomophaga Infection. Appl Environ Microbiol 2015; 81:6404-14; PMID: 26162867; http://dx.doi.org/10.1128/AEM.00790-15
- Benthall G, Touzel RE, Hind CK, Titball RW, Sutton JM, Thomas RJ, Wand ME. Evaluation of antibiotic efficacy against infections caused by planktonic or biofilm cultures of Pseudomonas aeruginosa and Klebsiella pneumoniae in Galleria mellonella. Int J Antimicrob Agents 2015;46:538-45; PMID: 26364845; http://dx.doi.org/10.1016/j.ijantimicag.2015.07.014
- Chadwich JS. An assessment of the ability of individual moieties of Pseudomonas aeruginosa endotoxin to induce immunity in larvae of Galleria mellonella. J Invertebr Pathol. 1971; 17:299-300; PMID: 4995870; http://dx.doi.org/10.1016/0022-2011(71)90113-3
- Koch G, Nadal-Jimenez P, Cool RH, Quax WJ. Assessing Pseudomonas virulence with nonmammalian host: Galleria mellonella. Methods Mol Biol 2014;1149:681-8; PMID: 24818942
- Lebreton F, Le Bras F, Reffuveille F, Ladjouzi R, Giard JC, Leclercq R, Cattoir V. Galleria mellonella as a model for studying Enterococcus faecium host persistence. J Mol Microbiol Biotechnol 2011; 21:191-6; PMID: 22286046; http://dx.doi.org/10.1159/000332737
- Gibreel TM, Upton M. Synthetic epidermicin NI01 can protect Galleria mellonella larvae from infection with Staphylococcus aureus. J Antimicrob Chemother 2013; 68:2269-73; PMID: 23711896
- Amorim-Vaz S, Delarze E, Ischer F, Sanglard D, Coste AT. Examining the virulence of Candida albicans transcription factor mutants using Galleria mellonella and mouse infection models. Front Microbiol 2015; 6:367; PMID: 25999923; http://dx.doi.org/10.3389/fmicb.2015.00367
- Jacobsen ID. Galleria mellonella as a model host to study virulence of Candida. Virulence 2014; 5:237-9; PMID: 24384470; http://dx.doi.org/10.4161/viru.27434
- Maurer E, Browne N, Surlis C, Jukic E, Moser P, Kavanagh K, Lass-Flörl C, Binder U. Galleria mellonella as a host model to study Aspergillus terreus virulence and amphotericin B resistance. Virulence 2015; 6:591-8; http://dx.doi.org/10.1080/21505594.2015.1045183
- Fallon JP, Troy N, Kavanagh K. Pre-exposure of Galleria mellonella larvae to different doses of Aspergillus fumigatus conidia causes differential activation of cellular and humoral immune responses. Virulence 2011; 2:413-21; PMID: 21921688; http://dx.doi.org/10.4161/viru.2.5.17811
- Jackson JC, Higgins LA, Lin X. Conidiation color mutants of Aspergillus fumigatus are highly pathogenic to the heterologous insect host Galleria mellonella. PLoS One 2009; 4:e4224; PMID: 19156203; http://dx.doi.org/10.1371/journal.pone.0004224
- Ramarao N, Nielsen-Leroux C, Lereclus D. The insect Galleria mellonella as a powerful infection model to investigate bacterial pathogenesis. J Vis Exp 2012: 11:e4392;. PMID: 23271509
- Tsai CJ, Loh JM, Proft T. Galleria mellonella infection models for the study of bacterial diseases and for antimicrobial drug testing. Virulence 2016; 7:214-29; PMID: 26730990; http://dx.doi.org/10.1080/21505594.2015.1135289
- Fallon J, Kelly J, Kavanagh K. Galleria mellonella as a model for fungal pathogenicity testing. Methods Mol Biol 2012; 845:469-85; PMID: 22328396
- Binder U, Maurer E, Lass-Florl C. Galleria mellonella: An invertebrate model to study pathogenicity in correctly defined fungal species. Fungal Biol 2016; 120:288-95
- Ranallo RT, Barnoy S, Thakkar S, Urick T, Venkatesan MM. Developing live Shigella vaccines using lambda Red recombineering. FEMS Immunol Med Microbiol 2006; 47:462-9; PMID: 16872384; http://dx.doi.org/10.1111/j.1574-695X.2006.00118.x
- Murphy KC, Campellone KG. Lambda Red-mediated recombinogenic engineering of enterohemorrhagic and enteropathogenic E. coli. BMC Mol Biol 2003; 4:11; PMID: 14672541; http://dx.doi.org/10.1186/1471-2199-4-11
- Mahmoud RY, Stones DH, Li W, Emara M, El-Domany RA, Wang D, Wang Y, Krachler AM, Yu J. The Multivalent Adhesion Molecule SSO1327 plays a key role in Shigella sonnei pathogenesis. Mol Microbiol 2016; 99:658-73; PMID: 26481305; http://dx.doi.org/10.1111/mmi.13255
- Harding CR, Schroeder GN, Collins JW, Frankel G. Use of Galleria mellonella as a model organism to study Legionella pneumophila infection. J Vis Exp 2013: 22:e50964; PMID: 24299965
- Harding CR, Stoneham CA, Schuelein R, Newton H, Oates CV, Hartland EL, Schroeder GN, Frankel G. The Dot/Icm effector SdhA is necessary for virulence of Legionella pneumophila in Galleria mellonella and A/J mice. Infect Immun 2013; 81:2598-605; PMID: 23649096; http://dx.doi.org/10.1128/IAI.00296-13
- Harding CR, Schroeder GN, Reynolds S, Kosta A, Collins JW, Mousnier A, Frankel G. Legionella pneumophila pathogenesis in the Galleria mellonella infection model. Infect Immun 2012; 80:2780-90; PMID: 22645286; http://dx.doi.org/10.1128/IAI.00510-12
- Kavanagh K, Reeves EP. Exploiting the potential of insects for in vivo pathogenicity testing of microbial pathogens. FEMS Microbiol Rev. 2004;28:101-12; PMID: 14975532; http://dx.doi.org/10.1016/j.femsre.2003.09.002
- Correa W, Manrique-Moreno M, Behrends J, Patino E, Marella C, Pelaez-Jaramillo C, Garidel P, Gutsmann T, Brandenburg K, Heinbockel L. Galleria mellonella native and analogue peptides Gm1 and DeltaGm1. II) anti-bacterial and anti-endotoxic effects. Biochim Biophys Acta 2014; 1838:2739-44; http://dx.doi.org/10.1016/j.bbamem.2014.07.005
- Wojda I, Taszlow P. Heat shock affects host-pathogen interaction in Galleria mellonella infected with Bacillus thuringiensis. J Insect Physiol 2013; 59:894-905; PMID: 23834825; http://dx.doi.org/10.1016/j.jinsphys.2013.06.011
- Trevijano-Contador N, Herrero-Fernandez I, Garcia-Barbazan I, Scorzoni L, Rueda C, Rossi SA, García-Rodas R, Zaragoza O. Cryptococcus neoformans induces antimicrobial responses and behaves as a facultative intracellular pathogen in the non mammalian model Galleria mellonella. Virulence 2015; 6:66-74; PMID: 25531532; http://dx.doi.org/10.4161/21505594.2014.986412
- Bergin D, Murphy L, Keenan J, Clynes M, Kavanagh K. Pre-exposure to yeast protects larvae of Galleria mellonella from a subsequent lethal infection by Candida albicans and is mediated by the increased expression of antimicrobial peptides. Microbes Infect 2006; 8:2105-12; PMID: 16782387; http://dx.doi.org/10.1016/j.micinf.2006.03.005
- Andrejko M, Mizerska-Dudka M, Jakubowicz T. Antibacterial activity in vivo and in vitro in the hemolymph of Galleria mellonella infected with Pseudomonas aeruginosa. Comp Biochem Physiol B Biochem Mol Biol 2009;152:118-23; PMID: 18996217; http://dx.doi.org/10.1016/j.cbpb.2008.10.008
- Sowa-Jasilek A, Zdybicka-Barabas A, Staczek S, Wydrych J, Mak P, Jakubowicz T, Cytryńska M. Studies on the role of insect hemolymph polypeptides: Galleria mellonella anionic peptide 2 and lysozyme. Peptides 2014; 53:194-201; PMID: 24472857; http://dx.doi.org/10.1016/j.peptides.2014.01.012
- Lapointe JF, Dunphy GB, Mandato CA. Haemocyte-haemocyte adhesion and nodulation reactions of the greater wax moth, Galleria mellonella are influenced by cholera toxin and its B-subunit. Results Immunol 2012; 2:54-65; PMID: 24371567; http://dx.doi.org/10.1016/j.rinim.2012.02.002
- Bender JK, Wille T, Blank K, Lange A, Gerlach RG. LPS structure and PhoQ activity are important for Salmonella Typhimurium virulence in the Galleria mellonella infection model [corrected]. PLoS One 2013; 8:e73287; PMID: 23951347; http://dx.doi.org/10.1371/journal.pone.0073287
- Banville N, Fallon J, McLoughlin K, Kavanagh K. Disruption of haemocyte function by exposure to cytochalasin b or nocodazole increases the susceptibility of Galleria mellonella larvae to infection. Microbes Infect 2011; 13:1191-8; PMID: 21782965; http://dx.doi.org/10.1016/j.micinf.2011.07.001
- Mukherjee K, Altincicek B, Hain T, Domann E, Vilcinskas A, Chakraborty T. Galleria mellonella as a model system for studying Listeria pathogenesis. Appl Environ Microbiol 2010;76:310-7; PMID: 19897755; http://dx.doi.org/10.1128/AEM.01301-09
- Mak P, Zdybicka-Barabas A, Cytrynska M. A different repertoire of Galleria mellonella antimicrobial peptides in larvae challenged with bacteria and fungi. Dev Comp Immunol 2010; 34:1129-36; PMID: 20558200; http://dx.doi.org/10.1016/j.dci.2010.06.005
- Zdybicka-Barabas A, Staczek S, Mak P, Piersiak T, Skrzypiec K, Cytrynska M. The effect of Galleria mellonella apolipophorin III on yeasts and filamentous fungi. J Insect Physiol 2012; 58:164-77; PMID: 22100292; http://dx.doi.org/10.1016/j.jinsphys.2011.11.003
- Abi Khattar Z, Rejasse A, Destoumieux-Garzon D, Escoubas JM, Sanchis V, Lereclus D, Givaudan A, Kallassy M, Nielsen-Leroux C, Gaudriault S. The dlt operon of Bacillus cereus is required for resistance to cationic antimicrobial peptides and for virulence in insects. J Bacteriol 2009; 191:7063-73; PMID: 19767427; http://dx.doi.org/10.1128/JB.00892-09
- Vogel H, Altincicek B, Glockner G, Vilcinskas A. A comprehensive transcriptome and immune-gene repertoire of the lepidopteran model host Galleria mellonella. BMC Genomics 2011; 12:308; PMID: 21663692; http://dx.doi.org/10.1186/1471-2164-12-308
- Arvanitis M, Fuchs BB, Mylonakis E. Nonmammalian model systems to investigate fungal biofilms. Methods Mol Biol 2014; 1147:159-72; PMID: 24664832
- Arvanitis M, Glavis-Bloom J, Mylonakis E. Invertebrate models of fungal infection. Biochim Biophys Acta 2013; 1832:1378-83; PMID: 23517918; http://dx.doi.org/10.1016/j.bbadis.2013.03.008
- Alenizi D, Ringwood T, Redhwan A, Bouraha B, Wren BW, Prentice M, McNally A. All Yersinia enterocolitica are pathogenic: virulence of phylogroup 1 Y. enterocolitica in a Galleria mellonella infection model. Microbiology 2016; 162:1379-87; PMID: 27221796; http://dx.doi.org/10.1099/mic.0.000311
- Champion OL, Cooper IA, James SL, Ford D, Karlyshev A, Wren BW, Duffield M, Oyston PC, Titball RW. Galleria mellonella as an alternative infection model for Yersinia pseudotuberculosis. Microbiology 2009;155:1516-22; PMID: 19383703; http://dx.doi.org/10.1099/mic.0.026823-0
- Giannouli M, Palatucci AT, Rubino V, Ruggiero G, Romano M, Triassi M, Ricci V, Zarrilli R. Use of larvae of the wax moth Galleria mellonella as an in vivo model to study the virulence of Helicobacter pylori. BMC Microbiol 2014; 14:228; PMID: 25170542; http://dx.doi.org/10.1186/s12866-014-0228-0
- Seitz V, Clermont A, Wedde M, Hummel M, Vilcinskas A, Schlatterer K, Podsiadlowski L. Identification of immunorelevant genes from greater wax moth (Galleria mellonella) by a subtractive hybridization approach. Dev Comp Immunol 2003; 27:207-15
- Schuhmann B, Seitz V, Vilcinskas A, Podsiadlowski L. Cloning and expression of gallerimycin, an antifungal peptide expressed in immune response of greater wax moth larvae, Galleria mellonella. Arch Insect Biochem Physiol 2003; 53:125-33; PMID: 12811766; http://dx.doi.org/10.1002/arch.10091
- Khoa DB, Takeda M. Expression analysis of inhibitor of apoptosis and related caspases in the midgut and silk gland of the greater wax moth, Galleria mellonella, during metamorphosis and under starvation. Gene 2012; 510:133-41; PMID: 22975212; http://dx.doi.org/10.1016/j.gene.2012.08.036
- Khoa DB, Takeda M. Expression of autophagy 8 (Atg8) and its role in the midgut and other organs of the greater wax moth, Galleria mellonella, during metamorphic remodelling and under starvation. Insect Mol Biol 2012; 21:473-87; PMID: 22830988; http://dx.doi.org/10.1111/j.1365-2583.2012.01152.x
- Semenova AD, Glazachev YI, Slepneva IA, Glupov VV. Quantitative determination of nitric oxide production in haemocytes: nitrite reduction activity as a potential pathway of NO formation in haemolymph of Galleria mellonella larvae. Nitric Oxide 2014; 37:46-52; PMID: 24406682; http://dx.doi.org/10.1016/j.niox.2013.12.011
- Khoa DB, Trang LT, Takeda M. Expression analyses of caspase-1 and related activities in the midgut of Galleria mellonella during metamorphosis. Insect Mol Biol 2012; 21:247-56; PMID: 22229544; http://dx.doi.org/10.1111/j.1365-2583.2011.01131.x
- Renwick J, Daly P, Reeves EP, Kavanagh K. Susceptibility of larvae of Galleria mellonella to infection by Aspergillus fumigatus is dependent upon stage of conidial germination. Mycopathologia 2006; 161:377-84; PMID: 16761185; http://dx.doi.org/10.1007/s11046-006-0021-1
- Kane KA, Dorman CJ. VirB-mediated positive feedback control of the virulence gene regulatory cascade of Shigella flexneri. J Bacteriol 2012; 194:5264-73; PMID: 22821978; http://dx.doi.org/10.1128/JB.00800-12
- Mowlds P, Kavanagh K. Effect of pre-incubation temperature on susceptibility of Galleria mellonella larvae to infection by Candida albicans. Mycopathologia 2008;165:5-12; PMID: 17922218; http://dx.doi.org/10.1007/s11046-007-9069-9
- Taszlow P, Wojda I. Changes in the hemolymph protein profiles in Galleria mellonella infected with Bacillus thuringiensis involve apolipophorin III. The effect of heat shock. Arch Insect Biochem Physiol 2015; 88:123-43; PMID: 25308190; http://dx.doi.org/10.1002/arch.21208
- Vertyporokh L, Taszlow P, Samorek-Pierog M, Wojda I. Short-term heat shock affects the course of immune response in Galleria mellonella naturally infected with the entomopathogenic fungus Beauveria bassiana. J Invertebr Pathol 2015; 130:42-51; PMID: 26149823; http://dx.doi.org/10.1016/j.jip.2015.07.001
- Mowlds P, Barron A, Kavanagh K. Physical stress primes the immune response of Galleria mellonella larvae to infection by Candida albicans. Microbes Infect 2008; 10:628-34; PMID: 18457977; http://dx.doi.org/10.1016/j.micinf.2008.02.011
- Banville N, Browne N, Kavanagh K. Effect of nutrient deprivation on the susceptibility of Galleria mellonella larvae to infection. Virulence 2012; 3:497-503; PMID: 23076277; http://dx.doi.org/10.4161/viru.21972