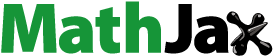
ABSTRACT
Although fecal microbiota composition is considered to preserve relevant and representative information for distal colonic content, it is evident that it does not represent microbial communities inhabiting the small intestine. Nevertheless, studies investigating the human small intestinal microbiome and its response to dietary intervention are still scarce. The current study investigated the spatio-temporal dynamics of the small intestinal microbiome within a day and over 20 days, as well as its responses to a 14-day synbiotic or placebo control supplementation in 20 healthy subjects. Microbial composition and metabolome of luminal content of duodenum, jejunum, proximal ileum and feces differed significantly from each other. Additionally, differences in microbiota composition along the small intestine were most pronounced in the morning after overnight fasting, whereas differences in composition were not always measurable around noon or in the afternoon. Although overall small intestinal microbiota composition did not change significantly within 1 day and during 20 days, remarkable, individual-specific temporal dynamics were observed in individual subjects. In response to the synbiotic supplementation, only the microbial diversity in jejunum changed significantly. Increased metabolic activity of probiotic strains during intestinal passage, as assessed by metatranscriptome analysis, was not observed. Nevertheless, synbiotic supplementation led to a short-term spike in the relative abundance of genera included in the product in the small intestine approximately 2 hours post-ingestion. Collectively, small intestinal microbiota are highly dynamic. Ingested probiotic bacteria could lead to a transient spike in the relative abundance of corresponding genera and ASVs, suggesting their passage through the entire gastrointestinal tract. This study was registered to http://www.clinicaltrials.gov, NCT02018900.
Introduction
The human intestinal tract plays an important role in food digestion, nutrient absorption, and fermentation of unabsorbed components. Along the entire length of the intestinal tract, large numbers of microbes are present, collectively called intestinal microbiota, which plays an important role in human health.Citation1 In most studies, feces are used as a proxy for colonic microbiota due to ease of access. Although fecal microbiota composition is considered to preserve relevant and representative information for distal colonic content, it is evident that it does not represent microbial communities inhabiting the small intestine.Citation2–4
The small intestinal microbiota interacts with its host through many ways, including immune modulation, production of antimicrobial compounds and metabolites (e.g. short-chain fatty acids (SCFAs) and vitamins).Citation1,Citation5 The level of expression of SCFA receptors was found to be higher in the human ileum than in the distal colon.Citation6 Therefore, investigating factors that influence composition and functionality of the small intestinal microbiota can have important implications for our ability to understand its role in host health.
The human small intestine is much longer than the large intestine and is divided into different regions referred to as duodenum, jejunum and ileum. The small intestinal environment is characterized by a relatively short transit time and rapid luminal flux, as well as more gastric acid exposure and high concentrations of digestive enzymes, antimicrobial peptides and bile acids, which together act as selective forces for its inhabiting microbes.Citation7,Citation8 Nevertheless, due to the complications associated with sample collection, available human studies investigating the spatial differences in small intestinal microbiota are often based on only one or a few subjects, or comparing samples from different locations that are derived from different subjects.Citation9–13 As an alternative, collection of effluent from people with an end-ileostomy, i.e. subjects without a colon, enables repeated sampling in a noninvasive way.Citation14 However, ileostoma effluent cannot be used to study the spatial differences within small intestinal microbial composition and functionality. To this end, the recent development of techniques for delivery of compounds to or aspirating intestinal fluids from the intestinal lumen,Citation15,Citation16 including ingestible cathethers, now allows the study of dynamic processes in the intestinal lumen.
To date, the majority of dietary intervention studies investigated their impact on the fecal microbiota. In turn, the small intestinal microbiota that is more sensitive to dietary changes,Citation5,Citation17–20 received only limited attention.
In the current study, we aimed to investigate the spatial and temporal dynamics of the microbial and metabolomic composition in the luminal content of duodenum, jejunum, proximal ileum and feces, as well as their response to a synbiotic supplementation. The synbiotic was chosen because the probiotic mixture was previously shown to convey positive effects on intestinal barrier function in vitro and in vivo,Citation21,Citation22 being early intestinal colonizers,Citation23 and because the prebiotic has been shown to stimulate the activity of the probiotic mixture.Citation24 We hypothesized that microbiota composition differs per sampling site and that these location-specific microbial communities respond differently to the synbiotic supplementation.
Materials and methods
This study was approved by the Medical Ethics Committee of Maastricht University Medical Centre+ and registered at the US National Library of Medicine (http://www.clinicaltrials.gov, NCT02018900). All subjects gave written informed consent before screening. All authors had access to the study data and reviewed and approved the final manuscript.
Study overview
Details of the study design have been reported earlier.Citation24 This study followed a double-blind, randomized, controlled, parallel design. The 20 healthy adults (Figure S1) were randomly assigned to the placebo control (n = 10; P1 – P10) or synbiotic group (n = 10; S1 – S10) with no significant differences in age and BMI (Table S1). None of the participants took any medication 14 days prior to the study, nor antibiotics 90 days prior to the study. Subjects in the synbiotic group took 6 g/day of the probiotic mixture Ecologic®825 (1.5 × 1010 CFU/day, Winclove Probiotics BV, Amsterdam, the Netherlands) combined with 10 g/day FOS P6 (degree of polymerization 3–5) in two equal dosages. Ecologic®825 was composed of nine probiotic strains, namely Lactococcus lactis, Lacticaseibacillus paracasei, Lactobacillus acidophilus, Ligilactobacillus salivarius, Lacticaseibacillus casei, Lactiplantibacillus plantarum, Bifidobacterium bifidum, Bifidobacterium lactis and Bifidobacterium lactis. The control group received 10 g/day maltodextrin and 6 g/day of the probiotic carrier material. Subjects ingested the supplements for 14 days. Subjects ingested the supplements after dissolving the contents of the duo sachets in 200 ml water every morning and evening at the same time for 14 days
Sample collection and storage
The luminal content (from duodenum, jejunum and proximal ileum) was sampled using a multi-lumen customized sampling catheter (Mui Scientific, Mississauga, Ontario, Canada). The catheter was 270 cm long and contained four individual lumina, three of which contained side-holes at, respectively, 5, 65, and 125 cm proximal to the distal catheter tip, and an inflatable balloon near the distal tip. The catheter was introduced transnasally by a gastroenterologist. Under intermittent fluoroscopic control, when the distal tip passed the ligament of Treitz, the balloon was inflated with 10 ml of air to enhance catheter progression by peristalsis to the distal ileum. Appr. 3–5 hours afterward, the position of the cathether was checked by fluoroscopy. When the catheter was successfully positioned (catheter tip in the mid- to distal region of the ileum, according to the gastroenterologist), the balloon was deflated. The small intestinal luminal content was collected from side-holes of the catheter, i.e., duodenum (side-hole 125 cm proximal to the distal catheter tip, jejunum (side-hole 65 cm proximal to the distal catheter tip) and proximal ileum (side-hole 5 cm proximal to the distal catheter tip). Six days prior to the start of the intervention (day −6) the luminal content was sampled in the morning, after an overnight fast. At day 14, the luminal content was sampled from the same locations at three time points, i.e. in the morning after overnight fast, around noon (i.e. approximately 2 hours after the supplements intake), and around 16:00, i.e. 3 hours after a standardized lunch (noodles). At the same day as luminal content collection, single fecal samples were collected at day −6 and 14. All samples were snap frozen and stored at −80 °C
Microbiota composition analysis
Microbiota composition was determined with barcoded 16S ribosomal RNA (rRNA) gene amplicon sequencing (Illumina Hiseq2500 (2 × 150 bp)). The intestinal fluid (≤1 ml) was immediately transferred to a screw cap tube containing 0.25 g of 0.1 mm zirconia beads and 3 glass beads (diameter 2.5 mm) to which 300 µl Stool Transport and Recovery (STAR) buffer (Roche Diagnostics, United States) was added. The mix was subjected to repeated bead beating (5.5 ms 3 × 60 s), followed by 15 min heating at 95°C at 1000 rpm. The lysate was then centrifuged for 5 min at 4°C (14000 g). The supernatant was collected and stored in a separate tube. The pellet was subjected to another cycle of cell disruption with 200 µl of STAR buffer. Supernatants from both cycles were pooled. Two times 250 µl of the supernatant was purified using a Maxwell extraction instrument (Promega, United States) with a Maxwell® 16Tissue LEV Total RNA purification Kit Cartridge customized for DNA extraction (XAS1220) and eluted in 40 µl of nuclease free water. For fecal material, 0.25 g feces were transferred to a screw cap tube containing 0.5 g of 0.1 mm zirconia beads and 5 glass beads (diameter 2.5 mm) to which 700 µl STAR buffer was added. Subsequent procedures were the same as described above for small intestinal samples, except for the second-round of cell disruption that used 300 µl STAR buffer and only one time 250 µl supernatant being used for purification. Obtained total DNA was then diluted to 20 ng/µl before amplification.
The V4 region of the 16S rRNA gene was amplified in triplicate using primers 515FCitation25 and 806 RCitation26 and extracted DNA as template. The amplification program was as described previously,Citation27 but with an annealing temperature of 50°C. Purified PCR products were mixed in equimolar amounts and sent for sequencing (Eurofins Genomics, Konstanz, Germany). Throughout the process, mock communities (i.e. mix of 16S rRNA gene sequences of known composition), biological replicates of random samples as well as no-template controls were included for quality control. Raw sequence reads were processed using the NG-Tax 1.0 pipeline using default settings.Citation28 Taxonomy assignment was based on the SILVA database (version 138).Citation29,Citation30
Metabolomic analysis
Small intestinal fluids collected with empty stomach and feces were subjected to metabolomic analysis. Specifically, for each sample, 100 μl of intestinal fluids were mixed with 200 μl of phosphate buffered saline that consists of 1.9 mM Na2HPO4, 8.1 mM NaH2PO4, 150 mM NaCl and 1 mM TSP (sodium 3-(trimethylsilyl)-propionate-d4) in D2O. After centrifugation (17000 g, 10 min), 300 µl of supernatant was transferred to a 3 mm NMR tube for analysis. Fecal extracts were prepared by mixing 20 mg of frozen fecal material with 1 ml of phosphate buffered saline mentioned above. After mixing thoroughly, samples were centrifuged (17000 g, 5 min). The supernatant was filtered through a 0.2 µm membrane filter and 300 µl of the filtrate was transferred to a 3 mm NMR tube for analysis. The centrifugation time for the different sample types was determined based on visual inspection and the fact that a clear supernatant (for good NMR spectra) was obtained.
Citation1H-NMR spectra were recorded using 600 MHz Bruker spectroscopy, and theCitation1H NMR spectra were automatically reduced to ASCII files using AMIX (v. 3.7; Bruker Biospin). Spectral intensities of1H NMR spectra were scaled to total intensity and reduced to integrated regions of equal width (0.04 ppm) corresponding to the region 10.00–0.2. The regions of
4.96–4.56 were excluded as it is remnant D2O signal.
Metatranscriptomic analysis
Luminal content from two subjects in the synbiotic group (S8, S10) and one subject in the control group (P8) were used for metatranscriptomic analysis. Due to difficulties in sample collection and amount of samples used to study the microbial composition, we unfortunately had sufficient amounts of material left to be used for metatranscriptome analysis for these three subjects, allowing for exploratory analyses only. RNA from small intestinal fluid was isolated as described earlier,Citation31 with minor modifications. Briefly, about 1 ml intestinal fluid was equally split into four bead beating tubes (containing 0.8 g 0.1 mm zirconia beads each), and mixed with 500 µl ice-old 3×TE buffer, 0.18 g macaloid suspension, 50 µl 10% SDS and 500 µl acid phenol (4°C; pH 4.5), followed by repeated bead beating (5.5 m/s 3 × 45 s) with 90 s break in between on ice and centrifugation at 13,400 g 4°C for 15 min. The obtained aqueous phase (in a new tube) was mixed with 250 µl ice-old acid phenol and 250 µl chloroform:isoamylalcohol (24:1) by vortexing and centrifuged at 13,400 g at 4°C for 5 min. The obtained aqueous phase (in a new tube) was then mixed with 500 µl chloroform:isoamylalcohol (24:1), followed by vigorously shaking and centrifugation at 13,400 g at 4°C for 5 min. The supernatant was mixed with 1/10 volume 3 M NaAc (pH 5.2) and 3 volumes of 95% ethanol of −20°C, and stored at −80°C overnight. Afterwards, they were rehydrated by centrifugation at 13,400 g at 4°C for 15 min. The pellet was washed with 70% ethanol of −20°C, followed by centrifugation at 13,400 g at 4°C for 5 min, dried at room temperature for 15 min, and resuspended in 100 µl 1×TE buffer, followed by DNAse digestion using RNeasy mini kit (Qiagen Inc.) according to manufacturer’s protocol. Obtained RNA was sent to Novogene (Cambridge, United Kingdom), where samples were further processed to remove rRNA using the Ribo-Zero rRNA Removal Kit (California, USA), to synthesize cDNA and double-stranded cDNA library, followed by paired-end sequencing on an Illumina NovaSeq instrument (150bp). The sequence data was trimmed with TrimmomaticCitation32 and potential host transcripts were tested against human genome database with bowtie2Citation33 before merged pair-end reads with PEAR.Citation34 The remaining ribosomal reads were removed with SortMeRNACitation35 by default settings. Functional profiling of remaining reads (i.e. the mRNA) was performed using default settings of HUMAnN2Citation36 with the full ChocoPhlAn,Citation37 UniRef 90Citation38 and MetaCyc databases.Citation39
Statistical analysis
The 16S rRNA gene sequence read counts were normalized to relative abundance. The microbial alpha diversity indices (Faith’s phylogenetic diversity, Inverse Simpson and Pielou index) were calculated based on amplicon sequence variants (ASVs). Normality of the data was checked by Shapiro-Wilk test. As data were not normally distributed in the current study, the Friedman test and pairwise Wilcoxon signed-rank tests were used for paired measurements. At baseline, the number of samples per sample type, i.e. feces, jejunum, duodenum and proximal ileum, was unequal, due to unsuccessful sample collection and/or DNA isolation. Therefore, the pairwise Mann-Whitney U test was used as an alternative. Correlation analysis (Spearman) was conducted using the rcorr function in the Hmisc package.Citation40 Significant differences between groups were tested by permutational multivariate analysis of variance (PERMANOVA) based on pairwise weighted and unweighted UniFrac distances at ASV level. Intervention effects on individual genus-level taxa were tested using a linear mixed effect model, with subject as random factor, and time and treatment as fixed factors. For metabolomic analysis, orthogonal partial least squares-discriminant analysis (OPLS-DA) was performed and validated in SIMCA-P+ (Umetrics, Umeå, Sweden). OPLS-DA model was validated using the CV-ANOVA method in SIMCA-P+ software. p values were corrected for multiple testing by the false discovery rate (FDR) using the Benjamini-Hochberg procedure. Corrected p values (referred to as q values) < 0.05 were considered to indicate significance. All statistical analyses were conducted in R (R-3.5.0).
Results
Gradual change in microbial and metabolomic composition from upper, mid, to lower sections of the intestine
We first set out to assess spatial gradients in microbial and metabolomic composition based on baseline data obtained 6 days prior to the start of the intervention. PERMANOVA based on weighted UniFrac (considering relative abundance of ASVs and their position in the phylogenetic tree) and unweighted UniFrac (considering only presence or absence of ASVs and their phylogenetic position) distance matrices, revealed significant (all q < 0.05) differences between the microbiota of feces and that of small intestinal fluids at baseline, i.e., day −6 of the trial (). Within the small intestine, principal coordinate analysis (PCoA) based on weighted and unweighted UniFrac distance matrices indicated significant differences in microbial composition between duodenum and jejunum (q=0.0036 and q=0.0024), between jejunum and proximal ileum (q=0.0140 and q=0.0060), as well as between duodenum and proximal ileum (both q=0.0015) (). Overall, sample site explained 66.7% (based on weighted UniFrac distance) or 55.0% (based on unweighted UniFrac distance) of the observed variation in microbial composition. Observed variation in microbial composition within each sample site was not significantly explained by recorded subject characteristics (i.e. age, sex and BMI).
Figure 1. Gradual change in microbial composition from upper, to mid, to lower sections of the intestine at baseline at day − 6 of the trial. PCoA of microbial composition based on (a) weighted or (b) unweighted UniFrac distance matrices. Significant differences between samples based on pairwise weighted and unweighted UniFrac distances at ASV level were tested by PERMANOVA. The percentage of variation in microbial composition explained by the two principal coordinates is shown at the axes. (b) Microbial diversity and (d) phylogenetically weighted richness. (e) Differences in microbial composition between different sample types within each individual. Distance was calculated based on weighted UniFrac distance. Scale ranges from 0 to 1, with lower values indicating higher similarity. Comparison of distances between sample types was done using a linear mixed effect model with subject as random intercept. Numbers of samples per subject vary due to technical reasons (for details, please refer to table S2). * indicates significant differences (q < 0.05) between groups.

Microbial diversity (Inverse Simpson) changed gradually along the small intestine (). The fecal microbial diversity was significantly higher than that of jejunum (q=0.0029) and proximal ileum (q=0.0001). The fecal microbial richness was higher than that of jejunum (q=0.0124) and proximal ileum (q<0.0001), but not significantly different from that of duodenum (). Within the small intestine, no significant differences were found in microbial diversity between duodenum and jejunum, but the microbial diversity of duodenum and jejunum was significantly higher than that of ileum (q=0.0021 and q=0.0457, respectively). Differences between small intestinal locations were more pronounced with respect to phylogenetic richness (), with significant differences between duodenum and jejunum (q=0.0039), jejunum and proximal ileum (q=0.0030), as well as between duodenum and proximal ileum (q<0.0001). Moreover, in line with findings in microbial diversity, weighted UniFrac distance between the microbiota of feces and that of small intestinal fluids was significantly (all q<0.05) larger than that among small intestinal samples ().
Zooming into the microbial composition, in addition to a large inter-individual variation, at family level the fecal microbiota was in general predominated by members of Lachnospiraceae and Ruminococcaceae. In some subjects, however, we observed high relative abundance of Bifidobacteriaceae, and few subjects showed high relative abundance of Prevotellaceae (). Concurrently, Streptococcaceae predominated the duodenal and jejunal microbiota, with some subjects having high relative abundance of Prevotellaceae in duodenum, and Veillonellaceae, Pasteurellaceae or Enterobacteriaceae in jejunum and/or ileum. Furthermore, at the genus level, the relative abundance of eight out of 197 observed taxa was significantly (all q<0.05) higher in luminal duodenal microbiota compared to proximal ileum (). The relative abundance of four of these eight genus-level taxa, namely Prevotella, Prevotella 7, Porphyromonas and Fusobacterium, was also significantly (all q<0.05) higher in the duodenal microbiota comparing to that of jejunum (). In contrast, no significant differences were observed in the relative abundance of individual taxa between jejunum and proximal ileum. Collectively, these data indicate a gradual change in microbial composition along the small intestine from upper, mid to lower sections, being in line with the biogeographical and physiological distances along the intestinal tract.Citation41
Figure 2. Microbial composition in different sample types at baseline at day − 6 of the trial. (a) Relative abundance of most predominant bacterial families (top 12, ranked base on the average relative abundance across the entire dataset). Top 12 microbial families are listed in the legend. Other families are summarized as “other”. Each column represents a given type of sample from one subject. (b) Heatmap of microbial genus-level taxa that significantly differed between samples taken at different regions along the small intestine. Color in the heatmap reflects the relative abundance normalized per taxon, with blue color indicating lower than average relative abundance and red color indicating higher than average relative abundance. Scaling of colors in the heatmap was done by subtracting the overall mean of a given genus across the entire dataset from its value for a specific sample and dividing by the standard deviation of the given genus. Numbers of samples per subject vary due to technical reasons (for details, please refer to table S2).

OPLS-DA was applied to visualize the differences in the metabolome of intestinal fluids (). The fecal metabolome was well separated (all q<0.05) from that observed in small intestinal content (). OPLS-DA without fecal samples revealed that the duodenal metabolome was well separated (all q<0.05) from that of jejunum and ileum, whereas the difference between the metabolome of jejunum and proximal ileum was not significant (). When evaluating individual metabolites, concentrations of uracil, adenine, ethanol and formate were found to be higher in feces compared to small intestinal fluids. Metabolomes in small intestinal fluids were less diverse than in feces and consisted mostly of amino acids (phenylalanine, tyrosine, threonine, alanine, valine, isoleucine), carbohydrates (mainly glucose), amines (choline) and glycerol. In addition, duodenum fluids had lower levels of glucose compared to all of the other parts.
Figure 3. Metabolomic composition in different types of samples and their correlation coefficient with microbial composition. (a) Orthogonal partial least squares-discriminant analysis (OPLS-DA) of metabolomic data collected at the different sites. (b) OPLS-DA of metabolomic data in small intestinal fluids. Numbers of samples per subject vary due to technical reasons. Specifically, we failed in collection of one duodenum sample (for details, please refer to table S2). (c) Correlation analysis between the microbial and metabolomic composition. Color in the heatmap reflects Spearman correlation coefficients, with blue color indicating negative correlation and red color indicating positive correlation. Hereby only significant correlations with absolute correlation coefficients above 0.7 or below − 0.7 are included.

Correlation analysis between relative abundances of observed genus-level taxa and metabolites, revealed significant correlations between microbial and metabolomic compositions with Spearman correlation coefficients ranging from −0.77 to 0.98 (Figure S2). Among those, the relative abundance of 37 and 9 out of the 197 observed genus-level taxa positively or negatively correlated with metabolomic data with absolute Spearman correlation coefficients bigger than 0.70 (). The relative abundance of three genus-level taxa (i.e., Dorea, Subdoligranulum and Lachnospiraceae NK4A136 group) correlated with the amount of different metabolomic compounds, both positively and negatively.
Re-assessing microbial community composition along the small intestine at different time points
Microbial composition along the small intestine was reevaluated 20 days after the first evaluation (baseline: at day −6). Consistent with findings reported for baseline measurements, small intestinal samples collected with empty stomach, at noon before lunch and three hours after lunch showed the same trend with respect to microbial diversity and richness, i.e. being highest in duodenum followed by jejunum then proximal ileum (Figure S3). Nevertheless, within the small intestine, differences in microbial diversity and richness were only significant between the microbiota of duodenum and proximal ileum (all q < 0.05) and between duodenum and jejunum (all q < 0.05). No significant difference was detected between the microbiota of jejunum and proximal ileum.
PERMANOVA based on unweighted UniFrac distance matrices revealed significant differences between the different small intestinal segments after overnight fasting, whereas at noon and three hours after lunch, no significant difference was measurable between the different small intestinal segments. When using weighted UniFrac distance matrices, differences were only found significant (q = 0.0091) between the microbiota of duodenum and proximal ileum after overnight fasting (Figure S4).
Dynamics of small intestinal microbiota within a day and over 20 days
In terms of microbial diversity () and richness (), no significant changes were observed in time (hours within a day and over 20 days). PERMANOVA revealed that microbiota composition was only significantly different (p = .009) between jejunum microbiota at noon and three hours after lunch based on weighted UniFrac distance matrix. Except that, no significant changes were identified in microbiota composition within a day (), and over 20 days. Although overall, weighted UniFrac distances between the small intestinal microbiota at different time points showed no significant difference (), visual inspection of microbiota composition demonstrated remarkable intra-individual dynamics within a day and over 20 days ().
Figure 4. Longitudinal changes in small intestinal microbial composition within a day and over 20 days, based on subjects included in the placebo control group. Changes in microbial diversity of duodenum (a), jejunum (b) and proximal ileum (c) over time in individual subjects. Changes in microbial richness of duodenum (d), jejunum (e) and proximal ileum (f) over time in individual subjects. PCoA of microbial composition based on weighted UniFrac distance matrices of duodenum (g), jejunum (h) and proximal ileum (i). Percentages at the axes indicated the percentage of variation explained. Weighted UniFrac distances between samples collected at different timepoints in terms of duodenum (j), jejunum (k) and proximal ileum (l). (m) Relative abundance of different bacterial families (top 12, ranked base on the average relative abundance across the entire dataset, see legend for taxa) in different sample types. Each column represents a given sample from one subject at a specific timepoint. Missing columns are due to technical issues (for details, please refer to table S2). Subject ID was re-coded to adhere to privacy regulations.

Changes in microbial and metabolomic composition after synbiotic supplementation
Comparative analysis before and after the synbiotic intervention, corrected using data obtained for the placebo control group, revealed neither significant changes in overall microbial composition in duodenum, jejunum, proximal ileum or feces ( and Figure S5a-d), nor in the relative abundance of individual taxa. Microbial diversity in the jejunum decreased significantly (q = 0.0206) after synbiotic supplementation (), attributing to the significant decrease in microbial evenness (q = 0.0072, ). In contrast, neither the microbial diversity in duodenum, proximal ileum or feces (), nor phylogenetically weighted richness in duodenum, jejunum, proximal ileum and feces were affected significantly after synbiotic supplementation (). As such, the lower microbial diversity in jejunum can be attributed to the decrease in microbial evenness in the jejunum. Both in the placebo control group and in the synbiotic group, weighted UniFrac distance between samples taken at day −6 and day 14 was significantly (p = .0043 and p = .0407) higher in proximal ileum than that in feces (), indicating ileal microbiota had lower stability than fecal microbiota. Moreover, it is worth mentioning that small intestinal response to the synbiotic supplementation was highly personalized.
Figure 5. Comparison of microbial composition before and after the intervention (placebo control, synbiotic). PCoA of microbial composition pre vs post intervention in (a) duodenum, (b) jejunum, (c) proximal ileum and (d) feces, as well as corresponding changes in microbial diversity in (e) duodenum, (f) jejunum, (g) proximal ileum and (h) feces. (i) Weighted UniFrac distances between samples collected at day − 6 and day 14. PCoA is based on weighted UniFrac distances. Values are presented in scatter plots and linked per individual. Small intestinal samples were collected after overnight fasting. Pre: before Intervention; post: 14 days after start of the intervention. Percentages at the axes indicated the percentage of variation explained. Comparative analysis between timepoints within each sample type and supplementation group (e.g. duodenum pre synbiotic vs duodenum post synbiotic), was performed with PERMANOVA. Within each sample type, intervention effects on the microbial diversity were compared with variance components (random intercept) linear mixed models and correction for baseline values. Sample sizes vary due to technical reasons (for details, please refer to table S2).

Figure 6. OPLS-DA of metabolomes pre vs post intervention in (a) duodenum, (b) jejunum, (c) proximal ileum and (d) feces. Sample sizes vary due to technical reasons.Specifically, one duodenum sample (post intervention) failed in obtaining NMR data. In addition, we failed in the sample collection of jejunum (n = 2) and ileum (n = 3) samples after over night fasting (for details, please refer to table S2).

No significant intervention impact was identified on the metabolomic data of duodenum, jejunum, ileum or feces (). This result was line with findings of the microbiota data and highlighted the strong correlation between microbiota and metabolome data ().
Kinetics of ingested microbes throughout the intervention period
Bacterial strains included in the synbiotic belong to Lactococcus, Bifidobacterium, Lactobacillus, Lacticaseibacillus, Lactiplantibacillus and Ligilactobacillus genera. At day 14 (, white area), four subjects in the synbiotic group showed a strong increase in the relative abundance of the genera included in the synbiotic formulation, in the duodenum (p values corrected and calculated for all participants, all p > .05), jejunum (all Q > 0.05) and proximal ileum (Lactococcus q=0.08, Lacticaseibacillus q=0.08, Lactiplantibacillus q=0.15, Bifidobacterium q=0.28) about two hours after ingestion of the synbiotic. However, these levels returned to baseline within three hours (). Concurrently, subjects in the placebo control group did not show any significant alteration in the relative abundance of these genera, neither after two weeks of supplementation, nor during day 14.
Figure 7. Kinetics of ingested bacteria. Relative abundance of (a) lactococcus, (b) bifidobacterium, (c) lactobacillus, (d) lacticaseibacillus, (e) lactiplantibacillus and (f) ligilactobacillus before and after supplementation of placebo (control) or synbiotic.Samples are linked per individual. Faeces was collected at the same day as small intestinal samples. Day − 6 morning and Day 14 morning: samples were collected with empty stomach. Day 14 before lunch: samples were collected about 2 hours after last ingestion. Day 14 afternoon: samples were collected about three hours after standardized lunch (noodles). Sample sizes vary due to technical reasons (for details, please refer to table S2).

A comparison of samples taken in the morning at baseline (day −6) and day 14 showed that 14 days of supplementation did not significantly affect the relative abundance of Lactococcus, Bifidobacterium, Lactobacillus, Lacticaseibacillus, Lactiplantibacillus or Ligilactobacillus in duodenum, jejunum, proximal ileum or feces when considering all subjects ( beige background), albeit with inter-individual differences with respect to the observed variation at different locations. After synbiotic supplementation, one subject (subject S8) had increased levels of Bifidobacterium in jejunum. Another subject (subject S3) had increased relative abundance of Lactobacillus and Lactiplantibacillus in jejunum. Another subject (subject S10) showed an increased level of Ligilactobacillus. However, the relative abundance of these genera decreased again during the last intervention day ( white background). This can likely be attributed to the transient presence of bacterial DNA from the synbiotic strains in the small intestine.
At ASV level, sequences corresponding to ingested probiotic species were observed in the 16S rRNA gene sequence data (). Specifically, the changes in the relative abundance of ASVs classified as Lactococcus lactis and Bifidobacterium were in line with the observations at genus level, i.e. increased relative abundance about two hours after ingestion and rapid decrease afterward (). Moreover, we observed ASVs corresponding to all Lactobacillus, Lacticaseibacillus, Lactiplantibacillus and Ligilactobacillus species in the probiotic mixture, including L. plantarum, L. acidophilus, L. salivarius, L. paracasei and L. casei. The relative abundance of these Lacticaseibacillus and Ligilactobacillus species was highest about two hours after the ingestion at day 14, coinciding with the observation at genus level (). It should be noted, however, that the inherent limitations with respect to species-level resolution in V4 sequence data did not in all cases allow for unequivocal assignment of ASVs to specific species (). Similarly, for Bifidobacterium, observed sequences corresponded to B. bifidum, while B. lactis was classified as B. longum/B. lactis, with an increase in relative abundance after synbiotic ingestion in all sample types. These data strongly suggest the transient passage of bacterial DNA from the ingested probiotic strains.
With the increase in the relative abundance of ingested probiotic strains in the small intestine about two hours after consumption of supplements, we investigated the microbial activity while the ingested microbes were passing through the small intestine by metatranscriptomic analysis, albeit only in a small number of subjects, owing to the limited availability of sufficient material. The microbial activity of small intestinal samples from two subjects (subject S8 and S10 in the synbiotic group) with large increase in the relative abundance of probiotic strains about two hours after the last supplementation was compared with that of one subject (subject P8) in the placebo control group. Despite the technical challenges due to the low biomass in these small intestinal samples (from about 1 ml intestinal fluid) and subsequent background noise, we obtained 1.89 × 10Citation5 to 1.38 × 10Citation7 mRNA reads per sample. Aside from the expression of housekeeping functions, like nucleotide biosynthesis pathways, glycolysis pathways and amino acid biosynthesis pathways etc, we observed considerable variation between the metatranscriptomes. However, we did not observe enrichment of metatranscriptomic reads that were specifically assigned to taxa corresponding to the probiotic strains in the samples taken from subjects that ingested the synbiotic formulation.
Discussion
In the current study, we investigated the spatio-temporal dynamics of the small intestinal microbiome within a day and over 20 days, as well as its responses to a 14-day synbiotic supplementation in 20 healthy subjects. We hypothesized that microbiota composition differs per sampling site and that these location-specific microbial communities respond differently to the synbiotic supplementation. We found significant differences between the microbiota and metabolome of duodenum, jejunum, proximal ileum content and feces. The significant difference along the small intestine was most pronounced in the morning after overnight fasting, whereas it became not always measurable around noon or in the afternoon. Over 20 days and over a few hours within a day, the small intestinal microbiota changed remarkably per individual, while this change demonstrated great heterogeneity between subjects. In addition, ingestion of probiotic bacteria induced a short-term spike in the relative abundance of corresponding genera approximately two hours after ingestion in some individuals. These ingested microbes were not active as based on metatranscriptome data, although it should be noted that this could only be measured in a small fraction (2 out of 10) of our study group, suggesting their passage through the small intestine. Technical limitations of this analysis (i.e. low biomass, time required for sample aspiration) however has to be considered. Except for decrease in jejunum microbial evenness, two weeks of synbiotic supplementation did not significantly affect the microbial and metabolomic composition in duodenum, jejunum, proximal ileum or feces. Nevertheless, their reponse to the supplemented synbiotic was highly personalized.
Although some subjects had high relative abundance of Veilonellaceae, Enterobacteriaceae or Pasteurellaceae, the small intestinal microbiota was generally predominated by Streptococcaceae, as found previously in ileostoma effluent from ileostomists and small intestinal fluid from healthy volunteers.Citation11,Citation12,Citation16,Citation42 Consistent with earlier studies,Citation2 the spatial and physiological differences between upper, mid and lower regions of the small intestine and feces, were reflected in their microbial and metabolomic composition. Differences between different small intestinal microbial communities were in line with the corresponding physical distance between sampling sites, attributing to the gradual change in physiological conditions along the intestine, like pH and concentrations of oxygen and nutrients.Citation43 This could also explain, in part, the higher relative abundance of several taxa that are known members of oral microbiota (like Leptotrichia, Campylobacter, Prevotella, Selenomonas, Fusobacterium, Porphyromonas and Alloprevotella) in duodenum compared to proximal ileum ,Citation44–47
Comparison of1H-NMR spectra showed that fecal metabolomes were more complex and diverse than those of small intestinal fluids. In most fecal samples, SCFAs such as acetate, propionate and butyrate were dominant metabolites.Citation48 Besides SCFAs, concentrations of uracil, adenine, ethanol and formate were higher in feces compared to intestinal fluids. Metabolomes in small intestinal fluids were less diverse and consisted mostly of amino acids (phenylalanine, tyrosine, threonine, alanine, valine, isoleucine), carbohydrates (mainly glucose), amines (choline) and glycerol. This reflects the main functions of the small intestine, which is digestion and absorption of nutrients from our diet. The most prominent difference in duodenum fluids compared to that of the other parts of the small intestine was a lower level of glucose.
In contrast to previously reported increasing microbial diversity from the stomach to the colon,Citation49 comparative analyses in the current study showed that the microbial diversity and richness decreased from upper to lower sections of the small intestine. Early on, researchers investigated the small intestinal microbiota based on specimens of recently deceased subjects, which are often hard to obtain and are small in sample size, and doubts have been raised with respect to these samples being representative for the in vivo situation.Citation50 Wang et al., compared the microbial diversity of jejunum and distal ileum in a single subject, and revealed lower microbial diversity in jejunum as compared to distal ileum.Citation10 Recently Seekatz et al. used a customized multichannel catheter and collected luminal content of duodenum and the proximal, mid- and distal jejunum from eight healthy subjects, demonstrating higher microbial diversity in duodenum compared to proximal and mid jejunum,Citation42 being in line with findings of the current study. Collectively, comparing to available studies, the current study is the largest study (based on 20 subjects) to date investigating spatio-temporal variation in small intestinal microbiota composition. In addition, we used a multichannel aspiration catheter which enabled comparative analysis among different sites of the small intestine within the same subject, therefore allowing to account for subject specificity, including subject- and location-specific responsiveness to a given intervention, such as the synbiotic supplement that was used here.
Many strains included in the supplemented synbiotic are known for their tolerance/resistance to low pH and bile,Citation51 as well as adhesion to mucus, epithelial cells or enterocyte-like Caco-2 cells,Citation52–54 Compared to single strain probiotics, multispecies probiotic mixtures are suggested to have potential advantages to convey additive or synergistic effects and exhibit better efficacy in health-related outcomes.Citation55 Two weeks of synbiotic supplementation of the healthy population in the current study did not alter microbial and metabolomic composition in the lumen of the small intestine and feces, except for a decrease of microbial evenness in jejunum. The cause and implication of the observed temporally decreased microbial evenness on health-related parameters in the jejunum remains to be determined. Nevertheless, Maxwell®825 was developed and studied in subjects with increased risk or even already developed chronic gastrointestinal disorders (e.g. IBD/IBS) and (low-grade) inflammatory conditions.Citation22,Citation56 Additionally, the response of intestinal microbiota in healthy subjects could differ from that of unhealthy subjects.
Findings of the current study are in line with those described earlier in which eight weeks of Maxwell®825 intervention did not affect the diversity of mucosal pouch microbiota in patients with active pouchitis.Citation22 In another study, Moser and colleagues showed that supplementation with the probiotic mixture OMNi-Ecologic® Stress Repair, containing the same strains as Ecologic®825, increased the microbial diversity in the upper gastrointestinal tract (i.e. gastric corpus and duodenum mucosal specimen), but not in the lower intestinal tract (ascending colon biopsies or feces), of IBS-D patients.Citation56 These authors found a significant increase in the relative abundance of unclassified Lactobacillaceae, and a decrease in relative abundance of Moraxella and Moryella in feces. In the current study, no significant effects of two weeks intervention with respect to the relative abundance of bacterial groups were observed, including genera included in the synbiotic. It should be noted, however, that comparability of both studies is limited. Differences between the current study and the study of Moser and colleaguesCitation56 with respect to target group (healthy subjects vs. IBS-D patients), sampling sites (lumen vs mucosal specimen), dosage of the probiotic (1.5 × 1010 CFU vs 6.75 × 1010 CFU) and prebiotic (10 g/day FOS P6 vs. inclusion in the carrier material only), and duration (two vs. four weeks) might at least in part have contributed to the observed differences in microbial data between studies.Citation57,Citation58 To this end, it is of interest to note that a number of studies reported reduced mucosal and/or fecal microbial diversity and richness in IBS patients vs healthy controls,Citation59–61 and thus, the more diverse communities in healthy individuals might be more resilient, and thus less amenable to change. In part, this might also contribute to explaining the previously observed lack of effects on intestinal permeability both under basal and under indomethacin-induced stressed conditions, immune function or intestinal symptoms in subjects receiving this synbiotic supplement.Citation24 In addition, the current study illustrated the passage of ingested probiotic microbes through the intestine, while cultivation of specified microbes is required to uneuivacally confirm their survival.
The metabolomes observed before and after synbiotic supplementation in the present study also showed a similar phenomenon. Two weeks intervention of synbiotic supplementation did not alter metabolomes in feces and in small intestinal fluids, the latter being in line with the observed limited influence on the microbiota composition in the small intestine. Fecal metabolites are the physiological product of microbial activity in the gut and therefore, it can likely be assumed that the fecal metabolome should reflect the current gut metabolic status and microbial activities.Citation62 Some studies showed that the luminal microbiota is altered during probiotic or synbiotic treatment and therefore leads to alterations in metabolic activity.Citation63 However, those studies were mostly based on long term intervention periods (3–6 months) and/or under different health conditions. Interestingly, a recent study showed activity of a probiotic strain in ileostomy effluent samples, which contrasts to the observations in the current study.Citation64 Whether differences in observations are due to site of sampling (effluent vs jejunum/ileum lumen), strain-specificity, or diminished probiotic strains’ survival, remains to be determined.
Consistent with earlier observations that small intestinal microbiota is highly dynamic,Citation2 we also observed fluctuations in small intestinal microbial composition within one day. In the synbiotic group, the fluctuation of ingested microbes’ relative abundance in the small intestine, albeit not in all subjects, could be partly attributed to individual differences in transit timeCitation65 and differences in baseline microbiota composition (). Up to now, although studies investigating the probiotic effect on mucosal transcriptomic responses exist, the short-term effect of synbiotic supplementation on small intestinal microbiota has not been addressed before. The implications of the observed elevated levels of probiotic strains approximately two hours after the ingestion on host gene expression remain to be addressed in follow-up studies.
Considering limitations of the current study, our metatranscriptome analysis was based on two subjects in the synbiotic group and one suject in the placebo group, only allowing for initial exploratory analyses. Although no metabolic activities of ingested probiotic strains were identified based on obtained metatransptome data, the conclusion on the activity of probiotic strains is unavoidably limited by the small sample-size used for the analysis. Another limitation of the current study was the use of healthy subjects, whose baseline microbiota might differ from that of unhealthy subjects also with respect to the small intestine. The intestinal microbiota response of unhealthy subjects might therefore also differ from that of healthy subjects.
Overall, the current study investigated the spatio-temporal dynamics of the small intestinal microbiome in healthy subjects, as well as its response to synbiotic supplementation, with a dedicated control panel of healthy individuals receiving placebo intervention. We demonstrated significant differences in microbial and metabolomic composition at baseline between duodenum, jejunum, proximal ileum content and feces. In addition, the significant difference in microbial composition along the small intestine was most pronounced in the morning after overnight fasting, while it was not always measurable around noon or in the afternoon. Two weeks of synbiotic supplementation did not affect the overall microbiota and metabolomic composition in small intestinal fluids and feces differently from placebo. Moreover, small intestinal microbiota is highly dynamic, and ingested probiotic bacteria were shown to lead to a transient spike in the relative abundance of corresponding genera and ASVs, suggesting their passage through the entire gastrointestinal tract.
Author contributions
Author contributions were as follows: Conceptualization, H.S., F.J.T., J.G., I.B.V., A.A.M, W.M.D.V. and G.T.R.; Formal analysis, R.A.; Funding acquisition, H.S., W.M.D.V., I.B.V., A.A.M, G.T.R. and F.J.T.; Investigation, R.A., J.G., E.W. H.K.K and C.S.P.; Methodology, J.G., E.W. H.K.K and F.J.T.; Resources, F.J.T. and H.S.; Supervision, H.S., D.M.A.E.J., E.G.Z., G.T.R., W.M.D.V. and F.J.T.; Writing original draft, R.A.
Supplemental Material
Download Zip (3.3 MB)Acknowledgments
This work was funded by Rijksdienst voor Ondernemend Nederland (FND-07013), and a consortium agreement was signed among Wageningen University & Research, Maastricht University Medical Centre+ and Winclove Probiotics. Winclove Probiotics (Winclove BV, Amsterdam, the Netherlands) provided the investigational products. This study was also supported by the unlimited Spinoza Award to WMdV (NWO 2008).
Disclosure statement
J.G. and I.B.V. are employees of Winclove Probiotics. They have no direct or additional financial interests, and moreover this does not alter our adherence to policies on open and transparent sharing of data and materials. All other authors disclose no conflicts of interest.
Data availability statement
The raw sequence data of the microbiota composition has been deposited into European Nucleotide Archive with accession number PRJEB42262. The RNA-seq data generated within this study has been deposited into European Nucleotide Archive with accession number PRJEB55599.
Supplementary material
Supplemental data for this article can be accessed online at https://doi.org/10.1080/19490976.2024.2350173
Additional information
Funding
References
- Nicholson JK, Holmes E, Kinross J, Burcelin R, Gibson G, Jia W, Pettersson S. Host-gut microbiota metabolic interactions. Science. 2012;336(6086):1262–19. doi:10.1126/science.1223813.
- Donaldson GP, Lee SM, Mazmanian SK. Gut biogeography of the bacterial microbiota. Nat Rev Microbiol. 2016;14(1):20–32. doi:10.1038/nrmicro3552.
- Costello EK, Lauber CL, Hamady M, Fierer N, Gordon JI, Knight R. Bacterial community variation in human body habitats across space and time. Science. 2009;326(5960):1694–1697. doi:10.1126/science.1177486.
- Shalon D, Culver RN, Grembi JA, Folz J, Treit PV, Shi H, Rosenberger FA, Dethlefsen L, Meng X, Yaffe E. et al. Profiling the human intestinal environment under physiological conditions. Nature. 2023;617(7961):581–591. doi:10.1038/s41586-023-05989-7.
- El Aidy S, van den Bogert B, Kleerebezem M. The small intestine microbiota, nutritional modulation and relevance for health. Curr Opin Biotechnol. 2015;32:14–20. doi:10.1016/j.copbio.2014.09.005.
- van der Beek CM. The gut in control of health and disease: unraveling the role of short-chain fatty acids in human metabolism. Datawyse/Universitaire Pers Maastricht. 2017.
- Bevins CL, Salzman NH. Paneth cells, antimicrobial peptides and maintenance of intestinal homeostasis. Nat Rev Microbiol. 2011;9(5):356–368. doi:10.1038/nrmicro2546.
- Islam KS, Fukiya S, Hagio M, Fujii N, Ishizuka S, Ooka T, Ogura Y, Hayashi T, Yokota A. Bile acid is a host factor that regulates the composition of the cecal microbiota in rats. Gastroenterology. 2011;141(5):1773–1781. doi:10.1053/j.gastro.2011.07.046.
- Constante M, Libertucci J, Galipeau HJ, Szamosi JC, Rueda G, Miranda PM, Pinto-Sanchez MI, Southward CM, Rossi L, Fontes ME. et al. Biogeographic variation and functional pathways of the gut microbiota in Celiac disease. Gastroenterology. 2022;163(5):1351–1363.e15. doi:10.1053/j.gastro.2022.06.088.
- Wang M, Ahrne S, Jeppsson B, Molin G. Comparison of bacterial diversity along the human intestinal tract by direct cloning and sequencing of 16S rRNA genes. FEMS Microbiol Ecol. 2005;54(2):219–231. doi:10.1016/j.femsec.2005.03.012.
- Booijink CC, El-Aidy S, Rajilic-Stojanovic M, Heilig HGHJ, Troost FJ, Smidt H, Kleerebezem M, De Vos WM, Zoetendal EG. High temporal and inter-individual variation detected in the human ileal microbiota. Environ Microbiol. 2010;12(12):3213–3227. doi:10.1111/j.1462-2920.2010.02294.x.
- Zoetendal EG, Raes J, Van Den Bogert B, Arumugam M, Booijink CCGM, Troost FJ, Bork P, Wels M, de Vos WM, Kleerebezem M. et al. The human small intestinal microbiota is driven by rapid uptake and conversion of simple carbohydrates. Isme J. 2012;6(7):1415–1426. doi:10.1038/ismej.2011.212.
- van den Bogert B, Erkus O, Boekhorst J, de Goffau M, Smid EJ, Zoetendal EG, Kleerebezem M. Diversity of human small intestinal streptococcus and Veillonella populations. FEMS Microbiol Ecol. 2013;85(2):376–388. doi:10.1111/1574-6941.12127.
- Tang Q, Jin G, Wang G, Liu T, Liu X, Wang B, Cao H. Current sampling methods for gut microbiota: a call for more precise devices. Front Cell Infect Microbiol. 2020;10:151. doi:10.3389/fcimb.2020.00151.
- van Trijp MP, Wilms E, Ríos-Morales M, Masclee AA, Brummer RJ, Witteman BJ, Troost FJ, Hooiveld GJ. Using naso- and oro-intestinal catheters in physiological research for intestinal delivery and sampling in vivo: practical and technical aspects to be considered. The American Journal Of Clinical Nutrition. 2021;114(3):843–861. doi:10.1093/ajcn/nqab149.
- van Trijp MPH, Rios-Morales M, Witteman B, Abegaz F, Gerding A, An R, Koehorst M, Evers B, van Dongen KCV, Zoetendal EG. et al. Intraintestinal fermentation of fructo- and galacto-oligosaccharides and the fate of short-chain fatty acids in humans. iScience. 2024;27(3):109208. doi:10.1016/j.isci.2024.109208.
- Martinez-Guryn K, Hubert N, Frazier K, Urlass S, Musch MW, Ojeda P, Pierre JF, Miyoshi J, Sontag TJ, Cham CM. et al. Small intestine microbiota regulate host digestive and absorptive adaptive responses to dietary lipids. Cell Host Microbe. 2018;23(4):458–469.e5. doi:10.1016/j.chom.2018.03.011.
- Poteres E, Hubert N, Poludasu S, Brigando G, Moore J, Keeler K, Isabelli A, Ibay ICV, Alt L, Pytynia M. et al. Selective regional alteration of the gut microbiota by diet and antibiotics. Front Physiol. 2020;11:797. doi:10.3389/fphys.2020.00797.
- Angelakis E, Armougom F, Carrière F, Bachar D, Laugier R, Lagier J-C, Robert C, Michelle C, Henrissat B, Raoult D. et al. A metagenomic investigation of the duodenal microbiota reveals links with obesity. PLoS One. 2015;10(9):e0137784. doi:10.1371/journal.pone.0137784.
- Jensen BAH, Heyndrickx M, Jonkers D, Mackie A, Millet S, Naghibi M, Pærregaard SI, Pot B, Saulnier D, Sina C. et al. Small intestine vs. colon ecology and physiology: why it matters in probiotic administration. Cell Rep Med. 2023;4(9):101190. doi:10.1016/j.xcrm.2023.101190.
- Carlsson AH, Lutgendorff F, Akkermans L, McKay DM, Söderholm JD. Probiotics modulate mast cell degranulation and reduce stress-induced barrier dysfunction in vitro. 2013.
- Persborn M, Gerritsen J, Wallon C, Carlsson A, Akkermans LMA, Söderholm JD. The effects of probiotics on barrier function and mucosal pouch microbiota during maintenance treatment for severe pouchitis in patients with ulcerative colitis. Aliment Pharmacol Ther. 2013;38(7):772–783. doi:10.1111/apt.12451.
- González-Rodríguez I, Ruiz L, Gueimonde M, Margolles A, Sánchez B. Factors involved in the colonization and survival of bifidobacteria in the gastrointestinal tract. FEMS Microbiol Lett. 2013;340(1):1–10. doi:10.1111/1574-6968.12056.
- Wilms E, Gerritsen J, Smidt H, Besseling-van der Vaart I, Rijkers GT, Garcia Fuentes AR, Masclee AAM, Troost FJ. Effects of supplementation of the synbiotic ecologic® 825/FOS P6 on intestinal barrier function in healthy humans: a randomized controlled trial. PLoS One. 2016;11(12):e0167775. doi:10.1371/journal.pone.0167775.
- Parada AE, Needham DM, Fuhrman JA. Every base matters: assessing small subunit rRNA primers for marine microbiomes with mock communities, time series and global field samples. Environ Microbiol. 2016;18(5):1403–1414. doi:10.1111/1462-2920.13023.
- Apprill A, McNally S, Parsons R, Weber L. Minor revision to V4 region SSU rRNA 806R gene primer greatly increases detection of SAR11 bacterioplankton. Aquat Microbial Ecol. 2015;75(2):129–137. doi:10.3354/ame01753.
- An R, Wilms E, Smolinska A, Hermes GDA, Masclee AAM, de Vos P, Schols HA, van Schooten FJ, Smidt H, Jonkers DMAE. et al. Sugar beet pectin supplementation did not alter profiles of fecal microbiota and exhaled breath in healthy young adults and healthy elderly. Nutrients. 2019;11(9):2193. doi:10.3390/nu11092193.
- Ramiro-Garcia J, Hermes GD, Giatsis C, Sipkema D, Zoetendal EG, Schaap PJ, Smidt H. NG-Tax, a highly accurate and validated pipeline for analysis of 16S rRNA amplicons from complex biomes. F1000Research. 2016;5:1791. doi:10.12688/f1000research.9227.1.
- Quast C, Pruesse E, Yilmaz P, Gerken J, Schweer T, Yarza P, Peplies J, Glöckner FO. The SILVA ribosomal RNA gene database project: improved data processing and web-based tools. Nucleic Acids Res. 2013;41(D1):590–596. doi:10.1093/nar/gks1219.
- Yilmaz P, Parfrey LW, Yarza P, Gerken J, Pruesse E, Quast C, Schweer T, Peplies J, Ludwig W, Glöckner, FO. The SILVA and “all-species living tree project (LTP)” taxonomic frameworks. Nucleic Acids Res. 2014;42(D1):643–648.
- Zoetendal EG, Booijink CC, Klaassens ES, Heilig HG, Kleerebezem M, Smidt H, de Vos WM. Isolation of RNA from bacterial samples of the human gastrointestinal tract. Nat Protoc. 2006;1(2):954–959. doi:10.1038/nprot.2006.143.
- Bolger AM, Lohse M, Usadel B. Trimmomatic: a flexible trimmer for Illumina sequence data. Bioinformatics. 2014;30(15):2114–2120. doi:10.1093/bioinformatics/btu170.
- Langmead B, Wilks C, Antonescu V, Charles R. Scaling read aligners to hundreds of threads on general-purpose processors. Bioinformatics. 2018;35(3):421–432. doi:10.1093/bioinformatics/bty648.
- Zhang J, Kobert K, Flouri T, Stamatakis A. PEAR: a fast and accurate Illumina paired-end reAd mergeR. Bioinformatics. 2014;30(5):614–620. doi:10.1093/bioinformatics/btt593.
- Kopylova E, Noé L, Touzet H. SortMeRNA: fast and accurate filtering of ribosomal RNAs in metatranscriptomic data. Bioinformatics. 2012;28(24):3211–3217. doi:10.1093/bioinformatics/bts611.
- Franzosa EA, McIver LJ, Rahnavard G, Thompson LR, Schirmer M, Weingart G, Lipson KS, Knight R, Caporaso JG, Segata N. et al. Species-level functional profiling of metagenomes and metatranscriptomes. Nat Methods. 2018;15(11):962–968. doi:10.1038/s41592-018-0176-y.
- Truong DT, Franzosa EA, Tickle TL, Scholz M, Weingart G, Pasolli E, Tett A, Huttenhower C, Segata N. MetaPhlAn2 for enhanced metagenomic taxonomic profiling. Nat Methods. 2015;12(10):902–903. doi:10.1038/nmeth.3589.
- Suzek BE, Huang HZ, McGarvey P, Mazumder R, Wu CH. UniRef: comprehensive and non-redundant UniProt reference clusters. Bioinformatics. 2007;23(10):1282–1288. doi:10.1093/bioinformatics/btm098.
- Caspi R, Billington R, Keseler IM, Kothari A, Krummenacker M, Midford PE, Ong WK, Paley S, Subhraveti P, Karp PD. et al. The MetaCyc database of metabolic pathways and enzymes - a 2019 update. Nucleic Acids Res. 2020;48(D1):445–453. doi:10.1093/nar/gkz862.
- Harrell FE, Dupont C. Hmisc: Harrell miscellaneous. R package version 4.1-1. R Found Stat Comput. 2018.
- Volk N, Lacy B. Anatomy and physiology of the small bowel. Gastrointest Endosc Clin N Am. 2017;27(1):1–13. doi:10.1016/j.giec.2016.08.001.
- Seekatz AM, Schnizlein MK, Koenigsknecht MJ, Baker JR, Hasler WL, Bleske BE, Young VB, Sun D. Spatial and temporal analysis of the stomach and small-intestinal microbiota in fasted healthy humans. mSphere. 2019;4(2):4. doi:10.1128/mSphere.00126-19.
- Booijink CC, Zoetendal EG, Kleerebezem M, de Vos WM. Microbial communities in the human small intestine: coupling diversity to metagenomics. Future Microbiol. 2007;2(3):285–295. doi:10.2217/17460913.2.3.285.
- Brennan CA, Garrett WS. Fusobacterium nucleatum—symbiont, opportunist and oncobacterium. Nat Rev Microbiol. 2019;17(3):156–166. doi:10.1038/s41579-018-0129-6.
- Welch JLM, Rossetti BJ, Rieken CW, Dewhirst FE, Borisy GG. Biogeography of a human oral microbiome at the micron scale. Proc Natl Acad Sci USA. 2016;113(6):791–800. doi:10.1073/pnas.1522149113.
- Downes J, Dewhirst FE, Tanner AC, Wade, WG. Description of alloprevotella rava gen. nov. sp. nov. isolated from the human oral cavity, and reclassification of prevotella tannerae Moore. 1994 as alloprevotella tannerae gen. nov. comb. nov. Int J Syst Evol Microbiol. 2013;63:1214.
- Wang K, Lu W, Tu Q, Ge Y, He J, Zhou Y, Gou Y, Van Nostrand JD, Qin Y, Li J. et al. Preliminary analysis of salivary microbiome and their potential roles in oral lichen planus. Sci Rep. 2016;6(1):22943. doi:10.1038/srep22943.
- Le Gall G, Noor SO, Ridgway K, Scovell L, Jamieson C, Johnson IT, Colquhoun IJ, Kemsley EK, Narbad A. Metabolomics of fecal extracts detects altered metabolic activity of gut microbiota in ulcerative colitis and irritable bowel syndrome. J Proteome Res. 2011;10(9):4208–4218. doi:10.1021/pr2003598.
- Zoetendal EG, Rajilic-Stojanovic M, de Vos WM. High-throughput diversity and functionality analysis of the gastrointestinal tract microbiota. Gut. 2008;57(11):1605–1615. doi:10.1136/gut.2007.133603.
- Savage DC. Microbial ecology of the gastrointestinal tract. Annu Rev Microbiol. 1977;31(1):107–133. doi:10.1146/annurev.mi.31.100177.000543.
- Ruiz L, Margolles A, Sanchez B. Bile resistance mechanisms in lactobacillus and bifidobacterium. Front Microbiol. 2013;4:396. doi:10.3389/fmicb.2013.00396.
- Kirjavainen PV, Ouwehand AC, Isolauri E, Salminen SJ. The ability of probiotic bacteria to bind to human intestinal mucus. FEMS Microbiol Lett. 1998;167(2):185–189. doi:10.1111/j.1574-6968.1998.tb13226.x.
- Garcia-Gonzalez N, Prete R, Battista N, Corsetti A. Adhesion properties of food-associated lactobacillus plantarum strains on human intestinal epithelial cells and modulation of IL-8 release. Front Microbiol. 2018;9:2392. doi:10.3389/fmicb.2018.02392.
- Kimoto H, Kurisaki J, Tsuji N, Ohmomo S, Okamoto T. Lactococci as probiotic strains: adhesion to human enterocyte‐like Caco‐2 cells and tolerance to low pH and bile. Lett Appl Microbiol. 1999;29(5):313–316. doi:10.1046/j.1365-2672.1999.00627.x.
- Chapman C, Gibson GR, Rowland I. Health benefits of probiotics: are mixtures more effective than single strains? Eur J Nutr. 2011;50(1):1–17. doi:10.1007/s00394-010-0166-z.
- Moser AM, Spindelboeck W, Halwachs B, Strohmaier H, Kump P, Gorkiewicz G, Högenauer C. Effects of an oral synbiotic on the gastrointestinal immune system and microbiota in patients with diarrhea-predominant irritable bowel syndrome. Eur J Nutr. 2019;58:2767–2778. doi:10.1007/s00394-018-1826-7.
- Mora D, Filardi R, Arioli S, Boeren S, Aalvink S, de Vos WM. Development of omics‐based protocols for the microbiological characterization of multi‐strain formulations marketed as probiotics: the case of VSL# 3. Microb Biotechnol. 2019;12(6):1371–1386. doi:10.1111/1751-7915.13476.
- Conn VS, Chan KC. How much, how often, how long? Addressing dosage in intervention studies. Los Angeles, CA: SAGE Publications Sage CA; 2016.
- Mättö J, Maunuksela L, Kajander K, Palva A, Korpela R, Kassinen A, Saarela M. Composition and temporal stability of gastrointestinal microbiota in irritable bowel syndrome â?? a longitudinal study in IBS and control subjects. FEMS Immunol Med Microbiol. 2005;43(2):213–222. doi:10.1016/j.femsim.2004.08.009.
- Jeffery IB, O’toole PW, Öhman L, Claesson MJ, Deane J, Quigley EMM, Simrén M. An irritable bowel syndrome subtype defined by species-specific alterations in faecal microbiota. Gut. 2012;61(7):997–1006. doi:10.1136/gutjnl-2011-301501.
- Sundin J, Rangel I, Fuentes S, Heikamp‐de Jong I, Hultgren‐Hörnquist E, de Vos WM, Brummer RJ. Altered faecal and mucosal microbial composition in post‐infectious irritable bowel syndrome patients correlates with mucosal lymphocyte phenotypes and psychological distress. Aliment Pharmacol Ther. 2015;41(4):342–351. doi:10.1111/apt.13055.
- Zierer J, Jackson MA, Kastenmuller G, Mangino M, Long T, Telenti A, Mohney RP, Small KS, Bell JT, Steves CJ. et al. The fecal metabolome as a functional readout of the gut microbiome. Nat Genet. 2018;50(6):790. doi:10.1038/s41588-018-0135-7.
- Krumbeck JA, Walter J, Hutkins RW. Synbiotics for improved human health: recent developments, challenges, and opportunities. Annu Rev Food Sci Technol. 2018;9(1):451–479. doi:10.1146/annurev-food-030117-012757.
- Zaccaria E, Klaassen T, Alleleyn AME, Boekhorst J, Chervaux C, Smokvina T, Troost FJ, Kleerebezem M. L. rhamnosus CNCM I-3690 survival, adaptation, and small bowel microbiome impact in human. Gut Microbes. 2023;15(1):2244720. doi:10.1080/19490976.2023.2244720.
- Degen LP, Phillips SF. Variability of gastrointestinal transit in healthy women and men. Gut. 1996;39(2):299–305. doi:10.1136/gut.39.2.299.