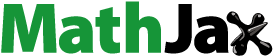
ABSTRACT
Fermented foods and beverages are a significant source of dietary bacteria that enter the gastrointestinal (GI) tract. However, little is known about how these microbes survive and adapt to the small intestinal environment. Colony-forming units (CFU) enumeration and viability qPCR of Lacticaseibacillus rhamnosus CNCM I-3690 in the ileal effluent of 10 ileostomy subjects during 12-h post consumption of a dairy product fermented with this strain demonstrated the high level of survival of this strain during human small intestine passage. Metatranscriptome analyses revealed the in situ transcriptome of L. rhamnosus in the small intestine, which was contrasted with transcriptome data obtained from in vitro cultivation. These comparative analyses revealed substantial metabolic adaptations of L. rhamnosus during small intestine transit, including adjustments of carbohydrate metabolism, surface-protein expression, and translation machinery. The prominent presence of L. rhamnosus in the effluent samples did not elicit an appreciable effect on the composition of the endogenous small intestine microbiome, but significantly altered the ecosystem’s overall activity profile, particularly of pathways associated with carbohydrate metabolism. Strikingly, two of the previously recognized gut-brain metabolic modules expressed in situ by L. rhamnosus (inositol degradation and glutamate synthesis II) are among the most dominantly enriched activities in the ecosystem’s activity profile. This study establishes the survival capacity of L. rhamnosus in the human small intestine and highlights its functional adjustment in situ, which we postulate to play a role in the probiotic effects associated with this strain.
Introduction
The Food and Agriculture Organization (FAO) and World Health Organization (WHO) define probiotics as live organisms that, when consumed in sufficient amounts, confer a health benefit to the host.Citation1 Most of the currently marketed probiotics belong to the bacterial families of the Bifidobacteriaceae or Lactobacilaceae,Citation2 which are commonly applied in fermented food products. They also inhabit various environments, including the human body.Citation3 Among the Lactobacillaceae, various probiotic strains of the species Lacticaseibacillus rhamnosus have been described and were shown in multiple studies to elicit beneficial effects on the host. In particular, L. rhamnosus was associated with relieving symptoms caused by intestinal microbiota dysbiosis, disruption of intestinal homeostasis, and amelioration of alcohol-induced liver injury.Citation4–6 Among the L. rhamnosus strains that displayed several probiotic qualities, L. rhgamnosus CNCM I-3690 was shown in preclinical studies to restore impaired intestinal barrier functions via its anti-inflammatory effectCitation7,Citation8 and tight junction modulationCitation9 to protect against oxidative stress in a colitis model,Citation10 and limit some of the immune and metabolic impairments caused by the pathobiont Bilophila wadsworthia.Citation11 Additionally, administration of L. rhamnosus CNCM I-3690 in a murine model of diet-induced obesity was shown to limit weight gain, improve glucose-insulin homeostasis, and reduce hepatic steatosis.Citation12 Finally, in a clinical study that explored the effects of this strain on the gut-brain axis it was reported to significantly reduce subjective stress levels in healthy adults in an academic stress model.Citation13 These studies underline the broad range of health benefits associated with the consumption of this candidate probiotic strain L. rhamnosus CNCM I-3690, warranting further research into its survival and activity during intestinal tract passage.
Passage of the human gastrointestinal tract constitutes a dynamic and challenging environment for ingested bacteria (e.g., probiotics), which may get harmed due to their exposure to gastric juice, bile salts, and host- or microbiome-derived antimicrobial or antagonistic interactions. Nevertheless, various studies have demonstrated the effective survival of probiotic Lactobacillaceae after human intestine transit, mostly employing enumeration of probiotic bacteria in stool samples but also exemplifying their low relative abundances (<1%) in feces (for a review, seeCitation14). Contrary to this, studies that evaluated the survival and abundance of probiotic bacteria in the small intestine (SI) concluded that these bacteria (transiently) constitute a prominent fraction of the SI microbial ecosystem.Citation14 Analogously, we have demonstrated that L. rhamnosus CNCM I-3690 can transiently inhabit the human SI at a relative abundance of 10–75% in some participants but substantially lower abundance in others. This high versus low colonization efficacy of the ingested L. rhamnosus CNCM I-3690 per participant was associated with predominance of carbon- versus amino acid-derived energy metabolism in the endogenous SI microbiome, where the latter situation was reflected by the high relative abundance of the Peptostreptococcaceae in the subject’s SI.Citation15 Thereby this study provided mechanistic insight in what was previously referred to as colonization-permissive versus -resistant microbiome communities.Citation15–17
With the prominent temporal presence of L. rhamnosus CNCM I-3690 in the small intestine, it may be anticipated that they significantly participate in the overall metabolic activity of the SI microbiome. By contrast, such participation would be substantially smaller in the large intestinal microbiome, where these probiotic bacteria constitute only a small fraction (<1%) of the overall community. Importantly, the SI is responsible for most of the nutrient digestion and absorption and is commonly estimated to be responsible for approximately 85–90% of the overall caloric intake in humans.Citation18 Rodent models have shown that the SI microbiota is involved in regulating a range of metabolic and physiological characteristics ranging from lipid digestion and adsorption,Citation19 whole-body glucose homeostasis,Citation20 bile acid metabolism and cycling,Citation21 as well as contributing to the bioavailability of several important micronutrients like vitamin K and B12Citation22,Citation23 and the circadian rhythm of immune homeostasis.Citation24 Moreover, the health-importance of the SI microbiome has been highlighted by the association of SI microbial dysbiosis with the pathogenesis of functional gastrointestinal disorders,Citation25 small intestinal bacterial overgrowth,Citation26 environmental enteric dysfunction,Citation27 and other pathologies.Citation28,Citation29 Overall, these studies support the need for a deeper understanding of the diet-microbe-host interactions in the SI region, where transient high-level presence and activity of probiotic strains may have an important impact.
The SI is a particularly harsh environment for bacterial life due to its short transit time, high concentration of digestive enzymes and bile salts, and antimicrobial peptides produced by Paneth cells. Consequently, the SI microbial community has a substantially lower density and diversity than the large intestinal community,Citation30 ranging from approximately 10Citation4,Citation5 to 10Citation7–9 bacteria per mL in the duodenum and distal ileum, respectively.Citation31 However, in contrast to the colon, the SI microbiome remains relatively poorly characterized due to the invasive technologies required for sampling this segment of the digestive tract. Recent developments in minimally invasive sampling capsules for small intestine microbiome and metabolome analyses may solve these sampling problems in the future.Citation32–34 As an alternative, subjects that underwent surgical colon removal (total colectomy) as a remedy for intestinal diseases like colorectal cancer or inflammatory bowel diseases (ulcerative colitis or Crohn’s) have been used to obtain samples from the small intestinal lumen. In a subgroup of these subjects, the ileum is connected to a stoma in their abdominal wall (ileostomists), allowing noninvasive and repeated collection of effluent of the SI tract.Citation30,Citation35,Citation36 Despite this history of intestinal disease, the SI of these individuals is considered to function similarly to the SI tract of a normal individual,Citation37 in the absence of comorbidities and need for medication. The normal functioning of the small intestine in these ileostomists is reflected by the significant similarity between the microbiota in the effluent samples and samples obtained from the proximal (jejunum, ileum) regions of the SI in healthy volunteers.Citation30 These effluent studies also revealed an individual-specific and highly dynamic ecosystem composition in the SI.Citation15 Despite its compositional variation (between individuals and over time), the SI microbiome is commonly dominated by fast-growing facultative anaerobe bacteria that thrive in a habitat characterized by a high nutrient availability and composition fluctuation.Citation30 This nutrient adaptability of the SI microbiome is reflected by its enrichment for genes related to acquisition (import) and utilization of simple carbohydrate substrates, rather than pathways associated with complex carbohydrates degradation that are enriched in the colonic microbiome.Citation15,Citation30 Additionally, metatranscriptome analyses in ileostoma effluent samples established high-level expression of sugar phosphotransferase systems, pentose phosphate-, lactate-, acetate-, and propionate-fermentation pathways by some of the most commonly found genera, Streptococcus, Veillonella, Staphylococcus, Escherichia, Clostridium and Bacteroides.Citation30,Citation35,Citation38 Although several studies have investigated the survival of ingested (probiotic) bacteria during SI transit,Citation39–41 little is known about their specific activity or their impact on the overall microbiome activity profile in this intestinal region.
In the present study, 10 ileostomists consumed a dairy product fermented by L. rhamnosus CNCM I-3690 as part of a standardized breakfast. Following consumption, ileostoma effluent was collected for 12 h to assess the effects of product consumption on the SI microbiota composition, but also to determine the population size and viability of L. rhamnosus CNCM I-3690. In addition, ileostoma effluent-metatranscriptomics was employed to identify the in situ L. rhamnosus CNCM I-3690 transcriptional adaptation, by comparative transcription analysis using various in vitro cultivation conditions. Finally, the relative contribution of the L. rhamnosus CNCM I-3690 transcriptome to the SI microbiome metatranscriptome highlighted its contribution to metabolic modules associated with carbohydrate utilizationCitation42 and those proposed to participate in modulation of the gut-brain axis.Citation43
Results
Study design and assessment of L. rhamnosus survival to the gastrointestinal passage
To evaluate the ability of L. rhamnosus to reach the upper gastrointestinal tract in a viable state and survive the passage of the small intestine, ileal effluent samples from 10 subjects were collected prior (0 H) and collectively in 4-h time intervals during an overall 12-h period (0–4, 4–8, and 8–12) after consumption of L. rhamnosus fermented product (total bacterial intake ~ 1011-5×1011 CFU) as part of a standardized breakfast. After collection and homogenization, sample aliquots were prepared for downstream analyses: metataxonomics, live-dead staining followed by V-PCR and serial dilution and plating in selective media. Importantly, L. rhamnosus was not detectable at baseline (no colonies detected on vancomycin-MRS plates, data not shown) and reached its recovery peak after 8 h of ingestion (). The total amount of L. rhamnosus CNCM I-3690 recovered during the 12-h sampling period of ileal effluent varied between subjects (, panel b, max total recovery VOL13: 1.2 × 1011 CFU, min recovery VOL11: 3.62 × 108 CFU). Approximately 5 × 1010 CFU were recovered per subject, corresponding to approximately 30% of the number of ingested bacteria. A notable degree of fluctuation was observed in the ileal effluent volumes obtained at the different time points in the different volunteers, which impacted on the estimated L. rhamnosus concentration per volume (, panel b) that on average was 2.1 × 108 CFU/ml but ranged from 1.1 × 109 CFU/ml (max; VOL03-8 H) to 4.4 × 104 CFU/ml (min; VOL11-8 H).
Figure 1. L. rhamnosus CNCM I-3690 recovery and concentration in ileal effluent. Samples were collected prior and collectively obtained during 4-h periods over a total timespan of 12 h(4, 8, and 12) after consuming L. rhamnosus CNCM I-3690 fermented product. Serial dilutions were plated in triplicate and L. rhamnosus CNCM I-3690 colonies were enumerated and identity confirmed after 4 (black dots), 8 (magenta dots), and 12 (green dots) hours, while the total amount recovered per volunteer is indicated with a blue asterisk. L. rhamnosus CNCM I-3690 recovery peaked after 8 h in most volunteers (Panel , while it was not detected before consumption (data not shown). Ileostomy effluent volumes fluctuated considerably, affecting the L. rhamnosus concentration per sample (Panel b), averaging approximately 2*108 CFU/mL.

We assessed relative viability of the total L. rhamnosus population by V-PCR, able to distinguish between live and dead bacteria (see Materials and Method), revealing that on average, 82% of L. rhamnosus population was in a viable state (), underlining the high survival capacity of L. rhamnosus during gastrointestinal passage. The resulting viable count estimates obtained by qPCR tended to be lower than those obtained by CFU enumerations (average accuracy of 55%), which is in agreement with previous studies that showed similar discrepancies between the two techniques.Citation44 These analyses indicate that a relatively high proportion of ingested L. rhamnosus CNCM I-3690 reaches the end of the SI in a viable form within 12 h from ingestion, although considerable variations in estimated survival rates and SI passage kinetics are seen between individuals (Max VOL13: 72%, Min VOL11: 0.2%, Average: 30%).
Figure 2. The small intestinal environment inducesMicrobiota composition analysis and L. rhamnosus recovery. a) L. rhamnosus CNCM I-3690 gene copies recovery in ileal effluent, assessed via qPCR using both PMAxx treated (black bars) and non-treated samples (pink-bars). Samples were collected prior and collectively obtained during 4-h periods over a total timespan of 12 h (4, 8, and 12) after consuming L. rhamnosus CNCM I-3690 fermented product and prior of it (0 H); b) overview of the microbiota composition throughout the study per volunteer at genus level, only the top 25 genera are displayed (comprising >95% of the total community). The genus formerly known as Lactobacillus is colored in red.

Analysis of L. rhamnosus fermented product consumption on SI microbial composition
To assess the effect of L. rhamnosus on the small intestinal microbiota, the microbial composition was evaluated via 16S rDNA sequencing. The present observations confirm our previously reported conclusions small intestinal microbiota composition and the impact of L. rhamnosus ingestion is highly individual-specific.Citation15 The subject ID clearly illustrated this individuality explaining approximately 50% of the total data variation at species level (Supplementary Figure S1) and substantial variability of the L. rhamnosus relative abundance in individual effluent samples. Moreover, there is a strikingly high degree of fluctuation in the microbial community composition during the 12 h in which samples were collected ().
The consumption of the L. rhamnosus CNCM I-3690 fermented product did not elicit a significant modification of the endogenous microbiota beyond the appearance of the ingested bacterial strain in variable relative abundance, nor did it significantly impact the α or β diversity of the endogenous microbial community (data not shown). These observations underline the dynamic nature of the SI microbiome, and that the short-term impact of L. rhamnosus fermented milk consumption on the SI microbiota is negligible, which we also observed upon prolonged consumption of the same fermented product (2 weeks, daily).Citation15
Transcriptome analysis of L. rhamnosus recovered from small intestinal effluent
To evaluate the in situ transcriptional activity of L. rhamnosus CNCM I-3690, we employed previously determined metatranscriptome dataCitation15. These datasets were mined for the L. rhamnosus small intestinal (in situ) transcription and compared to L. rhamnosus CNCM I-3690 transcriptome profiles obtained for 5 in vitro growth conditions, including MRS broth without supplementation of a carbon source (MRS-C), supplemented with glucose (MRS+G), lactose (MRS+L), or mannose (MRS+M), and milk. Principal component analysis (PCA) showed separate clustering of the in situ samples relative to the MRS samples, which were also distinct from the samples obtained from L. rhamnosus grown in milk (). Comparison of the Bray-Curtis dissimilarities within the same ‘sample group’ showed that the in situ samples have a significantly higher degree of variability (Supplementary figure S2), which we interpret to reflect the individuality of the niche and ecosystem of the small intestinal tract.
Figure 3. Transcriptome analysis of L. rhamnosus recovered from small intestinal effluent compared with in vitro conditions. a) Principal component analysis of L. rhamnosus transcriptome samples; separating the in situ samples from the MRS samples that also separate from the milk grown samples (green dots). b) volcano plot of the differential expression analysis performed via edgeR, in blue and in red downregulated (blue) and upregulated (red) genes in situ compared to in vitro; cut-off: FDR adjusted-p-value≤0.05, Log2FC≥2. c) Gene Set Enrichment Analysis (GSEA) using the differentially expressed genes (enrichment cut-off, p-value < 0.05; fold enrichment is given as the ratio between “hits” and “expected hits” on the selected gene category) identifies 13 KEGG categories, of which 11 belong to “Metabolism” encompassing 8 belonging to “Carbohydrate metabolism” (green box). Bar colours represents an average gene expression per pathway higher (red) or lower (blue) in situ compared to in vitro condition; d) heatmap of the expression of enriched pathways belonging to the KEGG category “Metabolism”, for each pathway, the average gene expression per volunteer was used to calculate the fold change from the row mean; hierarchical clustering: complete linkage based on Euclidian distances. Among the overrepresented gene sets, only two (Fatty acid biosynthesis and Pyruvate metabolism) had a higher expression in vitro than in situ, while the remaining 9 were higher expressed in situ.

To investigate the gene expression of L. rhamnosus in situ in comparison to its transcription profile when grown under in vitro conditions, we performed differential expression analysis, using edgeR, identifying 1572 differentially expressed genes (FDR adjusted p-value ≤0.05), of which 390 are highly differentially expressed (Log2FC ≥2). Notably, these numbers of differentially expressed genes correspond to 53 and 13% of the total L. rhamnosus gene repertoire, respectively, which illustrates the major transcriptional adaptation of this bacterium during its transit through the intestinal tract. Finally, most of the highly differentially expressed genes, (295, ~75%; ) were expressed at a higher level in the in situ samples compared to the in vitro samples.
L. rhamnosus highly modulates carbohydrate metabolism in situ
The stringently selected differentially expressed genes () were used to perform Gene Set Enrichment Analysis (GSEA), resulting in the identification of 13 significantly enriched KEGG categories among the genes that were differentially expressed in the intestinal tract compared to the in vitro conditions, most of which were induced in the intestinal tract (). Notably, 11 of the induced categories belonged to the overarching category of “Metabolism,” of which 8 were categorized under “Carbohydrate metabolism,” but which also included the “Uridine monophosphate biosynthesis” pathway, and the “Thiamine metabolism” (Supplementary Table S1).
Conversely, we found that genes involved in the “Fatty acid biosynthesis” and “pyruvate metabolism” were higher expressed (, Supplementary table S1) in vitro relative to in situ in the intestine. A similar pattern of in vitro induction of “pyruvate metabolism” associated genes was observed. Interestingly, L. rhamnosus growth in MRS that lacks a supplemented carbon source free, as well as the MRS supplemented with mannose did not induce these categories of genes (). This could relate to the lower bacterial growth rate under the latter two in vitro conditions, which could imply that growth rates in most of the intestinal tract samples is also lower as compared to the glucose or lactose supplemented MRS and milk grown in vitro samples. It is important to notice that, the expression of carbon and other nutrient acquisition functions was in many cases activated during intestinal transit in a highly individual-dependent manner, probably reflecting different diets and consequently different SI environments per individual, which probably underlies the observed variability of in situ gene expression (, Supplementary Figure S2). This is confirmed by the high coefficient of variation observed for the in situ expression values of specific genes related to nutrient acquisition and metabolism, such as (ABC) transporters (, I) and PTS systems (, II). For example, 9 out of the 18 PTS encoded by L. rhamnosus (, II) were, on average, expressed at a higher level in situ compared to in vitro conditions (predicted substrates include sucrose, β-glucoside, mannitol, cellobiose/diacerylchitobiose, mannose, sorbitol, galactitol, and ascorbate), but variable expression among the in situ samples is likely reflecting the differential availability of these substrates in an individuals’ SI environment (, I). Intriguingly, the global regulator involved in the regulation of many of the carbohydrate acquisition genes, Catabolite Control Protein A (ccpA, ) was significantly higher expressed in the in situ samples compared to the in vitro samples, albeit with substantial fluctuation of expression in the in situ samples that could reflect overall carbon source availability variations in situ.
Figure 4. L. rhamnosus response to the small intestinal environment. Panel a: The expression values are displayed as edgeR derived, FDR < 0.05, logFC ≥ 2, displayed as a heat map representing fold change relative to the mean expression per row; blue, and red intensities reflect relative down- and up-regulation, white reflects mean-level expression. The variable environmental conditions encountered by L. rhamnosus in each individual is reflected by diverse in situ gene expression of nutrient acquisition genes (e.g., sugar import functions, panel a, parts I and II). Conversely, a ribosomal protein gene cluster is consistently induced in situ, while a few ribosomal protein transcripts are induced in MRS-cultures (panel a, part III), suggesting adjustment of translation machinery associated functions in these contrasting conditions.
The degree of variation of in situ gene expression is illustrated by the coefficient of variation color scale at the left end of the heatmaps (white, red). The adjacent color scale (green, white, violet) indicates the average Log2FC in intestinal samples compared to in vitro samples. At the left end of the heatmap display a per category hierarchical clustering is displayed using linkage based on Euclidian distances.
Panel b: expression of the global regulator Catabolite Control Protein A gene (ccpA) is induced in situ relative to in vitro, but variable in in the intestinal samples (panels aI and aII). Panel c: variable in situ induction of the pilus encoding operon (spaD-F), which plays a role in adhesion and immune-related effects of L. rhamnosus. Significance of the differences in expression was assessed by FDR adjusted p-value <0.05; ****: FDR adjusted p-value <0.0001.

The sample variability of the metabolism associated functions observed in the intestinal samples is sharply contrasted by the highly consistent and homogeneous in situ induction of a subset of ribosomal protein encoding genes that are encoded in a gene cluster (rpsK, rpsM, rplO, rpmD, rpsE, rplR, rplF, rpsH, rplE, rplX, rplN, rpsQ, rpmC, rplP, rpsC, rplV, rpsS, and rplD; , III). Conversely, three other ribosomal protein encoding genes (rpsT, rpmF and rpmE2) were consistently expressed at a higher level in the MRS grown cultures than in situ samples. These observations suggest that L. rhamnosus CNCM I-3690 consistently adjusts its translation machinery in these two distinct conditions, but not during growth in milk (, III).
The small intestinal environment induces L. rhamnosus surface-protein alterations
Surface-associated and extracellular proteins play an important role in the interactions with, and adaptations to the environment in bacteria. Therefore, SignalP 5.0Citation45 was employed to predict the complete secretome and surface-exposed proteome of L. rhamnosus, identifying 120 exported proteins. Strikingly, no less than 70 genes encoding these exported proteins were significantly differentially expressed when comparing the in situ (intestinal tract) and in vitro samples. Of these 70 genes, 41 and 29 were up- and down-regulated in situ, respectively. Several of these proteins are components of transport systems or PTS, but there were also many proteins of poorly defined function. Among the in situ upregulated genes many contained specific domains suggesting that they were involved in bacterial adhesion (Supplementary figure S3), i.e., fibronectin-binding, peptidoglycan-binding (LysM), sugar-moiety binding lectin, and two mucin binding (Mub B2 and MucBP) serine-rich adhesins. In addition, several proteins belonging to the sortase anchored cell-wall bound proteins based on the presence of the canonical LPXTG-motif in their protein sequence were similar to proteins that were reported to be important in adhesion to host cells and biofilm formation.Citation46,Citation47 Also, the genes encoding components of the L. rhamnosus pilus (spaD-F, ) were upregulated in situ. These proteins form a surface bound multiprotein complex that forms an appendage on the cell-surface that has been shown to play a pivotal role in L. rhamnosus adhesion as well as immune-modulation.Citation8 Taken together, the secretome gene expression was on average, higher in situ than in vitro, but was also quite variable between subjects and timepoints, analogous to what was concluded for the nutrient acquisition systems.
L. rhamnosus metabolic contribution to the overall ecosystem
Following the L. rhamnosus in situ transcriptome analysis, we wanted to evaluate the ecosystem contribution of this species by examining its relative contribution to the overall activity of the same functional category within the SI ecosystem. To this end, we calculated the “contribution enrichment factor” (see Materials and Methods for details) as a proxy for the contribution to pathway activity by L. rhamnosus focusing on the enriched KEGG categories described above (). This analysis revealed that while most of the KEGG category activity levels only have a small contribution L. rhamnosus compared to the rest of the microbial community, the carbohydrate metabolism category displayed a significant overrepresentation of L. rhamnosus transcriptome activity relative to the overall community (). The contribution enrichment factors for “Carbohydrate metabolism” sub-pathways, revealed that activity contribution by L. rhamnosus was particularly pronounced for “Galactose metabolism”, “Fructose and mannose metabolism”, and “Inositol phosphate metabolism”. Conversely, the L. rhamnosus contributions to the ecosystem activity level of “Citrate cycle (TCA cycle)”, “C5-Branched dibasic acid metabolism”, and “Glyoxylate and dicarboxylate metabolism” () were virtually negligible. Among the most prominent L. rhamnosus contributions to the ecosystem activity, the “Inositol phosphate metabolism” is of particular interest since it includes one of the Gut-Brain Modules (GBM) that were previously proposed to potentially influence the human neuronal systemCitation43. Nine of these GBMs are encoded by L. rhamnosus (Supplementary Table S1), and two are significantly more expressed by the ingested bacteria than by the small intestinal endogenous microbiota, the “Inositol degradation” and “Glutamate biosynthesis II” modules. Moreover, the “Inositol degradation” module is among the highest contribution enrichment factors among the GBMs encoded by the L. rhamnosus genome, indicating that L. rhamnosus prominently contributes to the overall activity to this specific pathway (Supplementary Table S1, 2), which is in agreement with the observation that L. rhamnosus is responsible for most of the Inositol degradation expression in the small intestinal metatranscriptome (, mean 85.6% ± 7.9%). Moreover, most of the 13-gene inositol degradation operon of L. rhamnosus (LRHA10_v2_0256–0268) was strongly upregulated in situ relative to in vitro conditions (Supplementary Figure S4a, b, Figure S5e). While the Inositol degradation module is considered to be relatively rare in reference genomes of intestinal bacteria, with a prevalence of less than 10%, the Glutamate biosynthesis II module is more commonly found in the gut microbiota, being present in 76% of the bacterial population.Citation43
Figure 5. Ecosystem contribution by L. rhamnosus on the overall activity profile of the microbiota. The “contribution enrichment factor”, calculated as log2 of the fraction of all L. rhamnosus reads mapped to a category divided by the fraction of all other reads mapped to that category, was assessed for the main KEGG metabolism classes (panel a) and for the carbohydrate metabolism major categories (panel b). The analyses showed that L. rhamnosus dedicates more of its transcriptome compared with the endogenous microbiota to “Carbohydrate metabolism”, “Galactose metabolism”, “Fructose and mannose metabolism”, and “Inositol phosphate metabolism” (Wilcoxon tests, Holm-Sidak Post hoc test * p-value < 0.05, ** p-value < 0.01, ***p-value < 0.005).
The “Inositol phosphate metabolism” and the “Glutamate biosynthesis II” are identified as two of the nine Gut-Brain Modules encoded by L. rhamnosus genome and they are significantly more expressed by L. rhamnosus than by the small intestinal endogenous microbiota (c, Mann–Whitney test, Holm-Sidak Post hoc test ***p-value <0.005, **** p-value <0.001). L. rhamnosus is responsible for most of the Inositol degradation expression (d, red represent in L. rhamnosus contribution, in blue the endogenous one) and that most of the 13-genes-long operon (e), encoding the entire Inositol degradation pathway, is highly expressed in situ compared with in vitro conditions.

Discussion
The definition of probiotics by the FAO and WHO -live organisms that confer a health benefit to the host when consumed in sufficient amountCitation1− leaves the viability and activity of the probiotic during gastrointestinal transit uncharacterized. However, what happens after ingestion, including the bacterial viability, adaptation, and response to the new environment is crucial to understand host-microbes interaction and their possible physiological effects on the host.
Here, we demonstrate that L. rhamnosus CNCM I-3690 can effectively survive the passage of the human small intestine, and adjusts its gene expression based on the new environmental condition. We were able to consistently detect the strain in the ileostoma effluent for up to 12 h post-ingestion, with a peak in bacterial density observed in the 4–8-h interval and a high viability percentage within the L. rhamnosus population (average ~82%). Approximately 30% of the consumed bacteria were recovered from the effluent, indicating the metabolic contributions of the strain to the small intestinal ecosystem for at least 12 h. These findings align with existing literature that emphasized the robust survival capabilities within the upper gastrointestinal tract of members of the Lactobacillaceae family and that their ingestion does not induce significant changes to the endogenous SI microbiota, beyond the introduced strain, in high but variable relative abundance.Citation15,Citation40 The data collected in this study allowed us to estimate the community density during the 12 h of sample collection, which revealed a strikingly high degree of fluctuation. We noted considerable fluctuations in the community composition and density during sample collection, which reflect the microbial ecosystem’s response to nutrient availability and transit rate through the SI system, which plausibly affect transient selective pressure in the ecosystem as well as determine the growth rate and absolute abundance of its members. The dynamic nature of the human small intestine microbiota, following internal and external perturbations, has been reported in other studies,Citation48,Citation49 although never addressing such short timespan fluctuations as described in this study, supporting the importance of carbohydrate availability as a determinant in rapidly shaping the small intestinal ecosystem.Citation50
Recently, minimally invasive sampling capsules were successfully tested for the small intestine microbiome and metabolome analysis in human subjects.Citation32–34 These advancements can revolutionize gut microbiota research, by expanding the scope to the small intestine. However, although the capsules were enabling metabolite and microbiome composition analyses, there are still substantial challenges to overcome like adequate quenching procedures to stagnate further bacterial, growth, activity changes, and metabolic conversions once the sample is internalized into the sampling capsule because the capsule remains at body temperature for hours afterward, while transiting through the remainder of the gut. Particularly metatranscriptome measurements will most likely be unreliable in such samples, due to the short life-time of the average microbial mRNA as well as the very minute sample size that can be collected with these devices. This limited sample size, while capable of revealing valuable data, also raises concerns about representativeness, given the immense diversity and variability of the gut microbiota across different intestinal segments. Deploying multiple capsules could mitigate the latter issue and improve the samples’ representativeness. Although capsule sampling might not completely replace techniques aiming at obtaining larger sample volume sampling (e.g., ileostomy effluent) for comprehensive studies, it marks a significant advancement in gut microbiota investigations, which with further technical improvements may provide possibilities beyond microbiome composition and metabolism in the future.
The in situ transcriptome analysis revealed that more than half of all genes encoded by L. rhamnosus CNCM I-3690 were differentially expressed, primarily induced in situ, indicating that this strain is active during SI passage and drastically adjusts its gene expression profile in comparison to in vitro culturing conditions. Environmental conditions, particularly nutrient and carbon source availability, play a key role in modulating L. rhamnosus transcriptome. This is evidenced by the enrichment of differentially expressed genes related to carbohydrate metabolism and nutrient acquisition, which are crucial for bacterial survival during GI tract passage.Citation51,Citation52 Other lactobacilli, like L. rhamnosus, adapt their carbohydrate acquisition and surface protein expression to thrive in the variable dietary conditions in the human intestine.Citation53–55 Carbon source availability may be highly diverse and dynamic in the small intestine due to variable dietary intake. This sharply contrasts the nutrient-rich environment and single carbon source provided in the laboratory media that were used in this study. The diversity of the small intestine environment is evidenced by varying expression of carbohydrate metabolism-associated genes across different individuals and timepoints. Importantly, the in situ expression of the global regulatory function CcpA is induced, signifying its role in regulating bacterial adaptation to these changing environmental conditions. CcpA controls the transcription of many genes involved in carbohydrate metabolism, growth, stress response, and metabolite production in many gram-positive bacteriaCitation56 and is a pivotal regulator in bacterial adaptation to environmental changesCitation57,Citation58 that orchestrates transitions between environmental (nutrient) specialization (e.g., in stable and constant laboratory conditions) and environmental (nutrient) generalization (e.g., in dynamic conditions like the intestinal tract). Notably, PTS systems and Pyrimidine metabolism, both highly expressed in situ, were reportedly involved in acid stress tolerance that links CcpA regulation to generic environmental fitness and stress tolerance.Citation59
We observed an opposite expression pattern for most of the fatty acid biosynthesis pathways (fabF-Z, accA-D, and mleA) involved in controlling the fatty acid composition of the bacterial membrane to maintain its stability and normal physiological function, and its expression is downregulated in media containing high amounts of saturated fatty acids.Citation60,Citation61 This suggests that L. rhamnosus utilizes fatty acids that are available in the lumen of the small intestine, which is the main region of the intestinal tract for fat degradation and absorption, aligning with previous reports on Lactobacillus’s incfluence on fat absorption.Citation62
The remarkable adaptability of L. rhamnosus to the SI environment is exemplified by the consistent in situ induction of one of the major ribosomal gene clusters, potentially regulated by the multi-functional ribosomal protein L4 RplD, which could play a critical role in the adaptation to the SI environment.Citation63,Citation64 Apart from protein biosynthesis, ribosomal proteins, especially the L4 protein, also contribute to DNA repair, cell death, transcription regulation, and environmental sensing, and was proposed to contribute to bacterial environmental adaptation by modulating mRNA composition under stress conditions through RNA degradation.Citation63,Citation65
L. rhamnosus CNCM I-3690 has been associated with various probiotic activities, including the ability to prevent and/or hamper dysfunctions related to the gut barrier. One of the bacterial functions responsible for this ability has been proposed to be the L. rhamnosus CNCM I-3690 pilus (spaD-F),Citation8 a cell-associated extracellular proteinaceous appendage, homologues of which have been shown to influence bacterial adhesion and immune-modulation in various probiotic and commensal bacteria.Citation66,Citation67 The expression of the spaD-F was significantly induced in situ, demonstrating that this important host–microbe interaction function of L. rhamnosus CNCM I-3690 is actively expressed in the small intestine, albeit with considerably fluctuating levels of expression in different individuals. The in situ environment also influences the expression of other bacterial functions, such as surface and extracellular proteins, that are considered pivotal for host interaction and gut persistence. Specifically, mucus and extracellular matrix interaction genes are induced in situ, potentially influencing adhesion and biofilm formation.Citation46,Citation68,Citation69 L. rhamnosus CNCM I-3690 consumption significantly contributed to the ecosystem activity profile, especially on simple carbohydrate utilization pathways, including those for galactose, fructose and mannose. These pathways are relatively common among bacteria, and this finding is probably indicative of the competitive capacity of L. rhamnosus to acquire these substrates for growth.
An intriguingly high enrichment factor was found for the “inositol phosphate metabolism” pathway, where in many samples the majority of this activity in the ecosystem could be assigned to L. rhamnosus CNCM I-3690. Inositol, a widely present sugar especially in its Myo-inositol form, is critical for health, with aberrations linked to various metabolic imbalances and neurological disorders.Citation70,Citation71 In addition, inositol also is an important constituent of phosphatidylinositol (PI) and phosphoinositides (PIPs) that play important roles in lipid signaling, cell signaling and membrane trafficking, thereby affecting a range of functions in a variety of cell lineages and tissues. Notably, specific PIP derivatives have been associated with an expanding spectrum of neurological disorders (for a review seeCitation72). Based on these pleiotropic consequences for health, and neurological functioning, the inositol degradation function of the microbiome has been identified as one of the Gut-Brain Modules (GBM) involved in the microbiome metabolism of neuro-active or neuro-modulatory compounds.Citation43 Importantly, inositol degradation pathway is among the “rare” GBMs in microbial genomes, which is likely explaining the prominent contribution of L. rhamnosus to this pathway’s activity level in situ in the intestinal tract. The relevance of the high level in situ activity of the inositol pathway in L. rhamnosus remains to be deciphered, but based on the pleiotropic roles of inositol and its derivatives there may be various effects of the modulation of (local-mucosal) inositol levels by L. rhamnosus. In addition, L. rhamnosus has a significant contribution to the Glutamate synthesis II GBM in the SI ecosystem, which can affect glutamate balance in the gut and thereby influence the gut-brain axis due to the critical role that glutamate plays in the central nervous system.Citation73 The significant contribution of L. rhamnosus to the Glutamate synthesis II GBM activity in situ is particularly remarkable because this GBM is considered to be highly prevalent among intestinal bacteria. The prominent role of L. rhamnosus in the in situ expression of these rare and common GBMs warrants further investigation into its influence on the gut-brain axisCitation74 and it is tempting to speculate that these in situ activities of L. rhamnosus CNCM I-3690 could underlie the effects of its consumption on academic stress in healthy adults.Citation13
Altogether, our results clearly demonstrate that L. rhamnosus CNCM I-3690 effectively survives SI passage in humans and persists in this niche for at least 12 h. During its residence in the SI L. rhamnosus CNCM I-3690 drastically adapts its transcriptional profile as compared to in vitro culture conditions, prominently including activation of carbohydrate utilization pathways, cell-surface exposed functions, and a specific ribosomal protein gene cluster. A striking ecosystem contribution of L. rhamnosus CNCM I-3690 to the activity of specific GBMs inspires further investigation of the potential role of this activity in the modulation of the gut-brain axis. Thereby, this study can support the probiotic role of L. rhamnosus CNCM I-3690, which may elicit at least some of its health-benefit effects in the small intestine where it is transiently and abundantly present in a viable and active form, expressing functions that can modulate mucosal biochemistry and/or responses that underlie observed (pre-)clinical effects.
Materials and methods
Subjects
All the adult ileostomy patients without comorbidities recruited for the INSIDE intervention study,Citation15, registered in the US National Library of Medicine http://www.clinicaltrials.gov, ID NCT02920294), were asked to return to the laboratory facility within 4 weeks from the end of the study. As the additional test days extended the total duration of the INSIDE intervention, participants were asked for their separate consent for this part of the study. On the informed consent form, they indicated to consent to participation on these extra test days. Out of the 16 participants of the INSIDE study cohort, 10 participated also in the present study and gave written informed consent. Inclusion criteria did not filter for age, gender, or other demographic parameters due to the limited pool of available volunteers. The nature of our study necessitated working with ileostomy patients, of which there are few without comorbidities and willing to participate in such studies. Importantly, to avoid confounding effects on the study endpoints, study subjects were not allowed to consume pro-, pre- or symbiotics, fresh dairy fermented products (such as yogurt, cottage cheese, buttermilk, or soft-raw cheeses) as well as other food products that are fermented (i.e., sauerkraut) during the study period and three months prior to participation in this trial (a list of forbidden products was provided). Due to the very limited availability of human intervention studies that quantitatively investigate microbial survival in the small intestine after their ingestion, no a priori sample size calculation could be performed.
Study design and intervention products
Study participants received 100 mL of milk fermented by Lacticaseibacillus rhamnosus CNCM I-3690 (L. rhamnosus) intervention product together with a standardized breakfast (bread and jam, coffee or tea at libitum). The intervention product was manufactured by Danone (Danone Nutricia Research, Palaiseau, France) and consisted of 100 mL of milk fermented by Lacticaseibacillus rhamnosus CNCM I-3690 (L. rhamnosus) at approx. 109-5×109 Colony Forming Units (CFU) per mL (i.e., total intake ~ 1011-5×1011 CFU).Citation15 The CFU enumeration of the products was performed by the manufacturer using plating (in De Man, Rogosa and Sharpe, MRS agar supplemented with 1% lactose) and colony counting. Following this breakfast, participants stayed in Maastricht Medical Center for 12 h and collected the total ileostomy effluent during the time intervals of 0–4, 4–8, and 8–12 h after breakfast using 500 mL collection tubes (Corning, New York, United States). As a reference, an ileostomy effluent sample was taken just before product intake and breakfast (approximately 5 mL). Notably, the study lacks the 8–12 H sample of VOL01 because this participant could only take part in the first 8 h of the study. In addition, the 0-4 H and 4–8 H samples of VOL05 contained only very small effluent volumes (<5 mL), disallowing the L. rhamnosus CNCM I-3690 survival assessment, and only allowing the 16S analysis of the small intestine microbiome composition.
Survival determination
Ileostomy effluent volumes were determined, and the samples were kept on ice throughout the whole process. Initially, the samples were homogenized using Ultra-Turrax t50 (IKA, Germany), and when necessary defined volumes of sterile phosphate-buffered saline (PBS) solution were added to the sample to facilitate homogenization, and in the latter cases the consequential dilution factor was recorded. The viability of the L. rhamnosus population was determined using plating and colony enumeration, and using viability PCR (V-PCR). V-PCR is a molecular technique that combines qPCR and the PMAxx dye (Biotium, Fremont, CA, USA), a high affinity photoreactive DNA-binding dye. Upon light exposure, PMAxx covalently binds to DNA, preventing it from being amplified by PCR. The dye is membrane-impermeant, thus, it binds only to free DNA or DNA of bacteria with compromised cell membranes, enabling the discrimination between live and dead (compromised) bacteria.Citation75,Citation76
Following homogenization, part of the sample was aliquoted in triplicate (2 mL) and processed for live/dead bacterial ‘staining’ using PMAxx (see below), while 1 mL was serial diluted (1:10) and plated in duplicate on MRS agar plates supplemented with vancomycin (200 mg/L; Lacticaseibacillus rhamnosus CNCM I-3690 is naturally resistant to this antibiotic). After overnight incubating at 37°C, the colonies were counted, and the identity of the colonies was confirmed by strain-specific colony PCR using a random selection of 10–15 colonies per plate. The specificity of the primers used (FOR AS113: GTGACAACCGCAATCACTTG, REV AS114: TATCGGTGCCATTGAGTGAA), targeting a gene encoding a putative transcriptional regulator of the Cro/CI family, was verified both in silico via BLAST search paying particular attention to align the sequences with genomes of bacterial genera typically found in ileostomy effluents, and by performing PCR on DNA extracted from ileostomy effluents sampled prior L. rhamnosus product consumption.
For the samples designated for live-dead staining, debris was removed by slow-spin centrifugation (700 g, 15 min at 4°C), followed by harvesting the bacterial pellet (4000 g for 45 min at 4°C), which was subsequently washed in 1 mL of ice-cold sterile PBS, harvested again (4000 g for 45 min at 4°C) and finally resuspended in 800 μL of 10 mM Tris-Cl, pH 8.5. These samples were equally aliquoted into two vials (~400 μL each) and analyzed for total and intact (viable) bacterial counts. To determine intact (viable) bacteria, 2.2 μL of 20 mM PMAxx dye was added to the samples, followed by incubation in the dark for 10 min with periodic mixing, after which the samples were placed into the PMA-Lite LED Photolysis (Biotium, CA, USA) and exposed to the light for 17 min. This photoactivation step is essential for the dye to covalently bind to the bacterial DNA in cells that were penetrable by the dye (i.e., had compromised membrane integrity), thereby excluding this DNA from PCR-amplification. The matching sample aliquots with or without PMAxx were subjected to identical incubation and light exposure procedures. DNA was extracted using the DNA Faecal/Soil Microbe Kit (Zymo, CA, U.S.A) according to the manufacturer’s instructions. V-PCR was performed in triplicate for each treated and non-treated sample using iQ™ SYBR® Green buffer (Biorad, CA, U.S.A.) and the same primers as those used for the colony identification (FORAS113:GTGACAACCGCAATCACTTG, REV AS114: TATCGGTGCCATTGAGTGAA). The reactions were initiated at 95°C (5 min), followed by 40 amplification cycles with denaturation at 95°C for 10 s and annealing 58°C for 45 s and extension at 72°C or 1 min. The amplification of a single product was confirmed by running a melting curve analysis for all the samples (65–95°C with 0.5°C intervals) and agarose gel electrophoresis analysis of the qPCR products. A threshold cycle (Ct) value was derived from the V-PCR assay, and the Ct value was converted to colony-forming unit (CFU/mL) using a standard curve. The calibration curve was obtained using purified genomic DNA, quantified via Qubit® Fluorimeter (Thermo Fisher Scientific, MA, U.S.A.), extracted from a suspension of L. rhamnosus, and serially diluted to yield a concentration range corresponding to 10Citation7−10Citation3 CFU/ml. Before this, we evaluated the possible effect that chemical and physical characteristics of the ileostomy effluent may have on the limit of quantification, on the calibration curve quantification, and on the PMAxx efficacy. Therefore, known amounts of L. rhamnosus and of its genomic DNA were spiked in ileostomy effluents belonging to 3 different volunteers, sampled before L. rhamnosus product consumption. The V-PCR mentioned above protocol resulted from this optimization, and the limit of quantification with suitable precision and accuracy was set at LOG 3 CFU/ml. Calibration curves and conversion from Ct to CFU/ml were performed using CFX manager (Biorad, CA, U.S.A.) and GraphPad Prism® Version 9.0 (GraphPad Software, CA, USA). Congruency of V-PCR, indicating the similarity of V-PCR and plating results was calculated as .
Intestinal microbiota composition
Homogenized ileostomy effluent samples (10 mL) were transferred to 15 mL collection tubes (screw cap feces container tubes, Sarstedt, Germany) containing a 2× concentrated DNA shield (Zymo Research, CA, USA) and stored at −20°C until further processing. Samples were thawed, and larger debris were removed by low-speed centrifugation (4°C, 500 g, 3 minutes, no breaks), followed by microbiota collection by high-speed centrifugation (at 4°C, 8000 g, 10 minutes). DNA was extracted from the pellets obtained using the DNA Faecal/Soil Microbe Kit (Zymo, CA, U.S.A), according to manufacturer’s instruction. The DNA quantity and quality were checked by gel electrophoresis and Nanodrop DeNovix DS-11 spectrophotometer (DeNovix Inc., USA). The production of paired-end sequencing of the Illumina 16S rRNA gene amplicon libraries (V3–V4 region, primer 341F: CCTAYGGGRBGCASCAG and primer 806 R: GGACTACNNGGGTATCTAAT), was performed by Novogene (Hong Kong, China). The results were analyzed using CLC Genomics Workbench version v7.5.1 and the CLC Microbial Genomics Module version 1.5 (CLC bio, Arhus, Denmark). Briefly, the paired-end reads were merged into one high-quality representative (mismatch cost = 1, minimum score = 40, Gap Cost = 4, maximum unaligned end mismatches = 5), and primer and quality trimming processed (quality score = 0.05, trim ambiguous nucleotides: maximum number of ambiguities: 2, discards reads below length = 5). The remaining high-quality sequences were clustered into operational taxonomic units (OTU) using the SILVA 16S v132 97% database as the mapping reference database. A total number of paired-end, unique reads of ~1.6 M, with an average of 41,160 reads/samples (max: 58100, min: 26261), were clustered in 5101 operational taxonomic units (OTUs), of which 4566 with more than two counts.
The legitimate assignment of OTU-sequences to L. rhamnosus CNCM I-3690 was confirmed as previously described.Citation15,Citation35
In vitro and in situ transcriptome analyses
For the transcriptome analysis of in vitro cultured L. rhamnosus CNCM I-3690, bacteria were inoculated in and grown overnight at 37°C in milk, or in in-house made MRS that lacked an added carbon source (MRS-C), which was supplemented with 1% glucose (MRS+G), lactose (MRS+L) or mannose (MRS+M). The next day the overnight cultures were subcultured (1:100) in triplicate in the same medium, and bacterial growth at 37°C was monitored in MRS by measurement of the optical density at 600 nm (OD600) with a Microplate Spectrophotometer (SpectraMax M5, Molecular Devices, San Jose, California, USA). The bacterial growth in milk was followed via time-series sample collection, dilution, and plating in MRS agar while recording the pH changes. The colonies were counted after 24 h at 37°C. The bacterial cultures (5 mL) were sampled when they reached mid-exponential phase: 6–7 h from subculturing; ~ pH 4.8 in milk, OD600 ~1.2 in MRS+G and MRS+L. Bacterial cells were harvested by centrifugation (4°C, 12000 g, 3 min) immediately after sample collection, and the pellet was snap-frozen in liquid nitrogen until further processing. Notably, L. rhamnosus grown in MRS-C and MRS+M never reached a high cell-density (final OD600 ~ 0.8) and were therefore sampled at a lower OD600 (~0.4) in higher volumes (20 mL). The L. rhamnosus in situ transcriptome, when it resides in the small intestine, was determined by metatranscriptome analysis of the ileostomy effluent samples that were previously collected for that purpose.Citation15 The bacterial pellets obtained from in vitro cultured and effluent samples were resuspended in 100 µl of phenol-chloroform – isoamyl alcohol (pH 6.5–8.0, Sigma, Germany). RNA was extracted using the RNeasy PowerMicrobiome Kit (Qiagen, Germany) following the manufacturer’s instructions. RNA quality and quantity were analyzed by agarose gel electrophoresis and the TapeStation 2200 (Agilent Technologies, CA, United States), and RNA samples were stored at −80°C. The 250 ~ 300 bp insert cDNA library with rRNA removal (Ribo-ZeroTM Magnetic Kit, Illumina, USA) and sequencing was performed by Novogene (Hong Kong, China) using the Illumina HiSeq2500 platform (PE150, 12GB raw data/sample for the metatranscriptome analysis and 4 G raw data/samples for the in vitro transcriptome analysis).
Low-quality sequence regions and adapter-derived sequences were removed from the Illumina reads with fastp (v0.19.5) using default settings. The remaining rRNA sequences were removed with BBDuk (v38.32) using parameters k = 31 and ref=riboKmers.fa.gz. The RNAseq data obtained from in vitro cultured L. rhamnosus and the complete metatranscriptome RNAseq data obtained from the ileostomy effluent samples (n = 12Citation15) were mapped against the L. rhamnosus CNCM I-3690 genome followed by determination of mappings per gene to obtain read counts per gene with BBmap default settings and its corresponding Reads per kilobase per million mapped reads (rpkm). All ORFs that were detected to be transcribed were functionally re-annotated using KofamKOALA with KEGG v.92. SignalP 5.0 with the default settingsCitation45 was used to predict the presence of surface-associated and/or extracellular proteins in L. rhamnosus genome. For the remaining metatranscriptome reads that were not mapped to the L. rhamnosus CNCM I-3690 genome, the Human Gut Integrated Gene Catalog (IGC) was used for read mapping using BBmap with the following parameters: covstats, out, idhist, indelhist, ehist, sortscafs, statsfil, rpkm, ambig=random, unpigz=t, machineout=t, intronlen = 20, maxindel = 20, strictmaxindel=t. The values in the rpkm files were used to further process the read counts per gene. For taxonomic classification of the genes in the IGC database, the online available classifications were used (https://db.cngb.org/microbiome/genecatalog/genecatalog_human).
Statistical analyses
The microbial compositional data was filtered per reads count (at least two reads in 10% of the samples), MicrobiomeAnalystCitation77 was used for the calculation of α-diversity indices, including Observed species, Shannon and Chao1. The β-diversity, distance-based redundancy analysis (db-RDA), principal component analyses (PCA), partial and non-redundancy analysis (p/RDA), were obtained using the Canoco 5.10 software suiteCitation78 using 1000 permutations in (p-)RDAs to assess the significance, when needed, the relative abundance were log-transformed (Y’= Y + 1000) and centered. The effect of variables such as gender and time was evaluated by RDA and pRDA separately and excluded as co-variates in further analyses as they did not significantly impact the microbiota composition. Differential expression and abundance analyses were performed via Empirical Analysis of Digital Gene Expression (edgeR)Citation79 implemented in Network AnalystCitation80 after trimmed means of M-values (TMM) transformation, and false discovery rate (FDR) was used to correct for multiple testing, and an FDR adjusted p-value < 0.05 was considered statistically significant. The Gene Set Enrichment Analysis was performed via GSEA-pro V3 (http://gseapro.molgenrug.nl, Groningen University, the Netherlands.Citation81. GOmixer version 1.7.5.0. (http://www.raeslab.org/omixer/) was used for the dedicated functional analysis of the Gut-Brain modules with the default settings. Heatmaps were made in r, ComplexHeatmap, Bioconductor release 3.12,Citation82 hierarchical clustering using complete linkage based on Euclidian distances. The average gene expression per volunteer was used to calculate the fold change from the row mean for each pathway or module displayed in the heatmaps. The Coefficient of Variation was calculated as the percental ratio of the standard deviation and the mean of the in situ sample expression values. The “contribution enrichment factor” was calculated as log2 of the fraction of all L. rhamnosus reads mapped to a KEGG category over the fraction of all other reads mapped to that KEGG category. GraphPad Prism (9 for Windows, CA, USA) was used for graphical outputs and the Wilcoxon tests with Holm-Sidak Post hoc test and the Mann–Whitney test with Holm-Sidak post hoc test.
Availability of data and materials
The Metatranscriptome dataset supporting the conclusions of this article is available in the DANS repository, on the following doi: https://doi.org/10.17026/dans-xvh-yww8. The 16S data and the In vitro transcriptome data are available in the ENA repository under the following accession number: PRJEB61139 and PRJEB61087, respectively.
Ethics approval and consent to participate
This study was approved by the Medical Ethics Committee of Maastricht University (MU), Maastricht, the Netherlands, and performed in full accordance with the Declaration of Helsinki (latest amendment by the World Medic Association in Fortaleza, Brazil, in 2013) and Dutch Regulations on Medical Research involving Human Subjects (WMO, 1998), as well as with the International Conference on Harmonisation – Good Clinical Practice (ICH-GCP) guidelines. The study was performed at MU from 27 October 2016 (screening visit, first subject) until 27 October 2017 (last visit last subject). This study was registered in the US National Library of Medicine (http://www.clinicaltrials.gov, ID NCT NCT02920294). All subjects gave written informed consent before screening.
Supplemental Material
Download MS Word (401.8 KB)Disclosure statement
This author discloses the following: T. Smokvina and C. Chervaux are employees of Danone Nutricia Research. The remaining authors declare that they have no competing interests.
Supplementary material
Supplemental data for this article can be accessed online at https://doi.org/10.1080/19490976.2023.2244720
Additional information
Funding
References
- Hill C, Guarner F, Reid G, Gibson GR, Merenstein DJ, Pot B, Morelli L, Canani RB, Flint HJ, Salminen S, et al. Expert consensus document. The international scientific association for probiotics and prebiotics consensus statement on the scope and appropriate use of the term probiotic. Nat Rev Gastroenterol Hepatol. 2014;11(8):506–21. doi:10.1038/nrgastro.2014.66.
- Andrade ME, Araujo RS, de Barros PA, Soares AD, Abrantes FA, Generoso SDV, Fernandes SOA, Cardoso VN. The role of immunomodulators on intestinal barrier homeostasis in experimental models. Clin Nutr. 2015;34(6):1080–1087. doi:10.1016/j.clnu.2015.01.012.
- Kant R, Rintahaka J, Yu X, Sigvart-Mattila P, Paulin L, Mecklin JP, Saarela M, Palva A, von Ossowski I. A comparative pan-genome perspective of niche-adaptable cell-surface protein phenotypes in Lactobacillus rhamnosus. PLoS One. 2014;9(7):e102762. doi:10.1371/journal.pone.0102762.
- DuPont AW, DuPont HL. The intestinal microbiota and chronic disorders of the gut. Nat Rev Gastroenterol Hepatol. 2011;8(9):523–531. doi:10.1038/nrgastro.2011.133.
- van Baarlen P, Wells JM, Kleerebezem M. Regulation of intestinal homeostasis and immunity with probiotic lactobacilli. Trends Immunol. 2013;34(5):208–215. doi:10.1016/j.it.2013.01.005.
- Zhu Y, Wang X, Zhu L, Tu Y, Chen W, Gong L, Pan T, Lin H, Lin J, Sun H, et al. Lactobacillus rhamnosus GG combined with inosine ameliorates alcohol-induced liver injury through regulation of intestinal barrier and Treg/Th1 cells. Toxicol Appl Pharmacol. 2022;439:115923. doi:10.1016/j.taap.2022.115923.
- Laval L, Martin R, Natividad JN, Chain F, Miquel S, Desclee de Maredsous C, Capronnier S, Sokol H, Verdu EF, van Hylckama Vlieg J, et al. Lactobacillus rhamnosus CNCM I-3690 and the commensal bacterium Faecalibacterium prausnitzii A2-165 exhibit similar protective effects to induced barrier hyper-permeability in mice. Gut Microbes. 2015;6(1):1–9. doi:10.4161/19490976.2014.990784.
- Martin R, Chamignon C, Mhedbi-Hajri N, Chain F, Derrien M, Escribano-Vazquez U, Garault P, Cotillard A, Pham HP, Chervaux C, et al. The potential probiotic Lactobacillus rhamnosus CNCM I-3690 strain protects the intestinal barrier by stimulating both mucus production and cytoprotective response. Sci Rep. 2019;9(1):5398. doi:10.1038/s41598-019-41738-5.
- Bhat MI, Sowmya K, Kapila S, Kapila R. Potential probiotic lactobacillus rhamnosus (MTCC-5897) Inhibits Escherichia coli impaired intestinal barrier function by modulating the host tight junction gene response. Probiotics Antimicrob Proteins. 2020;12(3):1149–1160. doi:10.1007/s12602-019-09608-8.
- Grompone G, Martorell P, Llopis S, Gonzalez N, Genoves S, Mulet AP, Fernández-Calero T, Tiscornia I, Bollati-Fogolín M, Chambaud I, et al. Anti-inflammatory Lactobacillus rhamnosus CNCM I-3690 strain protects against oxidative stress and increases lifespan in Caenorhabditis elegans. PLoS One. 2012;7(12):e52493. doi:10.1371/journal.pone.0052493.
- Natividad JM, Lamas B, Pham HP, Michel ML, Rainteau D, Bridonneau C, da Costa G, van Hylckama Vlieg J, Sovran B, Chamignon C, et al. Bilophila wadsworthia aggravates high fat diet induced metabolic dysfunctions in mice. Nat Commun. 2018;9(1):2802. doi:10.1038/s41467-018-05249-7.
- Wang J, Tang H, Zhang C, Zhao Y, Derrien M, Rocher E, van-Hylckama Vlieg JE, Strissel K, Zhao L, Obin M, et al. Modulation of gut microbiota during probiotic-mediated attenuation of metabolic syndrome in high fat diet-fed mice. Isme J. 2015;9(1):1–15. doi:10.1038/ismej.2014.99.
- Wauters L, Van Oudenhove L, Accarie A, Geboers K, Geysen H, Toth J, Luypaerts A, Verbeke K, Smokvina T, Raes J, et al. Lactobacillus rhamnosus CNCM I-3690 decreases subjective academic stress in healthy adults: a randomized placebo-controlled trial. Gut Microbes. 2022;14(1):2031695. doi:10.1080/19490976.2022.2031695.
- Derrien M, van Hylckama Vlieg JE. Fate, activity, and impact of ingested bacteria within the human gut microbiota. Trends Microbiol. 2015;23(6):354–366. doi:10.1016/j.tim.2015.03.002.
- Zaccaria E, Klaassen T, Alleleyn AME, Boekhorst J, Smokvina T, Kleerebezem M, Troost FJ. Endogenous small intestinal microbiome determinants of transient colonisation efficiency by bacteria from fermented dairy products: a randomised controlled trial. Microbiome. 2023;11(1):43. doi:10.1186/s40168-023-01491-4.
- Zhang C, Derrien M, Levenez F, Brazeilles R, Ballal SA, Kim J, Degivry M-C, Quéré G, Garault P, van Hylckama Vlieg JET, et al. Ecological robustness of the gut microbiota in response to ingestion of transient food-borne microbes. Isme J. 2016;10(9):2235–2245. doi:10.1038/ismej.2016.13.
- Zmora N, Zilberman-Schapira G, Suez J, Mor U, Dori-Bachash M, Bashiardes S, Kotler E, Zur M, Regev-Lehavi D, Brik RBZ, et al. Personalized gut mucosal colonization resistance to empiric probiotics is associated with unique host and microbiome features. Cell. 2018;174(6):1388–1405.e21. doi:10.1016/j.cell.2018.08.041.
- Volk N, Lacy B. Anatomy and physiology of the small bowel. Gastrointest Endosc Clin N Am. 2017;27(1):1–13. doi:10.1016/j.giec.2016.08.001.
- Martinez-Guryn K, Hubert N, Frazier K, Urlass S, Musch MW, Ojeda P, Pierre JF, Miyoshi J, Sontag TJ, Cham CM, et al. Small intestine microbiota regulate host digestive and absorptive adaptive responses to dietary lipids. Cell Host & Microbe. 2018;23(4):458–469.e5. doi:10.1016/j.chom.2018.03.011.
- Bauer PV, Duca FA, Waise TMZ, Rasmussen BA, Abraham MA, Dranse HJ, Puri A, O’Brien CA, Lam TKT. Metformin alters upper small intestinal microbiota that impact a glucose-SGLT1-sensing glucoregulatory pathway. Cell Metab. 2018;27(1):101–117.e5. doi:10.1016/j.cmet.2017.09.019.
- Sayin SI, Wahlstrom A, Felin J, Jantti S, Marschall HU, Bamberg K, Angelin B, Hyötyläinen T, Orešič M, Bäckhed F, et al. Gut microbiota regulates bile acid metabolism by reducing the levels of tauro-beta-muricholic acid, a naturally occurring FXR antagonist. Cell Metab. 2013;17(2):225–235. doi:10.1016/j.cmet.2013.01.003.
- Wexler AG, Schofield WB, Degnan PH, Folta-Stogniew E, Barry NA, Goodman AL. Human gut Bacteroides capture vitamin B12 via cell surface-exposed lipoproteins. Elife. 2018;7. doi:10.7554/eLife.37138.
- Shearer MJ, Newman P. Recent trends in the metabolism and cell biology of vitamin K with special reference to vitamin K cycling and MK-4 biosynthesis. J Lipid Res. 2014;55(3):345–362. doi:10.1194/jlr.R045559.
- Tuganbaev T, Mor U, Bashiardes S, Liwinski T, Nobs SP, Leshem A, Dori-Bachash M, Thaiss CA, Pinker EY, Ratiner K, et al. Diet diurnally regulates small intestinal microbiome-epithelial-immune homeostasis and enteritis. Cell. 2020;182(6):1441–1459.e21. doi:10.1016/j.cell.2020.08.027.
- Saffouri GB, Shields-Cutler RR, Chen J, Yang Y, Lekatz HR, Hale VL, Cho JM, Battaglioli EJ, Bhattarai Y, Thompson KJ, et al. Small intestinal microbial dysbiosis underlies symptoms associated with functional gastrointestinal disorders. Nat Commun. 2019;10(1):2012. doi:10.1038/s41467-019-09964-7.
- Bures J, Cyrany J, Kohoutova D, Forstl M, Rejchrt S, Kvetina J, Vorisek V, Kopacova M. Small intestinal bacterial overgrowth syndrome. World J Gastroenterol. 2010;16(24):2978–2990. doi:10.3748/wjg.v16.i24.2978.
- Chen RY, Kung VL, Das S, Hossain MS, Hibberd MC, Guruge J, Mahfuz M, Begum SMKN, Rahman MM, Fahim SM, et al. Duodenal microbiota in stunted undernourished children with enteropathy. N Engl J Med. 2020;383(4):321–333. doi:10.1056/NEJMoa1916004.
- Davidovics ZH, Carter BA, Luna RA, Hollister EB, Shulman RJ, Versalovic J. The fecal microbiome in pediatric patients with short bowel syndrome. JPEN J Parenter Enteral Nutr. 2016;40(8):1106–1113. doi:10.1177/0148607115591216.
- Engstrand Lilja H, Wefer H, Nyström N, Finkel Y, Engstrand L. Intestinal dysbiosis in children with short bowel syndrome is associated with impaired outcome. Microbiome. 2015;3(1):18. doi:10.1186/s40168-015-0084-7.
- Zoetendal EG, Raes J, van den Bogert B, Arumugam M, Booijink CC, Troost FJ, Bork P, Wels M, de Vos WM, Kleerebezem M, et al. The human small intestinal microbiota is driven by rapid uptake and conversion of simple carbohydrates. Isme J. 2012;6(7):1415–1426. doi:10.1038/ismej.2011.212.
- Hayashi H, Takahashi R, Nishi T, Sakamoto M, Benno Y. Molecular analysis of jejunal, ileal, caecal and recto-sigmoidal human colonic microbiota using 16S rRNA gene libraries and terminal restriction fragment length polymorphism. J Med Microbiol. 2005;54(11):1093–1101. doi:10.1099/jmm.0.45935-0.
- Shalon D, Culver RN, Grembi JA, Folz J, Treit PV, Shi H, Rosenberger FA, Dethlefsen L, Meng X, Yaffe E, et al. Profiling the human intestinal environment under physiological conditions. Nature. 2023;617(7961):581–591. doi:10.1038/s41586-023-05989-7.
- De la Paz E, Maganti NH, Trifonov A, Jeerapan I, Mahato K, Yin L, Sonsa-Ard T, Ma N, Jung W, Burns R, et al. A self-powered ingestible wireless biosensing system for real-time in situ monitoring of gastrointestinal tract metabolites. Nat Commun. 2022;13(1):7405. doi:10.1038/s41467-022-35074-y.
- Folz J, Culver RN, Morales JM, Grembi J, Triadafilopoulos G, Relman DA, Huang KC, Shalon D, Fiehn O. Human metabolome variation along the upper intestinal tract. Nat Metab. 2023;5(5):777–788. doi:10.1038/s42255-023-00777-z.
- van den Bogert B, Erkus O, Boekhorst J, de Goffau M, Smid EJ, Zoetendal EG, Kleerebezem M. Diversity of human small intestinal Streptococcus and Veillonella populations. FEMS Microbiol Ecol. 2013;85(2):376–388. doi:10.1111/1574-6941.12127.
- El Aidy S, van den Bogert B, Kleerebezem M. The small intestine microbiota, nutritional modulation and relevance for health. Curr Opin Biotechnol. 2015;32:14–20. doi:10.1016/j.copbio.2014.09.005.
- Keane C, Sharma P, Yuan L, Bissett I, O’Grady G. Impact of temporary ileostomy on long-term quality of life and bowel function: a systematic review and meta-analysis. ANZ J Surg. 2020;90(5):687–692. doi:10.1111/ans.15552.
- Van den Bogert B, Boekhorst J, Herrmann R, Smid EJ, Zoetendal EG, Kleerebezem M, van Schaik W. Comparative genomics analysis of Streptococcus isolates from the human small intestine reveals their adaptation to a highly dynamic ecosystem. PLoS One. 2013;8(12):e83418. doi:10.1371/journal.pone.0083418.
- Oozeer R, Leplingard A, Mater DD, Mogenet A, Michelin R, Seksek I, Marteau P, Dore J, Bresson J-L, Corthier G, et al. Survival of Lactobacillus casei in the human digestive tract after consumption of fermented milk. Appl Environ Microbiol. 2006;72(8):5615–5617. doi:10.1128/AEM.00722-06.
- Takada T, Chinda D, Mikami T, Shimizu K, Oana K, Hayamizu S, Miyazawa K, Arai T, Katto M, Nagara Y, et al. Dynamic analysis of human small intestinal microbiota after an ingestion of fermented milk by small-intestinal fluid perfusion using an endoscopic retrograde bowel insertion technique. Gut Microbes. 2020;11(6):1662–1676. doi:10.1080/19490976.2020.1766942.
- Wang J, Zhang J, Liu W, Zhang H, Sun Z. Metagenomic and metatranscriptomic profiling of Lactobacillus casei Zhang in the human gut. NPJ Biofilms Microbiomes. 2021;7(1):55. doi:10.1038/s41522-021-00227-2.
- Vieira-Silva S, Falony G, Darzi Y, Lima-Mendez G, Garcia Yunta R, Okuda S, Vandeputte D, Valles-Colomer M, Hildebrand F, Chaffron S, et al. Species–function relationships shape ecological properties of the human gut microbiome. Natr Microbiol. 2016;1(8):16088. doi:10.1038/nmicrobiol.2016.88.
- Valles-Colomer M, Falony G, Darzi Y, Tigchelaar EF, Wang J, Tito RY, Schiweck C, Kurilshikov A, Joossens M, Wijmenga C, et al. The neuroactive potential of the human gut microbiota in quality of life and depression. Natr Microbiol. 2019;4(4):623–632. doi:10.1038/s41564-018-0337-x.
- Desfosses-Foucault E, Dussault-Lepage V, Le Boucher C, Savard P, Lapointe G, Roy D. Assessment of probiotic viability during cheddar cheese manufacture and ripening using propidium monoazide-PCR quantification. Front Microbiol. 2012;3:350. doi:10.3389/fmicb.2012.00350.
- Almagro Armenteros JJ, Tsirigos KD, Sonderby CK, Petersen TN, Winther O, Brunak S, von Heijne G, Nielsen H. SignalP 5.0 improves signal peptide predictions using deep neural networks. Nat Biotechnol. 2019;37(4):420–423. doi:10.1038/s41587-019-0036-z.
- Petrova MI, Lievens E, Verhoeven TL, Macklaim JM, Gloor G, Schols D, Vanderleyden J, Reid G, Lebeer S. The lectin-like protein 1 in Lactobacillus rhamnosus GR-1 mediates tissue-specific adherence to vaginal epithelium and inhibits urogenital pathogens. Sci Rep. 2016;6(1):37437. doi:10.1038/srep37437.
- Frese SA, Mackenzie DA, Peterson DA, Schmaltz R, Fangman T, Zhou Y, Zhang C, Benson AK, Cody LA, Mulholland F, et al. Molecular characterization of host-specific biofilm formation in a vertebrate gut symbiont. PLoS Genet. 2013;9(12):e1004057. doi:10.1371/journal.pgen.1004057.
- Olsson LM, Boulund F, Nilsson S, Khan MT, Gummesson A, Fagerberg L, Engstrand L, Perkins R, Uhlén M, Bergström G, et al. Dynamics of the normal gut microbiota: A longitudinal one-year population study in Sweden. Cell Host & Microbe. 2022;30(5):726–739.e3. doi:10.1016/j.chom.2022.03.002.
- David LA, Materna AC, Friedman J, Campos-Baptista MI, Blackburn MC, Perrotta A, Erdman SE, Alm EJ. Host lifestyle affects human microbiota on daily timescales. Genome Biol. 2014;15(7):R89. doi:10.1186/gb-2014-15-7-r89.
- Mardinoglu A, Wu H, Bjornson E, Zhang C, Hakkarainen A, Rasanen SM, Lee S, Mancina RM, Bergentall M, Pietiläinen KH, et al. An integrated understanding of the rapid metabolic benefits of a carbohydrate-restricted diet on hepatic steatosis in humans. Cell Metab. 2018;27(3):559–571.e5. doi:10.1016/j.cmet.2018.01.005.
- Bron PA, Grangette C, Mercenier A, de Vos WM, Kleerebezem M. Identification of Lactobacillus plantarum genes that are induced in the gastrointestinal tract of mice. J Bacteriol. 2004;186:5721–5729. doi:10.1128/JB.186.17.5721-5729.2004.
- Marco ML, Peters TH, Bongers RS, Molenaar D, van Hemert S, Sonnenburg JL, Gordon JI, Kleerebezem M. Lifestyle of Lactobacillus plantarum in the mouse caecum. Environ Microbiol. 2009;11(10):2747–2757. doi:10.1111/j.1462-2920.2009.02001.x.
- Marco ML, de Vries MC, Wels M, Molenaar D, Mangell P, Ahrne S, de Vos WM, Vaughan EE, Kleerebezem M. Convergence in probiotic Lactobacillus gut-adaptive responses in humans and mice. Isme J. 2010;4(11):1481–1484. doi:10.1038/ismej.2010.61.
- Bron PA, Meijer M, Bongers RS, de Vos WM, Kleerebezem M. Dynamics of competitive population abundance of Lactobacillus plantarum ivi gene mutants in faecal samples after passage through the gastrointestinal tract of mice. J Appl Microbiol. 2007;103(5):1424–1434. doi:10.1111/j.1365-2672.2007.03376.x.
- Licandro-Seraut H, Scornec H, Pedron T, Cavin JF, Sansonetti PJ. Functional genomics of Lactobacillus casei establishment in the gut. Proc Natl Acad Sci U S A. 2014;111(30):E3101–9. doi:10.1073/pnas.1411883111.
- Muscariello L, Marasco R, De Felice M, Sacco M. The functional ccpA gene is required for carbon catabolite repression in Lactobacillus plantarum. Appl Environ Microbiol. 2001;67(7):2903–2907. doi:10.1128/AEM.67.7.2903-2907.2001.
- Chen C, Lu Y, Wang L, Yu H, Tian H. CcpA-Dependent carbon catabolite repression regulates fructooligosaccharides metabolism in Lactobacillus plantarum. Front Microbiol. 2018;9:1114. doi:10.3389/fmicb.2018.01114.
- Yang Y, Zhang L, Huang H, Yang C, Yang S, Gu Y, Jiang W. A flexible binding site architecture provides new insights into CcpA global regulation in gram-positive bacteria. mBio. 2017;8(1). doi:10.1128/mBio.02004-16.
- Zhu Z, Yang J, Yang P, Wu Z, Zhang J, Du G. Enhanced acid-stress tolerance in Lactococcus lactis NZ9000 by overexpression of ABC transporters. Microb Cell Fact. 2019;18(1):136. doi:10.1186/s12934-019-1188-8.
- Esteban-Torres M, Reveron I, Plaza-Vinuesa L, de Las Rivas B, Munoz R, Lopez de Felipe F. Transcriptional reprogramming at genome-scale of Lactobacillus plantarum WCFS1 in response to olive oil challenge. Front Microbiol. 2017;8:244. doi:10.3389/fmicb.2017.00244.
- Eckhardt TH, Skotnicka D, Kok J, Kuipers OP. Transcriptional regulation of fatty acid biosynthesis in Lactococcus lactis. J Bacteriol. 2013;195(5):1081–1089. doi:10.1128/JB.02043-12.
- Jang HR, Park HJ, Kang D, Chung H, Nam MH, Lee Y, Youn J. A protective mechanism of probiotic Lactobacillus against hepatic steatosis via reducing host intestinal fatty acid absorption. Experimental & Molecular Medicine. 2019;51(12):1–14. doi:10.1038/s12276-019-0352-x.
- Warner JR, McIntosh KB. How common are extraribosomal functions of ribosomal proteins? Mol Cell. 2009;34(1):3–11. doi:10.1016/j.molcel.2009.03.006.
- Yates JL, Nomura ME. E. coli ribosomal protein L4 is a feedback regulatory protein. Cell. 1980;21(2):517–522. doi:10.1016/0092-8674(80)90489-4.
- Singh D, Chang SJ, Lin PH, Averina OV, Kaberdin VR, Lin-Chao S. Regulation of ribonuclease E activity by the L4 ribosomal protein of Escherichia coli. Proc Natl Acad Sci U S A. 2009;106(3):864–869. doi:10.1073/pnas.0810205106.
- Segers ME, Lebeer S. Towards a better understanding of Lactobacillus rhamnosus GG–host interactions. Microb Cell Fact. 2014;Suppl 13(Suppl 1):S7. doi:10.1186/1475-2859-13-S1-S7.
- Ottman N, Reunanen J, Meijerink M, Pietila TE, Kainulainen V, Klievink J, Huuskonen L, Aalvink S, Skurnik M, Boeren S, et al. Pili-like proteins of Akkermansia muciniphila modulate host immune responses and gut barrier function. PLoS One. 2017;12(3):e0173004. doi:10.1371/journal.pone.0173004.
- Muscariello L, De Siena B, Marasco R. Lactobacillus cell surface proteins involved in interaction with mucus and extracellular matrix components. Curr Microbiol. 2020;77(12):3831–3841. doi:10.1007/s00284-020-02243-5.
- Goh YJ, Barrangou R, Klaenhammer TR. In Vivo transcriptome of Lactobacillus acidophilus and colonization impact on murine host intestinal gene expression. mBio. 2021;12(1):12. doi:10.1128/mBio.03399-20.
- Croze ML, Soulage CO. Potential role and therapeutic interests of myo-inositol in metabolic diseases. Biochimie. 2013;95(10):1811–1827. doi:10.1016/j.biochi.2013.05.011.
- Lepore E, Lauretta R, Bianchini M, Mormando M, Di Lorenzo C, Unfer V. Inositols depletion and resistance: principal mechanisms and therapeutic strategies. Int J Mol Sci. 2021;22(13):22. doi:10.3390/ijms22136796.
- Volpatti JR, Al-Maawali A, Smith L, Al-Hashim A, Brill JA, Dowling JJ. The expanding spectrum of neurological disorders of phosphoinositide metabolism. Dis Model Mech. 2019;12(8):12. doi:10.1242/dmm.038174.
- Bak LK, Schousboe A, Waagepetersen HS. The glutamate/GABA-glutamine cycle: aspects of transport, neurotransmitter homeostasis and ammonia transfer. J Neurochem. 2006;98(3):641–653. doi:10.1111/j.1471-4159.2006.03913.x.
- Bravo JA, Forsythe P, Chew MV, Escaravage E, Savignac HM, Dinan TG, Bienenstock J, Cryan JF. Ingestion of Lactobacillus strain regulates emotional behavior and central GABA receptor expression in a mouse via the vagus nerve. Proc Natl Acad Sci U S A. 2011;108(38):16050–16055. doi:10.1073/pnas.1102999108.
- Baymiev AK, Baymiev AK, Kuluev BR, Shvets KY, Yamidanov RS, Matniyazov RT, Chemeris DA, Zubov VV, Alekseev YI, Mavzyutov AR, et al. Modern approaches to differentiation of live and dead bacteria using selective amplification of nucleic acids. Microbiology. 2020;89(1):13–27. doi:10.1134/S0026261720010038.
- Lai CH, Wu SR, Pang JC, Ramireddy L, Chiang YC, Lin CK, Tsen H-Y. Designing primers and evaluation of the efficiency of propidium monoazide – Quantitative polymerase chain reaction for counting the viable cells of Lactobacillus gasseri and Lactobacillus salivarius. J Food Drug Anal. 2017;25(3):533–542. doi:10.1016/j.jfda.2016.10.004.
- Chong J, Liu P, Zhou G, Xia J. Using MicrobiomeAnalyst for comprehensive statistical, functional, and meta-analysis of microbiome data. Nat Protoc. 2020;15(3):799–821. doi:10.1038/s41596-019-0264-1.
- Braak CJFT, Šmilauer P. Canoco reference manual and user’s guide : software for ordination (version 5.10). Wageningen: Biometris, Wageningen University & Research; 2018.
- Robinson MD, McCarthy DJ, Smyth GK. EdgeR: a Bioconductor package for differential expression analysis of digital gene expression data. Bioinformatics. 2010;26:139–140. doi:10.1093/bioinformatics/btp616.
- Zhou G, Soufan O, Ewald J, Hancock REW, Basu N, Xia J. NetworkAnalyst 3.0: a visual analytics platform for comprehensive gene expression profiling and meta-analysis. Nucleic Acids Res. 2019;47(W1):W234–W241. doi:10.1093/nar/gkz240.
- de Jong A, Kuipers OP, Kok J. FUNAGE-Pro: comprehensive web server for gene set enrichment analysis of prokaryotes. Nucleic Acids Res. 2022;50(W1):W330–W336. doi:10.1093/nar/gkac441.
- Gu Z, Eils R, Schlesner M. Complex heatmaps reveal patterns and correlations in multidimensional genomic data. Bioinformatics. 2016;32(18):2847–2849. The following additional file is provided with the manuscript. doi:10.1093/bioinformatics/btw313.