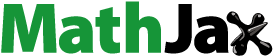
Abstract
Various meningococcal conjugate vaccines exist against serogroups A, C, W and Y. A new protein-based vaccine targeting serogroup B (MenB) is also now available. The potential of such vaccines to drive serogroup replacement is considered a possible public health concern when implementing nationwide routine immunization programmes. The aim of this work was to investigate if and how serogroup replacement may occur following widespread vaccination with a MenB vaccine that may protect against carriage. To that end, we built a dynamic transmission model with age and serogroup stratification, focusing on European settings where most invasive meningococcal disease (IMD) cases are caused by serogroups B and C. For illustration purposes, the model was employed in 2 such settings: UK (England and Wales) and Czech Republic. Preliminary model-based projections suggest that, under strong serogroup competition for colonization, vaccine-induced serogroup replacement may occur even with a relatively low vaccine efficacy against serogroup B carriage (e.g., 20%), with potential subsequent increase in serogroup C IMD. The magnitude and speed of the model-projected serogroup C IMD increase depend on the MenB vaccination strategy, vaccine efficacy against carriage and the extent of any potential cross-protection against other serogroups. These analyses are neither exhaustive nor definitive, and focused on simulating potential population-level trends in IMD post-vaccination, under certain assumptions. Due to present inherent limitations and uncertainties, this study has limited quantitative value and is best regarded as an explorative qualitative modeling approach, to complement and challenge the current status quo, and suggest areas where collecting additional data may be essential.
Abbreviations
CI | = | confidence intervals |
EU | = | European |
IMD | = | invasive meningococcal disease |
MenB | = | serogroup B meningococcal |
MenC | = | serogroup C meningococcal. |
Introduction
Invasive meningococcal disease (IMD) is a particularly serious but rare consequence of infection with Neisseria meningitidis. In contrast, asymptomatic nasopharyngeal carriage is common with a population prevalence of ~10% which varies with age, being highest in adolescents and lowest in young children. Most transmission of the meningococcus occurs silently through asymptomatic carriage, with carriage considered a pre-requisite for disease.Citation1 The epidemiology of IMD is extensively described and reviewed elsewhere, with serogroups B and C currently causing the majority of disease in Europe.Citation2 In contrast, the understanding of the dynamics and epidemiology of carriage remains incomplete. Studies investigating carriage prevalence by both serogroup and age are limited in number and are now becoming historical. Those available European (EU) carriage data indicate that serogroup B appears dominant among serogroupable carriage isolates.Citation3 Other important knowledge gaps of particular relevance include the contribution of similar commensal non-pathogenic species to the ecology of the nasopharynx (e.g., Neisseria lactamica) and the extent of any co-colonization by different meningococcal serogroups.
Monovalent and quadrivalent meningococcal conjugate vaccines targeting serogroup C and serogroups A, C, W and Y respectively have now been available for several years. An important component of their success is their additional ability to protect against carriage and reduce transmission, with significant herd protection induced especially when adolescents are targeted.Citation4 Routine schedules using these vaccines currently vary and may include infants, toddlers and/or adolescents.Citation5
The first meningococcal vaccine with the potential to provide broad coverage against serogroup B disease (4CMenB; Bexsero™) has recently been approved for use in Europe.Citation6 Developing a broadly protective serogroup B meningococcal (MenB) vaccine has been challenging because of the poor immunogenicity of the serogroup B capsule.Citation7 This has necessitated alternative approaches to those used for existing vaccines against serogroup A, C, W and Y disease, which use the organisms' polysaccharide capsules as the vaccine target. In contrast, 4CMenB is based on non-serogroup specific subcapsular proteins.Citation6,8
The ability of conjugate vaccines targeting bacterial meningitis to additionally protect against carriage has been an important component of their success.Citation9,10 At present it is not yet known if this could be replicated by immunization using 4CMenB. Preliminary data from a randomized controlled trialCitation11 in which United Kingdom university students received 2 doses of 4CMenB suggested that while the primary objectives (1 month post-vaccination) were not achieved, secondary analyses (from 3 months after dose 2) estimated a lower carriage of any N. meningitidis strain (18.2% [95% confidence intervals (CI), 3.4–30.8%] carriage reduction) and of capsular groups BCWY combined (26.6% [95% CI, 10.5–39.9%] carriage reduction), with 15.6% (95% CI, −11.0–35.9%) reduction for all sequence types of capsular B strains. However, only post-implementation surveys within large-scale vaccination programs can determine fully the population level impact of these vaccines.Citation11
Immunization with 4CMenB is intended to stimulate the production of bactericidal antibodies that recognize certain antigens and are expected to be protective against IMD. As these antigens are variably expressed by different strains, meningococci that express them at sufficient levels are susceptible to killing by vaccine-elicited antibodies.Citation12 A survey of approximately 1,000 different invasive meningococcal group B isolates collected during 2007–2008 in 5 EU countries showed that, overall, 78% of the strains were potentially susceptible to vaccine induced antibodies.Citation6,13 The efficacy of 4CMenB has not yet been evaluated through clinical trials, but inferred based on correlates of protection by demonstrating the induction of serum bactericidal antibody responses to each of the vaccine antigens.Citation6,12 One month following a 3-dose primary schedule in infants and a 2-dose schedule in older children and adolescents, respectively, protective serum bactericidal activity titers were achieved in most vaccinated individuals.Citation14-16
Regarding the potential strain coverage of the 4CMenB vaccine in non-B meningococci, a recent study that estimated the effect of 4CMenB on non-B meningococci by applying positive bactericidal thresholds established for MenBCitation17 found that overall predicted strain coverage of 4CMenB among non-B meningococcal isolates was 68% (95% CI, 44–79%). Capsular group-specific strain coverage was 80% (95% CI, 58–88%) for group C, 83% (95% CI, 44–94%) for group W-135, and 22% (95% CI, 12–38%) for group Y.Citation17 Serum bactericidal activity using human complement data for non-B strains will be needed to confirm whether the positive bactericidal thresholds derived for MenB strains will also apply to non-B strains.
These preliminary studies offer some indication that this vaccine may protect not only against disease caused by a wide range of serogroup B strains, but also potentially additionally provide both some protection against carriageCitation7 and cross protection against other capsular serogroups.Citation17 However, evidence is currently limited and these aspects remain to be further demonstrated. Therefore at present important assumptions need to be made regarding potential performance characteristics for 4CMenB in terms of these aspects. These can only be guided to some limited extent by those existing data available and may ultimately only become fully apparent following routine introduction of the vaccine and assessment of appropriate observational data.
An important public health concern of such vaccines with an ability to protect against asymptomatic carriage is their potential to drive a serogroup replacement event where, following their widespread introduction, other serogroups subsequently fill the ecological niche vacated by vaccine targeted serogroups being carried. This may potentially lead to some increase in disease caused by non-vaccine serogroups. For example, it is well documented that use of pneumococcal conjugate vaccines has resulted in a decrease in carriage and disease of vaccine serotypes and an increase in colonization and disease caused by non-vaccine serotypes.Citation18,19 To date there is no evidence that selective vaccination against certain meningococcal serogroups has led to a clinically significant increase in disease caused by non-vaccine serogroups. However, observation has mainly been limited to vaccination strategies involving monovalent serogroup C conjugate vaccine, corresponding to instances where carriage prevalence and subsequent potential for serogroup replacement were low.
Given the evidence that serogroup B meningococci may often be carried at a higher prevalence than other serogroups, there could in theory be significant potential to open a larger ecological niche and cause a serogroup replacement event if protein-based MenB vaccines are widely adopted and are able to impact carriage, subsequently driving up cases of IMD due to other serogroups. Here, we build a dynamic transmission model focusing on European settings (Western/Central EU countries) where most disease is caused by serogroups B and C, aiming to investigate and test hypotheses exploring if and how serogroup replacement may potentially occur following widespread vaccination with a MenB vaccine that may potentially offer some protection against carriage. To date, the dynamic transmission models developed by Trotter and colleaguesCitation20 have proved influential and often been used to guide vaccine policy makers. More recent model developments by the same group have focused on the cost-effectiveness of a serogroup B vaccine.Citation21 However, thus far none of the existing modeling frameworks has considered potential for serogroup replacement post-vaccination. Here we attempt to go beyond the existing models and allow potential for serogroup replacement to be considered. Of note, such approaches have been already employed for other pathogens like Streptococcus pneumoniae to evaluate vaccine-induced serotype replacement.Citation22,23 However, this currently necessitates use of a number of important assumptions relating to various levels of uncertainty pertaining to the epidemiology of meningococcal carriage and disease and the specific performance characteristics of the meningococcal vaccine under consideration. Key knowledge gaps remain with only limited supporting data. These aspects are described in detail in the Materials and Methods section at the end of this article, including relevant supporting evidence used to inform those assumptions used.
Results
Vaccination scenarios
Our primary objectives in this work were to assess the potential for epidemiological changes ("What-If" case scenarios) and to project potential subsequent trends in IMD at population-level in the EU setting following the introduction of MenB vaccination, with focus on potential serogroup replacement. The UK (England and Wales) and Czech Republic were selected for illustrative purposes, considered to reflect 2 different EU scenarios for comparative simulations here. This was based on the availability of age- and serogroup-stratified IMD data and a number of underlying differences: larger population (UK) vs. smaller population (Czech Republic), higher IMD incidence (UK) vs. lower IMD incidence (Czech Republic), and a different history of serogroup C meningococcal (MenC) vaccination (not included in the routine Czech childhood immunization schedule but well established in the UK).
The present modeling framework is sufficiently versatile to enable consideration of various vaccination strategies and scenarios. The following specific vaccination scenarios were considered of interest for the present simulations and are summarized in :
Table 1. 4CMenB vaccination scenarios
Routine infant/toddler vaccination, with primary series completed by 6 months of age and potential routine booster at the age of 1 y (scenarios 1–4);
Routine adolescent vaccination at the age of 17 y old, for instance prior to/upon admission to college (scenarios 5–7);
A one-time catch-up campaign in everybody aged 1–17 y old at the start of the routine MenB vaccination (scenarios 3 and 4).
Actual data, assumptions and parameters employed for the present simulations are described in a dedicated subsection (Data and parameters used for simulations) in the Methods section. All the model-based projections are shown here over time, for a period of 100 y post-vaccination, for a more complete illustration; however, in many of these simulations the change in the dynamics introduced by the serogroup-interplay post-vaccination is strong enough so that reaching an actual steady-state post-vaccination may take considerably longer beyond a 100-year time-span. For all practical purposes, however, given all the epidemiological changes that can naturally occur over longer time spans, model-projections over the shorter term (e.g., first 20 y post-vaccination) are likely more informative.
Model outcomes in the UK setting (England and Wales)
Baseline model
Starting in late 1999, following a significant increase in the number of cases of IMD caused by serogroup C, the UK introduced MenC vaccination into the routine immunization schedule. This led to significant changes in the epidemiology of IMD over the course of the next 10 y.Citation24
For completeness, here we calibrate the risk of IMD given carriage for 2 separate instances: the first, which we refer to as the "High-incidence IMD scenario," is based on IMD data from 1998–1999 in England and Wales, prior to introduction of MenC vaccination; the second, which we refer to as the "Low-incidence IMD scenario," is then based on the average IMD data from 2005–2009 in England and Wales, corresponding to a situation where the IMD caused by serogroups C and Others would be very low compared to the IMD caused by serogroup B.
Our model is able to capture the age- and serogroup-specific carriage prevalence, as well as corresponding IMD at baseline in each of the 2 scenarios described above, as illustrated in (carriage and IMD), respectively, which constitutes a key pre-requisite to ensure a reasonable model foundation for further simulating potential impact of vaccination.
Figure 1. (A) Illustration of best model fit against the synthesized carriage prevalence data at baseline, age-and serogroup-stratified, for the UK setting. (B) Illustration of best model fits against the IMD data for the UK setting (England and Wales) at baseline: annual number of cases, age-and serogroup-stratified. Left panel, "High-incidence IMD scenario," based on IMD data in 1998/1999; right panel, "Low-incidence IMD scenario" based on averaged IMD data from 2005–2009. Data-points corresponding to the following age groups (in years):<1, 1–4, 5–9, 10–14, 15–19, 20–24 and ≥25 .
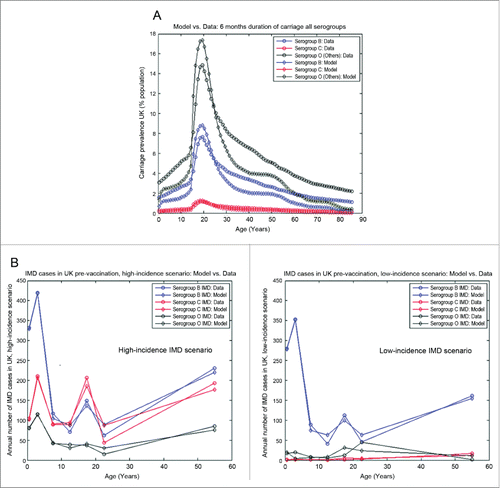
It is of note that the best-fit for the carriage component () here yielded very high corresponding reductions in the serogroup-specific risks for co-colonization: 97.6% for serogroup B, 97.8% for serogroup C, 97.5% for Others, which practically means very small niches for dual colonization and very strong competition for colonization.
For IMD (), it is apparent that in the "High-incidence IMD scenario," the risk of disease given carriage for serogroups B and C is considerably higher than for all Others, which dominate the carriage landscape but cause the fewest number of IMD cases. This is consistent with the Others category pooling together the less pathogenic strains. In the "Low-incidence IMD scenario," on the other hand, both serogroups C and Others would have a very low risk of disease given carriage, and serogroup B IMD would fully dominate.
Model-based outcomes for routine infant vaccination
The model-projected potential trends in the annual number of IMD cases over time in the UK setting (England and Wales) following scenario 1 (routine MenB infant vaccination with routine booster at 1 y of age starting from an unaltered high-incidence IMD scenario at baseline) with no cross-protection effects of the MenB vaccine are shown in , corresponding to an assumed (low) baseline of 20% vaccine efficacy against serogroup B carriage () vs. an assumed (high) value of 60% vaccine efficacy against serogroup B carriage (). In the first case, the decline in serogroup B IMD over time post-vaccination is limited (); no significant herd protection can be achieved here. In addition, the duration of protection is assumed relatively short in this case, with vaccinated individuals protected up to 5 y of age following booster. In the second case, a higher vaccine efficacy against carriage can lead to more serogroup replacement post-vaccination, due to a steeper decline in serogroup B carriage followed by an increase in serogroup C and Others carriage. This reflects subsequently in a more marked increase in serogroup C IMD in particular, as the risk of disease given carriage is considerably higher for serogroup C compared to Others. Overall in this scenario, the more pronounced decline in serogroup B IMD may be offset by the subsequent increase in serogroup C IMD primarily, as shown in , potentially diluting the net impact on the total IMD reduction.
Figure 2. Model-projected potential trends in the annual number of IMD cases over time in the UK setting (England and Wales) following routine MenB infant vaccination with routine booster at 1 y of age starting from an unaltered high-incidence IMD scenario at baseline (scenario 1). Serogroup-specific IMD (pooled across all age groups) and total IMD across all serogroups. (A) 20% assumed vaccine efficacy against serogroup B carriage. (B) 60% assumed vaccine efficacy against serogroup B carriage. Vaccine coverage: 85%. Vaccine efficacy against serogroup B IMD: 78%. No cross-protection effects.

When starting from a low-incidence IMD scenario at baseline (scenario 2), the risk of IMD given carriage is very low for both serogroup C and Others. As a consequence, replacement in carriage for serogroup C and Others is significantly offset in the corresponding IMD. The epidemiological landscape in such a case would be practically dominated by serogroup B IMD, with corresponding net reductions in total IMD illustrated in .
Figure 3. Model-projected potential trends in the annual number of IMD cases over time in the UK setting (England and Wales) following routine MenB infant vaccination with routine booster at 1 y of age, starting from a low-incidence IMD scenario at baseline (scenario 2). Serogroup-specific IMD (pooled across all age groups) and total IMD across all serogroups. (A) 20% assumed vaccine efficacy against serogroup B carriage. (B) 60% assumed vaccine efficacy against serogroup B carriage. Vaccine coverage: 85%. Vaccine efficacy against serogroup B IMD: 78%. No cross-protection effects.
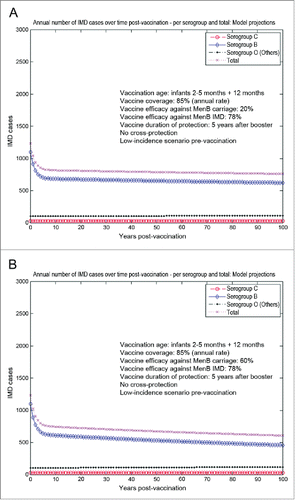
The model-based projections for infant vaccination under scenario 3 (no booster at 12 months of age plus a one-time MenB catch-up campaign in 1–17 year-olds, mimicking the original MenC vaccination campaign in the UK), starting from an unaltered high-incidence IMD scenario at baseline, are shown in . Here we assumed the low baseline value of 20% vaccine efficacy against serogroup B carriage, and performed a head-to-head comparison of corresponding IMD outcomes for a case with no cross-protection () vs. a case with assumed MenB vaccine cross-protection as described above (). The importance of a catch-up campaign toward faster and more substantial reduction in serogroup B IMD, particularly in the first 10–20 y post-vaccination, is apparent. Vaccinating individuals at older ages (particularly adolescents, which are the main carriage reservoir) introduces more potential for herd protection effects, in effect even at a 20% assumed vaccine efficacy against serogroup B carriage, and duration of vaccine protection is also assumed considerably longer (10 years). However, in the absence of any vaccine cross-protective effects against other serogroups, the accelerated reduction in serogroup B IMD is subsequently followed by a corresponding marked increase in serogroup C IMD in particular, which would eventually cause a significant offset in total IMD net reduction over time (). There is a great potential for vaccine cross-protective effects, which could have a big subsequent impact on overall IMD across all serogroups ().
Figure 4. Model-projected trends in the annual number of IMD cases over time in the UK setting (England and Wales) following routine MenB infant only (no booster) vaccination plus a one-time MenB catch-up campaign in 1–17 y old, starting from an unaltered high-incidence IMD scenario at baseline (scenario 3). Serogroup-specific IMD (pooled across all age groups) and total IMD across all serogroups. (A) 20% vaccine efficacy serogroup B carriage, 78% vaccine efficacy serogroup B IMD, no cross-protection. (B) 20% and 78% vaccine efficacy serogroup B carriage and IMD, respectively, 10% and 80% cross-protective efficacy serogroup C carriage and IMD, respectively, and 5% and 50% cross-protective efficacy all Others carriage and IMD, respectively. Vaccine coverage: age-dependent.
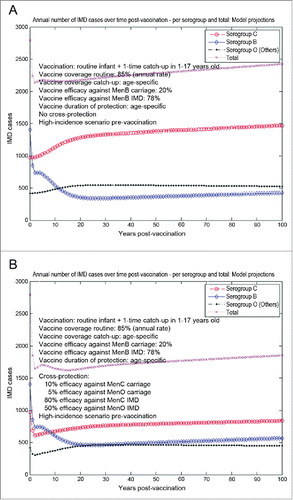
A note-worthy aspect in all these simulations is that decrease in MenB IMD post-vaccination may be further enhanced by serogroup replacement in a double feedback effect: vaccine-induced reduction in serogroup B leads to a subsequent increase in serogroup C and Others which can further enhance reduction in serogroup B via serogroup competition.
Compared to the previous scenario, in scenario 4 the MenB vaccination campaign would only start after 15 y (e.g., in 2015) of similar vaccination strategy with a MenC vaccine with assumed 70% efficacy against serogroup C carriage and 80% efficacy against serogroup C IMD. For all practical purposes, this case comes closest to simulating a more realistic picture for the UK, where the N. meningitidis epidemiological landscape was altered by the MenC vaccination programs that have been in place for over a decade now. With MenC vaccination in-place for 15 y prior to introduction of MenB, the serogroup C carriage and IMD are driven very low, while there is a mild increase in IMD caused by serogroup B and Others, more pronounced for serogroup B which has a higher risk of disease given carriage than the Others (). In these simulations, this is a direct consequence of a mild serogroup replacement caused here by the MenC vaccination during the initial 15 years; the replacement is only mild, as the original serogroup C carriage prevalence at baseline was already quite low, as illustrated in , hence presenting limited potential to create a significant ecological niche post-vaccination. Thus, at the start of the MenB vaccination campaign in this case, the serogroup C no longer plays an important role, and the focus shifts to the interaction between serogroup B and Others. As the risk of disease given carriage is the lowest in Others, there is practically less opportunity for cross-protection effects to make a significant impact, and total IMD net reductions without () or with MenB vaccine cross-protection () are comparable.
Figure 5. Model-projected trends in the annual number of IMD cases over time in the UK setting (England and Wales) following routine MenB infant only (no booster) vaccination plus a one-time MenB catch-up campaign in 1–17 y old, starting after 15 y of similar vaccination strategy with a MenC vaccine (scenario 4). Serogroup-specific IMD (pooled across all age groups) and total IMD across all serogroups. (A) 20% vaccine efficacy serogroup B carriage, 78% vaccine efficacy serogroup B IMD, no cross-protection. (B) 20% and 78% vaccine efficacy serogroup B carriage and IMD, respectively, with 10% and 80% cross-protective efficacy serogroup C carriage and IMD, respectively, and 5% and 50% cross-protective efficacy all Others carriage and IMD, respectively.
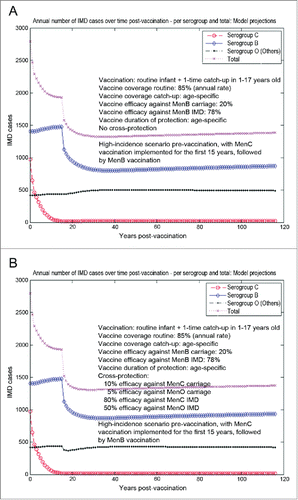
Model-based outcomes for routine adolescent vaccination
The model-projected potential trends in the annual number of IMD cases over time in the UK setting (England and Wales) under scenario 5 (routine MenB adolescent vaccination at the age of 17 y old, starting from an unaltered high-incidence IMD scenario at baseline) are shown in . Routine adolescent vaccination can enhance herd protection, even at relatively low (20%) vaccine efficacy against carriage. In the absence of any MenB vaccine cross-protective effects (a), while significant reduction in serogroup B IMD may be achieved relatively fast, there is potential for marked serogroup replacement over time, subsequently leading to a significant increase in serogroup C disease in particular. In such a case-scenario, great potential may exist for vaccine cross-protective effects (), with significant subsequent impact on overall IMD across all serogroups. However, if the MenB routine adolescent vaccination at the age of 17 y old started after 15 y (e.g., in 2015) of similar vaccination strategy with a MenC vaccine with 70% efficacy against serogroup C carriage and 80% efficacy against serogroup C IMD (scenario 6, ), the model suggests there is reduced opportunity for cross-protection effects, and total IMD net reductions without () or with MenB vaccine cross-protection () are comparable.
Figure 6. Model-projected trends in the annual number of IMD cases over time in the UK setting (England and Wales) following routine MenB adolescent vaccination at the age of 17 y old, starting from an unaltered high-incidence IMD scenario at baseline (scenario 5). Serogroup-specific IMD (pooled across all age groups) and total IMD across all serogroups. (A) 20% assumed vaccine efficacy against serogroup B carriage, 78% vaccine efficacy against serogroup B IMD, no cross-protection. (B) 20% and 78% assumed vaccine efficacy against serogroup B carriage and IMD, respectively, 10% and 80% assumed cross-protective efficacy against serogroup C carriage and IMD, respectively, and 5% and 50% assumed cross-protective efficacy against all Others carriage and IMD, respectively. Vaccine coverage: 60%.
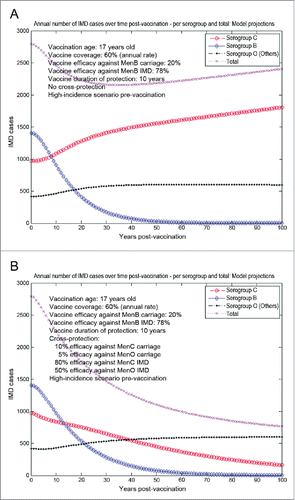
Figure 7. Model-projected trends in the annual number of IMD cases over time in the UK setting (England and Wales) following routine MenB adolescent vaccination at the age of 17 y old, starting after 15 y of similar vaccination strategy with a MenC vaccine (scenario 6). Serogroup-specific IMD (pooled across all age groups) and total IMD across all serogroups. (A) 20% vaccine efficacy against serogroup B carriage, 78% vaccine efficacy against serogroup B IMD, no cross-protection. (B) 20% and 78% vaccine efficacy against serogroup B carriage and IMD, respectively, 10% and 80% cross-protective efficacy against serogroup C carriage and IMD, respectively, and 5% and 50% cross-protective efficacy against all Others carriage and IMD, respectively. Vaccine coverage: 60%.

Finally, when starting from a low-incidence IMD scenario at baseline (scenario 7), the risk of IMD given carriage is very low for both serogroup C and Others and replacement in carriage for serogroup C and Others is consequently offset in the corresponding IMD (). The epidemiological landscape for this scenario would be practically dominated by serogroup B IMD, and significant net reductions in total IMD can be achieved.
Figure 8. Model-projected potential trends in the annual number of IMD cases over time in the UK setting (England and Wales) following routine MenB adolescent vaccination at the age of 17 y old, starting from a low-incidence IMD scenario at baseline (scenario 7). Serogroup-specific IMD (pooled across all age groups) and total IMD across all serogroups. (A) 20% vaccine efficacy against serogroup B carriage, 78% vaccine efficacy against serogroup B IMD, no cross-protection. (B) 20% and 78% vaccine efficacy against serogroup B carriage and IMD, respectively, 10% and 80% cross-protective efficacy against serogroup C carriage and IMD, respectively, and 5% and 50% cross-protective efficacy against all Others carriage and IMD, respectively. Vaccine coverage: 60%.
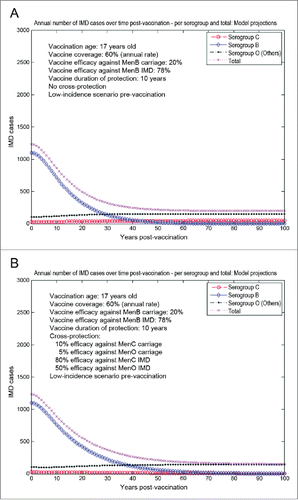
Model outcomes in the Czech setting
Baseline model
Unlike the UK, the Czech Republic has not yet to date introduced routine vaccination against serogroup C. The overall burden of disease at the population level is relatively low, with serogroup distribution comparable to the UK setting in the "High-incidence IMD scenario." Like in the UK setting above, our model is able to capture the age- and serogroup-specific carriage prevalence, as well as corresponding IMD in the Czech Republic, as illustrated in , with carriage shown in the left panel and IMD in the right panel, respectively. We assumed similar values of the serogroup-specific reduction factors for co-colonization in both the UK and Czech settings, as a pathogen-specific characteristic rather than setting-specific. Such assumptions can however be further relaxed if new data/evidence become available indicating otherwise.
Figure 9. Illustration of best fit model for the Czech setting at baseline, with age and serogroup stratification. Left panel: model fit against the synthesized carriage prevalence data. Right panel: model fit against the IMD data (annual number of cases, with data-points corresponding to the following age groups (in years):<1, 1–4, 5–9, 10–14, 15–19, 20–24, 25–34, 35–44, 45–54, 55–64 and ≥65 ). NRL, National Reference Laboratory for Meningococcal Infections, National Institute of Public Health, Prague, Czech Republic.
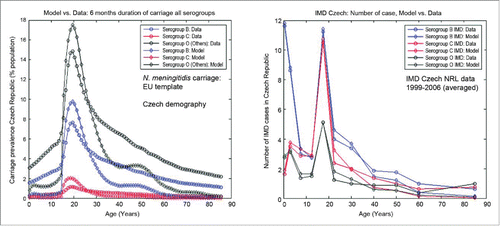
Model-based outcomes for routine vaccination
For the Czech setting, we show here results under scenario 1 (routine MenB infant vaccination with booster) in , and under scenarios 5 and 6 (routine MenB adolescent vaccination) in , respectively. The trends over time post-vaccination are consistent with their counterparts from the UK setting shown in for the routine infant strategy and for the routine adolescent strategy, which were described in more detail above.
Figure 10. Model-projected potential trends in the annual number of IMD cases over time in the Czech Republic following routine MenB infant vaccination with routine booster at 1 y of age (scenario 1). Serogroup-specific IMD (pooled across all age groups) and total IMD across all serogroups shown in the top panels. (A) 20% assumed vaccine efficacy against serogroup B carriage. (B) 60% assumed vaccine efficacy against serogroup B carriage. Vaccine coverage: 85%. Vaccine efficacy against serogroup B IMD: 78%. No cross-protection effects.
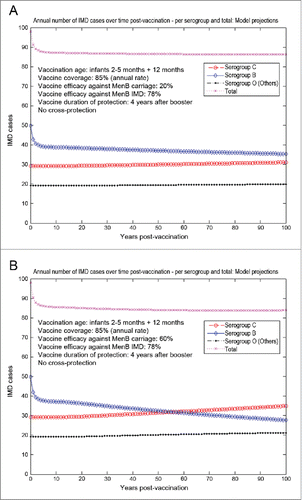
Overall, the model-projected trends in IMD post-vaccination appear consistent across the UK and Czech settings.
Discussion
We have built an age-structured dynamic transmission model for N. meningitidis focusing on EU settings where most invasive disease is caused by serogroups B and C, as a proof-of-concept aiming to investigate if and how serogroup replacement may potentially occur following widespread vaccination with a MenB vaccine impacting carriage.
The key qualitative insights based on the current simulations are:
the interplay between serogroups B and C may be key in EU settings;
under strong serogroup competition for colonization, vaccine-induced serogroup replacement may be possible even at relatively low (e.g., 20%) levels of MenB vaccine efficacy against carriage;
notable increase in serogroup C IMD may occur as a consequence of potential serogroup replacement post-vaccination with a MenB vaccine; the magnitude (how much) and speed (how fast) of this increase would depend on the MenB vaccination strategy, as well as the MenB vaccine efficacy against carriage and potential cross-protection;
decrease in serogroup B IMD post-vaccination may be further enhanced by serogroup replacement, in a double feedback effect: vaccine-induced reduction in serogroup B may lead to a subsequent increase in serogroup C and Others which may further enhance reduction in serogroup B via serogroup competition;
under certain circumstances, potential cross-protection effects of a MenB vaccine may play an important role towards alleviating impact of potential serogroup replacement on overall IMD; however, if serogroup C IMD is sufficiently low before MenB vaccination, the net additional impact of serogroup replacement on overall IMD may not be as important, and MenB vaccine cross-protection effects may be of less consequence.
The current model version is evaluating the potential for serogroup replacement following MenB vaccination at population-level under the assumption that different serogroups interact/compete for colonization. The evidence of capacity for sustained co-colonization with different strains for longer periods of time is quite limited (here we only allowed up to 10% dual colonization). This is supported in part by those little data that are currently available,Citation23,25,26 and led to a potential configuration where the different serogroups explicitly represented in the model (B, C, and Others) may exhibit strong competition for colonization while still co-existing at the population level (heterogeneity introduced by age structure in the model can enable such configurations).Citation27 This produces a delicate balance, which can be very sensitive to even small perturbations introduced by vaccination. When considering potential serogroup-replacement, this is likely a worst case scenario. While changes in epidemiology are possible,Citation28,29 the model outcomes shown here might well potentially over-estimate the magnitude of serogroup replacement at a population level, possibly driven down in practice by secular trends and/or evolving carriage dynamics and/or interaction with other commensals (e.g., N. lactamica)/environmental factors (presently not included in the model). At a minimum, as a starting point, more studies investigating the actual likelihood and extent of sustained serogroup co-colonization and documenting prevalence of multiple carriage of N. meningitidis in different settings would be needed, given the key role such data may have on potential for serogroup replacement.
We stress on the fact that the analyses presented here were neither intended as exhaustive nor definitive, and were focused primarily on potential qualitative trends post-vaccination with a MenB vaccine under different scenarios. This study was a proof of concept to evaluate if and how (under what conditions) potential serogroup replacement post-MenB vaccination may occur. Due to inherent limitations and uncertainties at present, this piece of work is not intended for decision making or related economic analyses and has to be regarded solely as a "What-If" type of modeling study, testing and generating hypotheses to complement and challenge existing knowledge, and eventually point to areas where collecting additional data may be of essence. Some readily apparent key limitations are discussed below.
Carriage dynamics over time might play an important role; however, very limited appropriate carriage data investigating this aspect are currently available, with little knowledge of how it may actually vary over time. In the absence of such longitudinal carriage data, current model version assumes endemic equilibrium pre-vaccination. Also, in the absence of robust contemporary country-specific age-stratified carriage prevalence data, in the present analyses we opted for employing a common well-documented EU carriage template with age stratification,Citation1 on which we applied a serogroup distribution uniformly based on very limited available literature,Citation30 resulting in a synthesized carriage profile with age and serogroup stratification. This was further used for model calibration in conjunction with country-specific demography. In reality, the actual serogroup distribution may be different across different age groups, and actual age- and serogroup-specific carriage prevalence may well be country-specific, with subsequent impact on setting-specific model calibration and outcomes. Collection of longitudinal country-specific carriage data with age and serogroup stratification is therefore crucial toward more realistic modeling studies.
The potential interplay, interaction and competition between both different serogroups and with other commensals is currently also not well studied, introducing considerable uncertainty when trying to account for this aspect. Corresponding assumptions (e.g., strength of competition for colonization of susceptible hosts) can have a significant impact on subsequent model-based projections for vaccination outcomes, in particular regarding potential for serogroup replacement post-vaccination.
The relationship between carriage and invasive disease is not well understood at present, so it is currently modeled in a top-level way, with no specific mechanisms considered. Also, in the current model version, risk of disease given carriage at the population level is assumed constant over time. In theory, such an assumption could be adjusted so that the risk of disease is allowed to vary over time for instance, but this should be undertaken with caution given the absence of appropriate evidence, in particular that providing a better understanding of carriage dynamics over time, and could further lead to artifacts in the model-based projections (e.g., if the risk of disease may decline naturally, then it becomes more difficult to distinguish the true impact of vaccination).
The model-projected impact of MenB vaccination, and related level of potential serogroup replacement post-vaccination, could well be different from setting to setting depending on the specific local epidemiological landscape (carriage/IMD distribution). Preliminary model-based projections highlight the potential importance of considering MenC vaccination in conjunction with MenB vaccination in EU settings where IMD caused by serogroups B and C is predominant. Also, the importance of MenB vaccines with appropriate levels of cross-protection is apparent. We stress the fact that the values used in these analyses for different types of vaccine efficacy are hypothetical at present, particularly in terms of impact against carriage and cross-protection effects. The present modeling study and related outcomes are intended solely for illustrative purposes in the context. They primarily serve to challenge the current status quo and stimulate further thinking about the importance of collecting data to elucidate aspects that may play an important role toward potential epidemiological changes, particularly following introduction of mass vaccination.
Materials and Methods
Overview of the mathematical model
The aim here was to develop an age-structured dynamic transmission model for N. meningitidis, allowing potential interaction between different serogroups, for primary use in EU settings where serogroups B and C cause most of the invasive meningococcal disease.
Dynamic transmission models are valuable mathematical tools to help simulate the transmission of infectious diseases in a population and to assess the subsequent potential impact of preventative measures. A dynamic transmission modeling framework enables more complex assessments of population-level impact of vaccination, while accounting for different complexities of transmission dynamics, including potential herd protection effects and serogroup replacement. Such models are typically employed to project infection reduction at population level following various vaccination/control scenarios under different working assumptions.
The approach is mechanistic and deterministic. The baseline model structure, with underlying states and corresponding flows, is illustrated in .
Figure 11. Model-projected potential trends in the annual number of IMD cases over time in the Czech Republic following routine MenB adolescent vaccination at the age of 17 y old (scenario 5). Serogroup-specific IMD (pooled across all age groups) and total IMD across all serogroups shown. (A) 20% assumed vaccine efficacy against serogroup B carriage, 78% vaccine efficacy against serogroup B IMD, no cross-protection. (B) 20% and 78% assumed vaccine efficacy against serogroup B carriage and IMD, respectively, 10% and 80% assumed cross-protective efficacy against serogroup C carriage and IMD, respectively, and 5% and 50% assumed cross-protective efficacy against all Others carriage and IMD, respectively. Vaccine coverage: 60%.

Figure 12. Model-projected trends in the annual number of IMD cases over time in the Czech Republic following routine MenB adolescent vaccination at the age of 17 y old, starting after 15 y of similar vaccination strategy with a MenC vaccine (scenario 6). Serogroup-specific IMD (pooled across all age groups) and total IMD across all serogroups in the top panels. (A) 20% vaccine efficacy against serogroup B carriage, 78% vaccine efficacy against serogroup B IMD, no cross-protection. (B) 20% and 78% vaccine efficacy against serogroup B carriage and IMD, respectively, 10% and 80% cross-protective efficacy against serogroup C carriage and IMD, respectively, and 5% and 50% cross-protective efficacy against all Others carriage and IMD, respectively. Vaccine coverage: 60%.
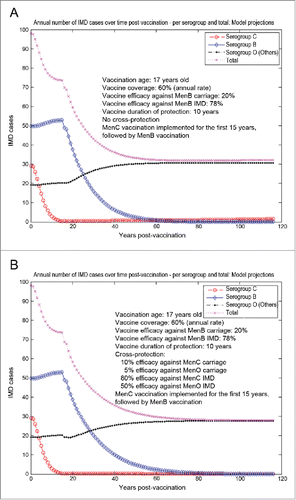
Model structure and assumptions
Baseline model
– | Serogroups B and C are explicitly and separately accounted for, while all the other serogroups (including the nongroupable) are pooled together under the Others category; this is a simplification appropriate for EU countries (where serogroups B and C cause most of the IMD) to reduce model complexity, particularly in the absence of more detailed serogroup-specific data. | ||||
– | There is implicit interaction between different serogroups, which are allowed to compete for the Susceptible pool. We also allow for dual co-colonization (simultaneous carriage of serogroups B and C, B and Others, and C and Others, respectively, without accounting for the actual order in which this may occur), with potential reduced risk of colonization by a different class if already carrying one. A key aspect that will influence potential for serogroup replacement is the strength of competition and subsequent possibility of sustained co-colonization.Citation23 The stronger the competition, the less co-colonization and more potential for serogroup replacement. Very limited evidence exists for N. meningitidis, which seems to indicate that simultaneous carriage of different serotypes of N. meningitidis is rare.Citation25,26 In a multiple-country questionnaire-based survey discussed at the bi-annual meeting of the European Monitoring Group on Meningococci (EMGM) 2009, no significant carriage of multiple strains was reported (0.9% in the Czech Republic, very low in the UK, none in Norway, Greece and New Zealand). In a carriage study in healthy Dutch children,Citation31 while overall low carriage prevalence was reported (consistent with that particular age segment), out of 8 and 9 children colonized with serogroups B and C, respectively, only 1 was a carrier of 2 different strains (B+C). Based on this limited evidence, in the current model we allow up to 10% dual colonization, hereby imposed as a constraint at the time of model calibration. | ||||
– | For simplicity, the average duration of carriage here is assumed the same across all serogroups (B, C, and Others, respectively), and it is fixed at 6 months.Citation32 The clearance rate is defined as 1/(average duration of carriage). | ||||
– | Acquisition of carriage is serogroup-specific (B, C, and Others, respectively), governed by a serogroup-specific time-varying force of infection. | ||||
– | Individuals with single colonization of a given serogroup (B, C, or Others) are equally infectious with dual-colonized individuals carrying the same serogroup (they contribute equally to the serogroup-specific force of infection). In the absence of data documenting potential differences between carriers of a single serogroup vs. carriers of multiple serogroups (e.g., bacterial density, thresholds for infectiousness, etc.), this is the simplest assumption that also prevents the need to introduce additional unknown parameters into the model. Similar approaches have been employed in related contexts for other pathogens.Citation22 The modeling framework can allow for potential different levels of infectiousness, but data would be needed to support such further considerations. | ||||
– | N. meningitidis is transmitted from person-to-person through droplets of respiratory or throat secretions from carriers. IMD is not an essential component for the transmission cycle.Citation20,33 Close and prolonged contacts or living in close quarters with an infected person (a carrier) facilitate transmission. In order to capture adequate mixing between people of different ages here, we employed an empirical social contact matrix (based on POLYMOD,Citation34 courtesy of Dr. Niel Hens, Hasselt University) for close contacts longer than 15 minutes. Such empirical matrices are employed here only as a mean to render contact patterns, assuming similar social contact patterns in Western EU countries.Citation34 The rate of social contacts between individuals in different age groups can be further multiplied by various proportionality factorsCitation35,36 to render an actual infection-specific transmission matrix. In the present work, in order to account for inherent differences between different serogroups transmission, we allow for serogroup-specific proportionality factors: one for serogroup B, one for serogroup C, and one for all Others. | ||||
– | Regarding the possibility of dual colonization, we allow for a reduced risk of colonization by a different serogroup if already carrying one. This can be modeled through corresponding natural reduction factors in the corresponding serogroup-specific force of infection.Citation37 Here we consider serogroup-specific reduction factors, one for each serogroup represented in the model (B, C, and Others, respectively). These are characterized by 3 model parameters a priori unknown, constrained between values of 0 and 1, estimated as an integral part of the overall calibration process by fitting the model-based age- and serogroup-stratified carriage prevalence against corresponding carriage data under the current model assumptions and related constraints. Values equal to 1 here would characterize 100% reduction in the corresponding risk of dual colonization and thus complete exclusion of dual colonization (maximal serogroup competition for colonization), while conversely values equal to 0 would represent 0% reduction in the corresponding risk of dual colonization and complete serogroup independence (no serogroup competition for colonization). With this interpretation, values close to 1 indicate strong competition for colonization, while conversely values close to 0 would indicate weak competition. | ||||
– | As in the original models by Trotter et al,Citation20 IMD is considered separately outside the transmission cycle, linked to carriage by a serogroup - and age-dependent risk function, defined as the ratio between the incidence of IMD and the incidence of carriage. This function is modeled as a parametric function of age for each serogroup represented in the model (B, C, and Others, respectively), with the underlying set of parameters estimated by fitting against serogroup – and age-stratified IMD data. The approach is described in detail in Trotter et al.Citation38 |
Vaccination model
We include 3 separate compartments for vaccination, to represent routinely vaccinated and protected, vaccinated and waned, and catch-up vaccinated and protected, respectively. All the 'Vaccinated' compartments in our model replicate the structure of the baseline model illustrated in , with corresponding states and flows. While the resulting modeling framework is sufficiently versatile to enable consideration of various potential vaccine modes-of-action, like faster clearance rates or reduced infectiousness in vaccinated and protected compared to unvaccinated individuals, such potential additional effects were not considered in the present analyses. Besides the impact of a vaccine on IMD, we only consider the potential of a vaccine to have an effect on carriage, translated into a reduction in the risk of carriage acquisition in vaccinated and protected individuals compared to unvaccinated individuals. Throughout this paper, we refer to this as vaccine efficacy against carriage. Further, we also consider a reduction in the risk of IMD in vaccinated and protected carriers, for simplicity referred to systematically as vaccine efficacy against IMD throughout the paper. Both types of efficacy, against carriage and IMD, respectively, can be serogroup-specific, allowing for potential vaccine cross-protection effects.
We assume that the duration of vaccine protection against carriage and IMD is the same.
Mathematical framework
The original mathematical model formulation with continuous age structure is a classic one, based on a set of nonlinear partial differential equations with respect to time and ageCitation39,40 , illustrated below for the baseline model.
Following the schematic in , let α = age (with A=the maximum age/life expectancy) , t=time , : number of susceptible individuals,
: number of individuals with single carriage of serogroup B,
: number of individuals with single carriage of serogroup C,
: number of individuals with single carriage of serogroup Other,
: number of individuals with double carriage of serogroups B and C,
: number of individuals with double carriage of serogroups B and Other, and
: number of individuals with double carriage of serogroups C and Other.
Then the baseline transmission model can be formally written as follows:
where represent serogroup-specific carriage clearance rates (inversely proportional with the corresponding carriage duration; here we assumed for simplicity
);
represent serogroup-specific reduction factors in the corresponding force of infection for co-colonization, and
represent the serogroup-specific transmission rates between people of age a and
.
is the age-specific natural death rate.
For the actual numerical solutions here, we used an age discretization with 1-year age groups for all ages >1 y old and <86 y old (which is the assumed life expectancy in this case), and two 6-month age groups for the 0–1 y old age segment.
Model calibration and validation
Model calibration is done here as follows:
– | The baseline dynamic transmission model with age structure is calibrated by fitting the model-based age- and serogroup-stratified carriage prevalence against corresponding carriage data. | ||||
– | The age-dependent serogroup-specific risk of IMD given age- and serogroup-stratified carriage is further calibrated by also fitting the model-projected age- and serogroup-stratified IMD against corresponding IMD data. |
Appropriate combined carriage/IMD data is essential for full calibration/validation of such modeling frameworks. Here, for the baseline model calibration, we work under the assumption of endemic equilibrium, which is an accepted practice for N. meningitidis in developed countries.Citation20,41 Although baseline models that reflect time variations/epidemics are desirable, lack of consistent combined longitudinal carriage/disease datasets makes it difficult in practice to attempt a fully dynamic calibration.
Robust contemporary country-specific N. meningitidis carriage data with age and serogroup stratification are largely missing. In the present analyses here, we opted to employ an average well-documented EU carriage template with age stratification as given in Christensen et al.Citation1 This template, however, does not have serogroup distribution. We further use the distribution of carried isolates of N. meningitidis in 3 EU countries (the Czech Republic, Greece and Norway) between 1991 and 2000: serogroup B isolates: 32.3% (95% CI, 27–37%), serogroup C isolates: 4.8% (95% CI, 2.8–7.6%), Others isolates: 62.9% (95% CI, 58–68%),Citation30 which we apply uniformly to the age-stratified carriage template to yield synthesized serogroup- and age-stratified carriage data for model calibration.
As a reality-check, the resulting carriage data profile is in-line with an older serogroup- and age-stratified carriage data set previously used in the UK setting.Citation38
We chose 2 different EU settings for actual model calibration and validation: the UK (England and Wales) and the Czech Republic, respectively. The UK setting was chosen because there is currently significant burden of IMD caused by serogroup B, and introduction of a MenB vaccine in the routine immunization schedule is already being considered.Citation42 The Czech Republic setting was chosen as it currently does not routinely vaccinate against meningococcal disease.
Data and parameters used for simulations
The following data sets were used for model calibration: for the UK setting, age- and serogroup-stratified IMD data from national routine surveillance undertaken in England and Wales from 1998 to 2009 was made available by the Health Protection Agency;Citation43 for the Czech setting, age- and serogroup-stratified data at the population level for IMD was also extracted from a national surveillance system (data from 1999 to 2008 IMD provided by Dr. Pavla Kriz, National Reference Laboratory for Meningococcal Infections, National Institute of Public Health, Prague, Czech Republic).Citation44
For simplicity, we assumed stationary populations in the model, with underlying demography informed by available country-specific data.Citation45,46
Vaccination coverage and duration of protection were assumed age-dependent, guided by Trotter et al.Citation20, Christensen et al.Citation21 Routine infant vaccination coverage was assumed at 85%, while vaccination coverage for routine adolescent vaccination at 17 y of age was assumed at 60%. The average duration of vaccine protection against both carriage and IMD were assumed to be 15 months in individuals vaccinated before 1 y of age, 4 y in those vaccinated around 1 y of age and 10 y in those vaccinated at older ages.
In simulating potential population-level impact of vaccination, vaccine characteristics like modes-of-action and related efficacies are vital. In particular here, for assessing potential for serogroup replacement post-vaccination, the potential impact of the vaccine on carriage as well as potential cross-protection effects are key parameters. For the new MenB vaccine, little is currently known in this regard, although there are hopes for both reduction in carriage and some cross-protection. In order to keep tractability and reduce complexity, particularly given all the uncertainties involved at present, we assumed a baseline value for the MenB vaccine efficacy against serogroup B carriage acquisition of 20%, guided by the preliminary data regarding the currently available MenB vaccine.Citation11 As a hypothetical alternative, to illustrate the impact of a higher MenB vaccine efficacy against carriage, we also considered a 60% efficacy, as in Christensen et al.Citation21 The MenB vaccine efficacy against all serogroup B IMD was assumed 78% (likely an optimistic scenario) throughout the simulations.
For simulating potential cross-protection effects here, based on the data available in Claus et al,Citation17 we assumed 80% efficacy against serogroup C IMD and 50% efficacy against IMD caused by all Others (non B and C). Also, as our baseline assumption for vaccine efficacy against acquisition of serogroup B carriage was 20%, when simulating cross-protection, we also allowed for a 10% vaccine efficacy against serogroup C carriage and 5% efficacy against all Others, respectively – as a simple theoretical choice here, to still reflect a primary MenB nature of the vaccine while enabling some potential impact on other serogroups carriage. As the Others pool here is rather diverse, comprising of all N. meningitidis serogroups except those belonging to serogroups B and C, we hypothesized it would elicit the lowest theoretical cross-protective vaccine efficacy.
All our numerical simulations were performed in MATLAB 2013b, the MathWorks, Inc., Natick, Massachusetts, United States.
Disclosure of Potential Conflicts of Interest
All authors are employed by the GSK group of companies and own shares/stock options in the GSK group of companies.
Acknowledgments
All authors would like to thank Dr. Niel Hens, University of Hasselt and University of Antwerp, Belgium, for providing European contact rates based on the POLYMOD project, Dr. Pavla Kriz, National Reference Laboratory for Meningococcal Infections, Czech Republic, and Public Health England, UK (formerly the Health Protection Agency) for providing more detailed IMD data with age and serogroup stratification generated as part of the routine surveillance programs for invasive meningococcal disease in each of these countries that are not publicly available. The authors also thank Dr. Vasile Coman (XPE Pharma & Science on behalf of GSK Vaccines) who provided editorial support and Carole Desiron (Business & Decision Life Sciences on behalf of GSK Vaccines) for publication coordination and management.
Authors' Contributions
All authors participated in the design of the study and interpretation of the results. C. Hogea and A. Vyse collated the data. C. Hogea performed the analyses. A. Vyse and T. Van Effelterre reviewed the outcomes. All authors reviewed and approved the present manuscript.
Trademarks Statement
Bexsero™ is a trademark of Novartis AG.
Funding
GlaxoSmithKline Biologicals SA was the funding source and supported all costs associated with the development and the publishing of the present manuscript.
References
- Christensen H, May M, Bowen L, Hickman M, Trotter CL. Meningococcal carriage by age: a systematic review and meta-analysis. Lancet Infect Dis 2010; 10:853-61; PMID:21075057; http://dx.doi.org/10.1016/S1473-3099(10)70251-6
- ECDC, 2013. Annual epidemiological report Reporting on 2011 surveillance data and 2012 epidemic intelligence data. Available at: http://www.ecdc.europa.eu/en/publications/Publications/Annual-Epidemiological-Report-2013.pdf [Accessed 10 February 2015].
- Soriano-Gabarro M, Wolter J, Hogea C, Vyse A. Carriage of Neisseria meningitidis in Europe: a review of studies undertaken in the region. Expert Rev Anti Infect Ther 2011; 9:761-74; PMID:21905785; http://dx.doi.org/10.1586/eri.11.89
- Zahlanie YC, Hammadi MM, Ghanem ST, Dbaibo GS. Review of meningococcal vaccines with updates on immunization in adults. Hum Vaccin Immunother 2014; 10:995-1007; PMID:24500529; http://dx.doi.org/10.4161/hv.27739
- Cohn AC, MacNeil JR, Clark TA, Ortega-Sanchez IR, Briere EZ, Meissner HC, Baker CJ, Messonnier NE. Prevention and control of meningococcal disease: recommendations of the Advisory Committee on Immunization Practices (ACIP). MMWR Recomm Rep 2013; 62:1-28; PMID:23515099
- Martin NG, Snape MD. A multicomponent serogroup B meningococcal vaccine is licensed for use in Europe: what do we know, and what are we yet to learn? Expert Rev Vaccines 2013; 12:837-58; PMID:23984957; http://dx.doi.org/10.1586/14760584.2013.814862
- Sadarangani M, Pollard AJ. Serogroup B meningococcal vaccines-an unfinished story. Lancet Infect Dis 2010; 10:112-24; PMID:20113980; http://dx.doi.org/10.1016/S1473-3099(09)70324-X
- Serruto D, Bottomley MJ, Ram S, Giuliani MM, Rappuoli R. The new multicomponent vaccine against meningococcal serogroup B, 4CMenB: immunological, functional and structural characterization of the antigens. Vaccine 2012; 30 Suppl 2:B87-97; PMID:22607904; http://dx.doi.org/10.1016/j.vaccine.2012.01.033
- Trotter CL, Maiden MC. Meningococcal vaccines and herd immunity: lessons learned from serogroup C conjugate vaccination programs. Expert Rev Vaccines 2009; 8:851-61; PMID:19538112; http://dx.doi.org/10.1586/erv.09.48
- Maiden MC, Stuart JM. Carriage of serogroup C meningococci 1 year after meningococcal C conjugate polysaccharide vaccination. Lancet 2002; 359:1829-31; PMID:12044380; http://dx.doi.org/10.1016/S0140-6736(02)08679-8
- Read RC, Baxter D, Chadwick DR, Faust SN, Finn A, Gordon SB, Heath PT, Lewis DJ, Pollard AJ, Turner DP, et al. Effect of a quadrivalent meningococcal ACWY glycoconjugate or a serogroup B meningococcal vaccine on meningococcal carriage: an observer-blind, phase 3 randomised clinical trial. Lancet 2014; 384:2123-31; PMID:25145775; http://dx.doi.org/10.1016/S0140-6736(14)60842-4
- Bexsero: Summary of product characteristics. Available from: http://www.ema.europa.eu/docs/en_GB/document_library/EPAR_-_Product_Information/human/002333/WC500137881.pdf [Accessed 10 February 2015].
- Vogel U, Taha MK, Vazquez JA, Findlow J, Claus H, Stefanelli P, Caugant DA, Kriz P, Abad R, Bambini S, et al. Predicted strain coverage of a meningococcal multicomponent vaccine (4CMenB) in Europe: a qualitative and quantitative assessment. Lancet Infect Dis 2013; 13:416-25; PMID:23414709; http://dx.doi.org/10.1016/S1473-3099(13)70006-9
- Gossger N, Snape MD, Yu LM, Finn A, Bona G, Esposito S, Principi N, Diez-Domingo J, Sokal E, Becker B, et al. Immunogenicity and tolerability of recombinant serogroup B meningococcal vaccine administered with or without routine infant vaccinations according to different immunization schedules: a randomized controlled trial. JAMA 2012; 307:573-82; PMID:22318278; http://dx.doi.org/10.1001/jama.2012.85
- Santolaya ME, O'Ryan ML, Valenzuela MT, Prado V, Vergara R, Munoz A, Toneatto D, Grana G, Wang H, Clemens R, et al. Immunogenicity and tolerability of a multicomponent meningococcal serogroup B (4CMenB) vaccine in healthy adolescents in Chile: a phase 2b/3 randomised, observer-blind, placebo-controlled study. Lancet 2012; 379:617-24; PMID:22260988; http://dx.doi.org/10.1016/S0140-6736(11)61713-3
- Vesikari T, Esposito S, Prymula R, Ypma E, Kohl I, Toneatto D, Dull P, Kimura A. Immunogenicity and safety of an investigational multicomponent, recombinant, meningococcal serogroup B vaccine (4CMenB) administered concomitantly with routine infant and child vaccinations: results of two randomised trials. Lancet 2013; 381:825-35; PMID:23324563; http://dx.doi.org/10.1016/S0140-6736(12)61961-8
- Claus H, Borrow R, Taha M-K, Hong E, Gilchrist S, Findlow J, Lucidarme J, Orlandi L, Rigat F, Serruto D, et al. Potential coverage of the 4CMenB vaccine in non-B meningococci. XVIIIth International Pathogenic Neisseria Conference (IPNC). Würzburg, Germany, 2012.
- Miller E, Andrews NJ, Waight PA, Slack MP, George RC. Herd immunity and serotype replacement 4 years after seven-valent pneumococcal conjugate vaccination in England and Wales: an observational cohort study. Lancet Infect Dis 2011; 11:760-8; PMID:21621466; http://dx.doi.org/10.1016/S1473-3099(11)70090-1
- Kayhty H, Auranen K, Nohynek H, Dagan R, Makela H. Nasopharyngeal colonization: a target for pneumococcal vaccination. Expert Rev Vaccines 2006; 5:651-67; PMID:17181439; http://dx.doi.org/10.1586/14760584.5.5.651
- Trotter CL, Gay NJ, Edmunds WJ. Dynamic models of meningococcal carriage, disease, and the impact of serogroup C conjugate vaccination. Am J Epidemiol 2005; 162:89-100; PMID:15961591; http://dx.doi.org/10.1093/aje/kwi160
- Christensen H, Hickman M, Edmunds WJ, Trotter CL. Introducing vaccination against serogroup B meningococcal disease: an economic and mathematical modelling study of potential impact. Vaccine 2013; 31:2638-46; PMID:23566946; http://dx.doi.org/10.1016/j.vaccine.2013.03.034
- Melegaro A, Choi YH, George R, Edmunds WJ, Miller E, Gay NJ. Dynamic models of pneumococcal carriage and the impact of the Heptavalent Pneumococcal Conjugate Vaccine on invasive pneumococcal disease. BMC Infect Dis 2010; 10:90; PMID:20377886; http://dx.doi.org/10.1186/1471-2334-10-90
- Van Effelterre T, Moore MR, Fierens F, Whitney CG, White L, Pelton SI, Hausdorff WP. A dynamic model of pneumococcal infection in the United States: implications for prevention through vaccination. Vaccine 2010; 28:3650-60; PMID:20359560; http://dx.doi.org/10.1016/j.vaccine.2010.03.030
- Maiden MC, Ibarz-Pavon AB, Urwin R, Gray SJ, Andrews NJ, Clarke SC, Walker AM, Evans MR, Kroll JS, Neal KR, et al. Impact of meningococcal serogroup C conjugate vaccines on carriage and herd immunity. J Infect Dis 2008; 197:737-43; PMID:18271745; http://dx.doi.org/10.1086/527401
- Caugant DA, Tzanakaki G, Kriz P. Lessons from meningococcal carriage studies. FEMS Microbiol Rev 2007; 31:52-63; PMID:17233635; http://dx.doi.org/10.1111/j.1574-6976.2006.00052.x
- Krizova P, Kalmusova J, Musilek M, Felsberg J, Haugvicova R, Vlckova J. [Study of long-term and multiple carriage of Neisseria meningitidis in a healthy population using molecular biology methods]. Epidemiol Mikrobiol Imunol 2004; 53:25-36; PMID:15052832
- Martcheva M, Pilyugin SS, Holt RD. Subthreshold and superthreshold coexistence of pathogen variants: the impact of host age-structure. Math Biosci 2007; 207:58-77; PMID:17087980; http://dx.doi.org/10.1016/j.mbs.2006.09.010
- Racloz VN, Luiz SJ. The elusive meningococcal meningitis serogroup: a systematic review of serogroup B epidemiology. BMC Infect Dis 2010; 10:175; PMID:20565757; http://dx.doi.org/10.1186/1471-2334-10-175
- Bettinger JA, Deeks SL, Halperin SA, Tsang R, Scheifele DW. Controlling serogroup B invasive meningococcal disease: the Canadian perspective. Expert Rev Vaccines 2013; 12:505-17; PMID:23659299; http://dx.doi.org/10.1586/erv.13.30
- Yazdankhah SP, Kriz P, Tzanakaki G, Kremastinou J, Kalmusova J, Musilek M, Alvestad T, Jolley KA, Wilson DJ, McCarthy ND, et al. Distribution of serogroups and genotypes among disease-associated and carried isolates of Neisseria meningitidis from the Czech Republic, Greece, and Norway. J Clin Microbiol 2004; 42:5146-53; PMID:15528708; http://dx.doi.org/10.1128/JCM.42.11.5146-5153.2004
- Bogaert D, Hermans PW, Boelens H, Sluijter M, Luijendijk A, Rumke HC, Koppen S, van Belkum A, de Groot R, Verbrugh HA. Epidemiology of nasopharyngeal carriage of Neisseria meningitidis in healthy Dutch children. Clin Infect Dis 2005; 40:899-902; PMID:15736029; http://dx.doi.org/10.1086/428351
- Leimkugel J, Hodgson A, Forgor AA, Pfluger V, Dangy JP, Smith T, Achtman M, Gagneux S, Pluschke G. Clonal waves of Neisseria colonisation and disease in the African meningitis belt: eight- year longitudinal study in northern Ghana. PLoS Med 2007; 4:e101; PMID:17388665; http://dx.doi.org/10.1371/journal.pmed.0040101
- Frosch M, Maiden MCJ. Handbook of meningococcal disease : infection biology, vaccination, clinical management Germany: Weinheim : Wiley-VCH, 2008.
- Mossong J, Hens N, Jit M, Beutels P, Auranen K, Mikolajczyk R, Massari M, Salmaso S, Tomba GS, Wallinga J, et al. Social contacts and mixing patterns relevant to the spread of infectious diseases. PLoS Med 2008; 5:e74; PMID:18366252; http://dx.doi.org/10.1371/journal.pmed.0050074
- Goeyvaerts N, Hens N, Ogunjimi B, Aerts M, Shkedy Z, Damme PV, Beutels P. Estimating infectious disease parameters from data on social contacts and serological status. J Royal Statist Soc Series C: Applied Statistics 2010; 59:255-77; http://dx.doi.org/10.1111/j.1467-9876.2009.00693.x
- Wallinga J, Teunis P, Kretzschmar M. Using data on social contacts to estimate age-specific transmission parameters for respiratory-spread infectious agents. Am J Epidemiol 2006; 164:936-44; PMID:16968863; http://dx.doi.org/10.1093/aje/kwj317
- Lipsitch M, Colijn C, Cohen T, Hanage WP, Fraser C. No coexistence for free: neutral null models for multistrain pathogens. Epidemics 2009; 1:2-13; PMID:21352747; http://dx.doi.org/10.1016/j.epidem.2008.07.001
- Trotter CL, Gay NJ, Edmunds WJ. The natural history of meningococcal carriage and disease. Epidemiol Infect 2006; 134:556-66; PMID:16238823; http://dx.doi.org/10.1017/S0950268805005339
- Ogunjimi B, Hens N, Goeyvaerts N, Aerts M, Van Damme P, Beutels P. Using empirical social contact data to model person to person infectious disease transmission: an illustration for varicella. Math Biosci 2009; 218:80-7; PMID:19174173; http://dx.doi.org/10.1016/j.mbs.2008.12.009
- Sutton KL, Banks HT, Castillo-Chavez C. Public vaccination policy using an age-structured model of pneumococcal infection dynamics. J Biol Dyn 2010; 4:176-95; PMID:22876985; http://dx.doi.org/10.1080/17513750903023715
- Coen PG, Cartwright K, Stuart J. Mathematical modelling of infection and disease due to Neisseria meningitidis and Neisseria lactamica. Int J Epidemiol 2000; 29:180-8; PMID:10750621; http://dx.doi.org/10.1093/ije/29.1.180
- Wise J. Meningitis B vaccine to be introduced in UK after U turn on its cost effectiveness. BMJ 2014; 348:g2327; PMID:24665138; http://dx.doi.org/10.1136/bmj.g2327
- Health Protection Agency. Laboratory confirmed cases of all invasive meningococcal disease by serogroup, age and epidemiological year, England and Wales. Available at: https://www.gov.uk/government/collections/meningococcal-disease-guidance-data-and-analysis [Accessed 10 February 2015].
- Hogea C, Effelterre TV, Vyse A, Kriz P, Soriano-Gabarró M. Neisseria meningitidis: A baseline dynamic transmission model for the Czech Republic. 28th Annual ESPID Meeting; Nice, France; 2010.
- UK's Office for National Statistics. Population Estimates by Age and Sex. Available at: http://www.ons.gov.uk/ons/taxonomy/index.html?nscl=Population +Estimates+by+Age+and+Sex [Accessed 10 February 2015].
- Czech Statistical Office. Czech Demagraphic Handbook 2007. Available at: http://csugeo.i-server.cz/csu/2008edicniplan.nsf/engpubl/4032-08-2007 [Accessed 10 February 2015].