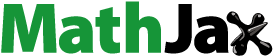
Abstract
Here a novel antibacterial nanocomposite was developed for combination cancer therapy. The synthesized nanocarrier was characterized by FTIR, 1H NMR, thermogravimetric analysis (TGA), and FESEM-EDX. Its antibacterial activity was assessed by determining minimum inhibitory concentration (MIC) values. Doxorubicin (DOX) and methotrexate (MTX) conjugation with nanocarrier sustained the release of both drugs with apparent pH-triggered manner. Co-administration of DOX with MTX leads to an efficient anticancer performance to MCF7 cell lines verified by qRT-PCR and MTT assay tests. It was concluded that this novel drug delivery vehicle makes antibacterial and anticancer therapeutic processes proceed spontaneously, representing more efficient drug delivery system in nanomedicine.
Introduction
Nanotechnology has been broadly used in the expansion of new tactics for drug delivery and cancer therapy (Hu et al. Citation2010, Laurent et al. Citation2010). Particularly nano-systems including stimulus-responsive segments have notable capacities which allow them to bypass biological barriers and reach targeted intracellular drug delivery (Chang et al. Citation2011, Jalil Karnoosh-Yamchi et al. Citation2015, Liu et al. Citation2014, Roya Salehi et al. Citation2009, Zhang et al. Citation2007). The body’s protection mechanisms are extremely complex and once triggered; they follow numerous pathways in cascade. Consequently, by using single drug molecules, targeting the whole cascade will be complex (Castro et al. Citation2012). Therefore smart carriers can increase the permeance and survival of drugs in tumors and decrease the side effect and toxicity related with most anti-cancer drugs (Schleich et al. Citation2013, Wang et al. Citation2015, Xiong et al. Citation2011). Unlike normal tissues which have neutral pH, tumor tissues show an acidic pH, therefore, pH responsive nanocarriers have been most extensively used to design controlled drug release systems in cancer therapy (Jin et al. Citation2013, Liu et al. Citation2009, Wei et al. Citation2009). Therefore pH-sensitive drug carriers can be a key part for designing controlled anticancer drug delivery systems (Argentiere et al. Citation2011).
Resistance of cancer cells to chemotherapy drugs is the most challenging part of cancer therapy that could be omitted when multiple drugs are delivered simultaneously; though, different drugs target different components of the cancer cell. The combined therapy from more than one anticancer drug can produce the optimal therapy effect (Ahmed et al. Citation2006, Askari et al. Citation2015, Kang et al. Citation2015, Lee and Nan Citation2012, Ouahab et al. Citation2014, Shen et al. Citation2013, Singh et al. Citation2011). Therefore, investigation of novel systems for ordered multiple-drug delivery in a single formulation is helpful in terms of development of future smart DDSs (Guo et al. Citation2010, Shen et al. Citation2013, Singh et al. Citation2011, Zhu et al. Citation2011).
On the other hand, the body's immune system under chemotherapy become weak and susceptible to infectious diseases, so during chemotherapy for these patients, antibiotics are prescribed. Regardless of great amount of antibiotics and chemotherapeutics accessible for medical use, meanwhile the growing number of multi-drug resistant microbial pathogens produced in the last decades revealed an extensive medical requirement for novel antimicrobial agents (Rizzotto Citation2012). The greater part of antibiotics acts on intracellular targets, leaving the bacterial morphology intact (Ng et al. Citation2014, Schwartz et al. Citation2012). There is an actual perceived requirement for the discovery of new compounds endowed with antimicrobial activity that are less sentient to resistance as they function by microbial membrane disruption. Therefore, new antimicrobial agents and nanotechnological compounds have to be produced to overcome resistant bacterial diseases. Polymer chemistry has various applications in medical field in modern days such as using as antibacterial agent (Eriksen and Kassem Citation1992). Several kinds of distinctive materials, such as antimicrobial peptides (AMPs) (Ng et al. Citation2014), synthetic cationic polymers (Engler et al. Citation2012, Muñoz-Bonilla and Fernández-García Citation2012), quaternized amine containing polymers (synthesized from methacrylate monomers containing quaternary ammonium, like quaternary ammonium salts of diethylaminoethyl methacrylate (DEAEMA) (Carmona-Ribeiro and de Melo Carrasco Citation2013, He et al. Citation2011, Mohammed et al. Citation2010, Vigliotta et al. Citation2012), guanidine derivatives, and polymeric guanidine salts like polyhexamethylene biguanide (PHMB) (Zhang et al. Citation1999) and antimicrobial nanoparticles (Rizzotto Citation2012), have emerged as potential substitutes for conventional antibiotics. The superiority of the novel antimicrobial agents is their different mechanism of action, which is distinct from those of well-known classes of antimicrobial agents that most of the clinically important pathogens are currently resistant (Rizzotto Citation2012). Doxorubicin (DOX) is a cationic anticancer drug that has been one of the most frequently employed chemotherapeutic drugs in diverse kinds of cancer. Although it reveals high effectiveness, DOX is also toxic to healthy organs, causing serious side effects (Salehi et al. Citation2014). Methotrexate (MTX) is a folic acid analog cancer drug which acts as an inhibitor 416 of DHFR to stop the tetrahydrofolate synthesis and causes cell death. The MTX-silica conjugated nanoparticles can amplify the affinity to FR (Alidadiyani et al. Citation2014, Jin et al. Citation2013). DOX and/or MTX formulated nanoparticles are thought to progress the drug efficacy, while reducing side effects. Thus, the delivery system efforts have changed toward more controlled and stable systems like polymeric nanoparticles. Recently some efforts were done to build antibacterial nanocarriers such as nanocapsules/vesicles as delivery system for anticancer drug (Wang et al. Citation2015, Zhou et al. Citation2013).
However, only one research was reported on antibacterial smart polymeric dual-anticancer drug delivery system (DDDS) that was done in our team previous work (Fatemeh Bazmi Zeynabad et al. Citation2015). The aim of this study was to synthesize a novel multi-potent smart antibacterial nanocarrier for dual anticancer drug delivery purposes. To achieve this goal, novel multi block silica based polymeric nanocarrier anticipating the anti-bacterial properties was designed and developed as dual anticancer drug delivery system. The chemical structure, stimuli responsive co-delivery of anticancer drugs, anti-bacterial, and anti-tumoral properties of the developed system were fully characterized.
Experimental
Materials and methods
N-Isopropylacrylamide (NIPAAm) (97%, Sigma-Aldrich, Schnelldorf, Germany) was refined by recrystallization in n-hexane and then vacuum dried. Methacrylic acid (MAA) and N,N-dimethylaminoethyl methacrylate (DMAEMA) (Sigma-Aldrich Co., Steinem, Germany) were distilled under reduced pressure in nitrogen atmosphere. Ethylenediamine (EDA) (≥99%) was purchased from Merck Co. (Readington, NJ). Cyanoguanidine, tetraethyl orthosilicate (TEOS), 3-amino propyltrimethoxysilane (3-ATMS), (3-chloropropyl) trimethoxysilane, and benzoyl peroxide were purchased from Sigma-Aldrich (Steinem, Germany) and used as received. Doxorubicin hydrochloride was purchased from Sobhan Pharmaceuticals, Rasht, Iran. Methotrexate (vial 50 mg/5 mL) was purchased from Koçak Farma, Istanbul, Turkey. MTT (3-(4, 5-dimethylthiazol-2-yl)-2, 5-diphenyltetrazolium bromide) and other biological reagents were obtained from Invitrogen (Carlsbad, CA). All other reagents were of analytical grade and used with no further purification.
Synthesis
Synthesis of amino functionalized modified silica nanoparticles (AMSNs)
Silica nanoparticles were prepared according to the sol–gel method (Rasouli et al. Citation2013, Rasouli et al. Citation2014). In a 250 mL round bottom flask, 750 μL (10 mmol) ammonia solution (37%) and 1.98 g (10 mmol) water were added to 100 mL absolute methanol. The solution was stirred vigorously for 10 min. Then 10.41 g (50 mmol) tetraethoxysilane (TEOS) was added dropwise. The solution was stirred for 10 min. After that, 1.02 g (5.7 mmol) (3-aminopropyl) trimethoxy silane was added dropwise. The final solution was stirred for 3 d at ambient temperature. Then modified silica nanoparticles were precipitated with n-hexane and separated through twice centrifugation at 12,000 rpm (Sigma 1–15 pK, Steinem, Germany). The obtained modified silica nanoparticles were freeze dried (Lyotrap Plus, LTE Scientific Ltd., Oldham, UK) to give the white powder.
Synthesis of N,N-dimethylaminoethylmethacrylate based ionic liquid (cationic-IL) monomer (DMAEMAQ-IL)
The synthesis method of DMAEMAQ-IL was reported in our previous work (Salehi et al. Citation2014). First N,N-dimethylaminoethylmethacrylate (4 g, 25.091 mmol) and 3-chloropropyl trimethoxysilane (6.042 g, 25.091 mmol) were refluxed for 3 d at 80 °C. The suspension was filtered off and the solvent evacuated. By addition of 150 mL dry dichloromethane, a precipitate appeared and it was filtered off under argon atmosphere. The product was then separated by distillation at 150 °C under vacuum (1 mbar) and cationic-IL monomer was obtained with a honey-like consistency at room temperature in 98% yield. In this way, functionalized quaternary amine containing ionic liquid monomer was synthesized.
Synthesis of novel cationic, dual pH/thermo-sensitive P(NIPAAm-MAA-DMAEMAQ-IL) terpolymer
The synthesized protocol of P(NIPAAm-MAA-DMAEMAQ-IL) terpolymer was described in detail in our previously work (Salehi et al. Citation2014). NIPAAm, MAA, and functionalized DMAEMAQIL (ionic liquid cationic monomer) monomers were dissolved in 1,4-dioxane as solvent with molar feed ratio of 20, 60, and 20, respectively (monomers molar ratio was different with our previous work). Then benzoyl peroxide was added as a free-radical initiator. The polymerization was performed in a 50 mL three-neck flask. The reaction mixture was magnetically stirred and kept for 10 min under argon flow. Then the tubes were sealed under argon atmosphere and the mixture polymerized for 24 h at 70–80 °C. The product was filtered and washed with n-hexane and dried in vacuum and kept in desiccator for next use.
Synthesis of polyethylene cyanoguanidine hydrochloride (PECGC)
Polyethylene cyanoguanidine hydrochloride was prepared with condensation polymerization of ethylene diamine and excess amount of cyanoguanidine (dicyanodiamide) in the presence of hydrochloric acid. The mixture was polymerized for 24 h at 80–85 °C. After completion of the reaction, product was precipitated in acetone and n-hexane and was collected by centrifugation and finally washed with diethyl ether and acetone. The final product was vacuum dried and stored in desiccators for next use.
Synthesis of cationic and dual thermo/pH responsive silica nanocomposites P(NIPAAm-MAA-DMAEMAQ)&MSNs& PECGC
P(NIPAAm-MAA-DMAEMAQ)&MSNs&PECGC was prepared in two step. First, AMSNs (1.5 g) was reacted with P(NIPAAm-MAA-DMAEMAQ) (0.75 g) via methoxy groups of DMAEMAQ with hydroxyl groups of AMSNs in DMSO as a solvent. Second, P(NIPAAm-MAA-DMAEMAQ)&AMSNs was reacted with PECGC (0.75 g) in the presence of hydrochloric acid at 80–85 °C for 24 h. Final product was vacuum dried and stored in desiccators.
Instrumentation
FTIR spectroscopy
The chemical structures of the P(NIPAAm-MAA-DMAEMAQ-IL), PECGC, and P(NIPAAm-MAA-DMAEMAQ)&MSNs&PECGC were studied by FTIR spectroscopy. All samples were mixed with KBr and were pressed to disk. Infrared (IR) spectra of the samples were scanned in the range from 400 to 4000 cm−1 and recorded on a Fourier transform IR spectrometer (Equinox 55 LS 101, Bruker, Karlsruhe, Germany).
1H NMR spectroscopy
1HNMR spectra of P(NIPAAm-MAA-DMAEMAQ-IL) and PECGC were recorded in d6-DMSO solvent on a Bruker DRX-400 spectrometer (Karlsruhe, Germany) with tetramethylsilane as internal reference.
SEM studies
The surface morphology, average diameter, particle size, and pore volume of P(NIPAAm-MAA-DMAEMAQ)&MSNs&PECGC polymeric nanocomposite were assessed by FESEM-EDX, S4160 Hitachi, Tokyo, Japan. The powder sample was propagated on a SEM stub and sputtered with gold. Particle size was achieved by measuring the diameters of at least 100 particles revealed through SEM, using image analysis software (Image-Pro plus 4.5; Media Cybernetics, Silver Spring, MD).
Thermogravimetric analysis (TGA) studies
Thermogravimetric analysis of P(NIPAAm-MAA-DMAEMAQ)& MSNs&PECGC polymeric nanocomposite was carried out with a Mettler-Toledo model 822 instrument (Columbus, OH). Decomposition profiles of TGA were recorded under a nitrogen atmosphere at a heating rate of 10 °C per minute from 50 to 950 °C.
Preparation of inoculum
The standard strain of P. aeruginosa (ATCC: 27853), Escherichia coli (ATCC: 25922), and Candida albicans (ATCC: 10231) used in this project were obtained in lyophilized form from Institute of Pasteur, Iran. They were activated by culturing in sterile Mueller Hinton agar for 48 h at 37 °C. A single colony from grown plate was transferred into (Mueller Hinton) broth and incubated over night at 37 °C. As a result, following incubation time the cells were collected by centrifugation at 4000 rpm for 10 min and washed tree times and re-suspended in Ringer solution to provide an optical density of around 0.1 at 540 nm with a spectrophotometer (Coleman, Golden, CO) or bacterial concentration around 108 CFU/mL.
Evaluation of the antimicrobial activity
The antimicrobial activities of prepared nanoformulations were studied by minimum inhibitory concentration (MIC) determination against standard strain of Pseudomonas aeruginosa (ATCC: 27853), Escherichia coli (ATCC: 25922), and Candida albicans (ATCC: 10231) using broth dilution method. Briefly, bacterial inoculum in Mueller–Hinton Broth medium in the concentrations equal to 0.5 of McFarland standard was added to serially diluted NPs suspension with different concentrations (0.04–80 mg.mL−1). After 24 h incubation at 37 °C, from the content of the tubes, streak cultures were made onto the Mueller–Hinton agar plates. The first concentration with no sign of bacterial growth on plates was considered as MIC. All experiments were performed in three separate occasions.
Drugs loading and release
Predetermined amount of P(NIPAAm-MAA-DMAEMAQ) &AMSNs&PECGC nanocomposite was ultrasonically dispersed in the DOX solution for 5 min. Then, it was magnetically stirred for 1 d under dark conditions. Then unloaded DOX was removed by centrifugation and several washing. After that, MTX was added to DOX&P(NIPAAm-MAA-DMAEMAQ) &AMSNs&PECGC mixture and dispersed with the help of ultrasonication (Sonics Vibra cell, Model: VCX 130 PB, Newtown, CT) for 5 min. Then unloaded MTX was eliminated by centrifugation and several washing. The ratio of carrier to drug was 5 to 1 for sum of both drugs. The final carrier/drug (DOX + MTX) ratio was 5 to 1 and also DOX to MTX molar ratio was 5/1. The mixture was stirred at ambient temperature for alternative 24 h under dark conditions. MTX&DOX-loaded nanocomposite was collected by centrifugation at 14,000 rpm for 10 min and vacuum dried over night at ambient temperature and stored in desiccators. Drug loading content and drug encapsulation efficiency of nanocomposites were calculated by the following equations:
The powdered MTX&DOX-loaded nanocomposite was re-dispersed in 2 mL buffer solutions and transferred into a dialysis bag (MWCO 12,000 Da, Sigma-Aldrich, St. Louis, MO). The bag was afterward located in an 8 mL of the same buffer solution of dialysis bag at 37 °C. The medium was placed into incubator and stirred constantly throughout the release study. At suitable time periods, the total solution (8 mL) was collected and meanwhile, 8 mL of the same fresh buffer solution was added and determined amounts of released DOX and MTX in the original buffer solutions. Three different pH values of 4, 5.8, and 7.4 were used to simulate the different biological environments. The HPLC-UV method was used for measurement amounts of DOX and MTX released at wavelength of 254 and 303 nm as previously described (Salehi et al. Citation2014, Citation2015). In order to reduce error, all measurements were repeated twice. Percentage of drug released from the nanocomposite was obtained using the following formula:
Cell culture and in vitro cytotoxicity assay
Human breast adenocarcinoma MCF7 cell lines were used as the target cells for evaluation of the antitumor activity of the DOX@MTX incorporated in the nanocarriers and cytotoxicity of nanocarriers using MTT assay as previously described (Rasouli et al. Citation2014). In this context, different concentration (20, 10, 5, and 2.5 μg/mL) of drug-loaded nanocarriers and pure drug was prepared in fresh cell growth medium and administered into the 96-well microplates with a cell density of 5 × 103 cells per well with different time's manners. Drug-free nanocarriers with different concentration (1000 and 500 μg/mL) were seeded to investigate the cytotoxicity of nanocarrier. After incubation for a predetermined time, a microplate was withdrawn for MTT assay. The MTT assay was as follows: 20 μL of MTT solution (5 mg/2 mL) in PBS (pH 7.4) was added to each well. The incubation was continued for another 4 h and then the solution was removed carefully from each well. After treating the cells with Sorenson buffer, the optical density of each well was read using a microplate reader (Multiskan MK3, Thermo Electron Corporation, Minneapolis, MN) at a wavelength of 570 nm, and growth inhibition was calculated. All of the tests were performed in duplicate and statistical analyses were carried out using SPSS 15 (SPSS, Chicago, IL); P < 0.05 was considered significant.
qRT-PCR
The MCF-7 cells were treated with different doses (10, 100, and 250 μg.mL) of both DOX@MTX-loaded P(NIPAAm-MAA-DMAEMAQ)&MSNs&PECGC (ZD) and DOX@MTX (D) for 48 h in 37 °C, 5% CO2, and humidified incubator.
The total RNA was extracted from each treatments including DOX@MTX-loaded P(NIPAAm-MAA-DMAEMAQ)&MSNs& PECGC (ZD) and DOX@MTX (D) and control non-treated MCF-7 cells, using MIRCURY RNA Isolation Kit Cell and Plant (Exiqon, Vedbæk, Denmark) then reverse transcribed into cDNA using RevertAid cDNA synthesis kit (Fermentas, Waltham, MA). Real-time PCR was performed using a Jena Bioscience Master SYBR Green on a Rotor gene 6000 (Corbett, Mortlake, NSW, Australia) system. Sequences of the cyclin D1 and GAPDH primers for amplification of cDNAs are summarized in . GAPDH was used as an internal control. All experiments were performed in triplicate. ΔΔCt method was used for data analysis.
Table 1. Primer sequences for qRT-PCR.
Statistical analysis
All data are shown as mean ± SD from at least three independent experiments. The t-test and ANOVA were used for examining significance (P < 0.05).
Results and discussion
The synthesized root of novel multifunctional P(NIPAAm-MAA-MAEMAQ)&MSNs&PECGC carrier are shown schematically in .
Characterization
Hydrogen nuclear magnetic resonance
shows 1HNMR spectra of P(NIPAAm-MAA-DMAEMAQ) in DMSO, in which the characteristic peaks of two component of NIPAAm were observed at d (ppm) = 3.94 ((CH3)2–CH) and 1.04 ((CH3)2CH). The signal attributed to MAA and DMAEMAQ methyl group appeared at d (ppm) = 1.78 (C‐CH3). The proton signals of DMAEMA moiety in polymer appeared at 3.6, 2.52, and 2.6 which attributed to (CH2‐O‐C=O), (CH2‐CH2‐O‐C=O) and ((CH3)2‐N‐), respectively. 3.55 (O‐CH3) and 0.65 (Si‐CH2) are attributed to the protons of trimethoxysilylpropyl moiety and the signals of N‐CH2‐CH2 and N‐CH2‐CH2 were emerged in 2.53 and 1.9, respectively. Appeared signals about 7.2 and 12 ppm appertain to NH and OH respectively in composite structure and signals at 1.3–1.9 (ppm) were pretending the other protons of polymer backbone. shows 1HNMR spectra of PEBGC in DMSO, in which the characteristic peak of (CH2)2 appeared in 3.075. The signals of NH groups were observed at δ (ppm) 7.33 (1H, NH=C‐NH‐C=NH), 8.13 (2(H, (CH2)2‐NH‐C=NH)), and 8.48 (C=NH). The HNMR spectra of P(NIPAAm-MAA-MAEMAQ) were reported in our previous work.
Fourier transform IR spectroscopy
The chemical structures of the AMSNs (), P(NIPAAm-MAA-DMAEMAQ) (), PECGC (), and P(NIPAAm-MAA-DMAEMAQ)&MSNs&PECGC () were studied by FTIR spectroscopy. In , the shoulder at 3500 cm−1 could be assigned to the stretching vibrations of Si‐OH groups in the structure of amorphous SiO2. The presence of the Si‐OH group is proved as bonded water. The very strong and broad IR band at 1000–1150 cm−1, band at 926 cm−1, and band at 810 cm−1 are usually assigned to the Si‐O‐Si asymmetric stretching vibrations, out-of-plane bending vibrations of free-silanol O‐H groups and Si‐O‐Si symmetric stretching vibrations, respectively. Whereas the IR band at 514 cm−1 is due to O‐Si‐O bending vibrations. The C‐H stretching vibration of the aliphatic section (ATMS) is manifested through the strong peak at 2890 cm−1. The peak at 1550 cm−1 was attributed to bending vibrations of NH2 groups and broad peak at 3350 was attributed to NH2 of ATMS and Si‐OH groups. indicates that the broad absorption bands at 1118 cm−1 and 1735 cm−1 can be attributed to the stretching vibration of Si‐O and ester carbonyl stretching vibration of DMAEMAQ moiety of copolymer. The C‐H stretching vibration of the aliphatic section and stretching vibration of OH and NH are manifested through strong peaks at 2950 cm−1 and 3400 cm−1. Also three peaks near (1633) cm−1, (1675) cm−1, and 1350 cm−1 were attributed to the stretching vibration of C=O of amide at NIPAAM, carboxylic acid in MAA moiety of copolymer and stretching vibration of C‐N. reports that there are five characteristic peaks for PECGC. The broad absorption band at 3330 cm−1 was attributed to the stretching vibration of NH. Appeared peak in 2150, 2190 cm−1 is related to CN. The C=N stretching frequency is found at 1680 cm−1. The bending frequency of NH is observed at about 1640 cm−1 and the stretching vibration of C‐N appeared at about 1340 cm−1. Meanwhile, there is also a characteristic peak at about 1556 cm−1, which indicates the bend vibration of NH2+. shows the IR spectroscopy of final carrier. In , the presence of all characteristic peaks are represented in and the elimination of CN peak, demonstrated PECGC and P(NIPAAm-MAA-DMAEMAQ) loading on the MSNs.
Morphology
presents the SEM micrographs illustrating the morphology and size of P(NIPAAm-MAA-DMAEMAQ)&MSNs& PECGC. The particles sizes were in the range of 30–100 nm with uniform size and morphology. EDX spectrums () of P(NIPAAm-MAA-DMAEMAQ)&MSNs&PECGC confirmed the presence of silica, nitrogen, carbon, and all other elements that were predicted to attend at the structure of final nanocomposite.
Thermal analysis
The TGA of P(NIPAAm-MAA-DMAEMAQ)&MSNs&PECGC nanocomposite was performed (). TGA thermographs of nanocomposite exhibited four-stage degradation behavior. The first minor weight loss below 150 °C was associated with the elimination of water and residual organic solvents, the second stage at the temperature range of 150–300 °C (around 50% weight loss) related to the cleavage of pending organic units from the polymer backbone and departure of copolymer blocks, the third stage at the temperature range of 300–630 °C revealed polymer degradation and the fourth at the temperature range of 630–900 °C was associated with the dehydroxylation of silanol groups on poly-SiO2 samples and formation of siloxane groups.
Drug loading and release studies
The drug loading experiments were performed at pH 7, in which free surface silanol groups of MSNs (pKa =6.2–6.8) and carboxylic acid groups of grafted PMAA in the carrier are deprotonated (pKa =6) (Rasouli et al. Citation2014) and thereupon negatively charged. On the other hand, at pH 7, amine functional groups of EDA part of NPs (pKa 7.6 and 10.7) (Zuidema Citation1985) and DOX (pKa = 8.3) are in protonated state and have positively charged (Salehi et al. Citation2014). The DOX was loaded by the composite nanoparticles due to the presence of electrostatic attraction between positively charged DOX with the negatively charged carrier due to deprotonated carboxylic and silanol groups in composite microspheres. At pH of 7, the net charge of MTX was negative (pKa 3.8, 4.8, and 5.6) (Rasouli et al. Citation2014) which leads to an electrostatic attraction among MTX and the cationic amino segment of DMAEMAQ as well as positively charged EDA part in silica-NPs. Hydrogen bonding is another way interaction between both drugs with nanocomposite. The drug loading results indicated that conjugation of DOX in modified NPs did not affect the loading capacity of nanoparticles for conjugation with second drug MTX and both the drugs were loaded separately with more than around 95.8 ± 3.2% encapsulation efficiency and 19.4 ± 1.3%. In addition, our developed delivery system needed low amount of carrier for efficient drug delivery because of high loading efficiency values. In this report, the simultaneous loading of multiple anticancer drugs, DOX and MTX, was obtained at pH 7 by electrostatic attraction and hydrogen bonding between carrier () and drugs to explore potential combination therapy applications.
Figure 5. (a) Scheme of simultaneous loading of multiple anticancer drugs including doxorubicin (DOX) and methotrexate (MTX) on pH-responsive P(NIPAAm-MAA-DMAEMAQ)&MSNs&PECGC nanoparticles and (b) cumulative release of methotrexate (MTX) and doxorubicin (DOX) from DOX@MTX loaded pH-responsive P(NIPAAm-MAA-DMAEMAQ)&MSNs&PECGC nanoparticles at different pH (4, 5.8, and 7.4) 37 °C.

The sustained release of drugs from the nanoparticles is an essential requirement for cancer therapy (Singh et al. Citation2011). Due to the mild acidic environment of most cancer tissues, pH-sensitive NPs are in the center of interests in cancer research. shows the drug release curves of dual-drug loaded smart P(NIPAAm-MAA-MAEMAQ)&MSNs&PECGC system in a simulative normal body fluid (50 mM PBS, pH 7.4) and an acidic environment (50 mM PBS, pH 5.8 and 4) for 25 d at 37 °C. The release profile for P(NIPAAm-MAA-DMAEMAQ) &MSNs&PECGC NPs was studied at three stages: an initial small burst release (stage I), followed by a decelerating release (stage II) and a constant release (stage III). The release was found to follow a diffusing way at the initial stages of the release (first 24 h), that around 5–15% of DOX and MTX were released no matter in buffer with natural or mild acidic pH. In the initial stage at 37 °C and pH 7.4, a negligible DOX was released (around 4%) from P(NIPAAm-MAA-DMAEMAQ) &MSNs&PECGC NPs. There was a high concentration gradient between the hydrogel surface and the release medium. Considering that it was the driving force for drug diffusion at first 24 h. Also the part of drugs that physically loaded on NPs released in this stage. After the burst period, a significant pH-dependent sustained release response could be observed for both drugs at stage II. In this stage, the part of DOX and MTX started to release that loaded by pH-dependent ionic interaction and hydrogen bonding in NPs. The dual drug released amount reached 40–85% between 24 and 260 h (stage II). After 260 h, the amount of drugs released from the NPs was at a constant and increased only 5–10%. There was a slight difference in the release profile and rate of the two drugs at pH values of 5.8 and 7.4. At the end of the release study, the percentage of MTX and DOX was only 60% and 40%, respectively. Furthermore, no significant differences were found in amounts and profile of released drugs at both mentioned pHs (5.8 and 7.4). If we decrease the pH value, an obvious increase in the drug release rate can be observed. The release profile of both drugs, MTX and DOX, was very similar at pH 4, the ultimate release percentage of both drugs reached about 100% at the end of study. MTX and DOX showed initial slow release within the first day and subsequent stable quasi linear release. The DOX release was considerably slow with an initial burst of about 4% within 24 h and reached 40% in 25 d. The mutual interactions between the drugs and the P(NIPAAm-MAA-DMAEMAQ)&MSNs&PECGC NPs influenced the differences in the release profiles for the drugs. In fact, at pH 5.8 and 7.4, the interactions between drugs and NPs greatly restricted the remaining drug from releasing. At pH =4, due to the repulsive force between drugs and NPs, the multilayer coating became loose and both drugs released from the DDDS. Thus, the developed dual-drug delivery system can provide an excellent tumor drug delivery character responding to mildly acidic environments.
In vitro antibacterial efficiencies of novel developed nanoparticles
The multifunctional P(NIPAAm-MAA-DMAEMAQ)&MSNs& PECGC NPs synthesized here have cationic quaternary amine and poly biguanide blocks, both of them are known to have antibacterial property (He et al. Citation2011, Mohammed et al. Citation2010, Vigliotta et al. Citation2012, Zhang et al. Citation1999). Therefore we expected that the novel developed nanoparticles here had potent antibacterial ability. To test this hypothesis, we performed the antibacterial test of novel developed nanoparticles against standard strains of Pseudomonas aeruginosa (ATCC: 27853), Escherichia coli (ATCC: 25922), and Candida albicans (ATCC: 10231).
The mentioned bacteria are treated with P(NIPAAm-MAA-DMAEMAQ)&MSNs&PECGC NPs of various concentrations (). The results indicated that the novel developed nanoparticle had antibacterial activity. A blank sheet without polymer vesicles was used as the control to compare the antibacterial activity. The antibacterial photographs were taken after incubation for 48 h at 37 °C. depicts the results of streak cultures on the surfaces of Mueller–Hinton agar plates, the first concentration with no sign of bacterial growth on plates considered as MIC. As shown in , dense bacterial colonies were observed in the control sample without any vesicle treatment, MIC values of novel developed P(NIPAAm-MAA-DMAEMAQ) &MSNs&PECGC nanoparticles were measured to be 10, 20, and 40 mg.mL−1 for Escherichia coli, Pseudomonas aeruginosa, and Candida albicans, respectively. Although the antibacterial ability of these compounds are not as severe as well known antibiotics but its antibacterial ability (10, 20, and 40 mg/mL depended on bacteria type) was comparable with repeatedly reported antibacterial synthetic molecules and polymeric compounds with MIC value in the range of 2.5–100 mg/mL (Alamri et al. Citation2012, Kenawy et al. Citation1998, Varadaraji et al. Citation2010). The resultant products are effective in controlling the growth of bacteria. It is also found that the MIC of novel developed nanoparticle as antimicrobial agent varies based on the microorganism species.
Figure 6. (a) Streak cultures on the surfaces of Mueller–Hinton agar plates, the first concentration with no sign of bacterial growth on plates considered as minimum inhibitory concentration (MIC), (b) cell growth inhibition rates by different concentration of MTX@DOX@Carrier and MTX@DOX on MCF7 cell lines after 72 h incubation, (c) cell viability of blank P(NIPAAm-MAA-DMAEMAQ)&MSNs&PECGC nanoparticles on adipose tissue derived mesenchymal stem cells after 72 h, and (d) the expression levels of cyclin D1 in each treatment of the MCF-7 cells were studied using qRT-PCR. GAPDH served as internal control. The cyclin D1 was down regulated after drug/polymer treatments, the expression levels were obviously smaller in lower doses of drug/polymer. *P < 0.05, **P < 0.01, and ***P < 0.001.

Table 2. Results of minimum inhibitory concentration (MIC) determination (mg.mL−1).
In vitro cell assay and quantitative real-time PCR (qPCR)
In order to study the anti-proliferation efficacy of the DOX@MTX-loaded multifunctional P(NIPAAm-MAA-DMAEMAQ)&MSNs&PECGC NPs, MCF7 breast cancer cells were exposed to free DOX@MTX or DOX@MTX@ P(NIPAAm-MAA-DMAEMAQ)&MSNs&PECGC with concentrations ranging from 2.5 to 50 μg/mL for 72 h, and the cell viabilities were quantified by an MTT assay (). This dual anticancer drug, DOX@MTX cytotoxicity in free form or loaded in NPs was increased in dose-dependent manner in which lower cancer cell viability was achieved by decreasing drug concentration (with IC50 value of 25 μg/mL). Hence, DOX@MTX antitumor efficacy was maintained while encapsulated in nanoparticles. The blank carrier of P(NIPAAm-MAA-DMAEMAQ)&MSNs&PECGC NPs showed very low cytotoxicity on the MCF7 cells even at high concentration (1000 μg.mL−1) with cell viability of 83%. Significant growth inhibition of MCF7 cells was observed when the cells were treated with either the suspension of DOX@MTX-loaded P(NIPAAm-MAA-DMAEMAQ)&MSNs&PECGC NPs or pure DOX and MTX. Only 28.5% and 16.6% of the cells remained viable at a DOX@MTX dose of 2.5 μg.mL after incubation with free DOX@MTX and DOX@MTX-loaded nanocomposite, respectively. The cell viability of dual drug-loaded NPs was much lower than that of free drugs, which demonstrated that DOX@MTX-loaded nanocomposite possessed higher growth inhibition property than free drugs, and the released DOX@MTX from the NPs still held high anticancer activity. We tested biocompatibility of the different concentrations of our nanocarrier (0, 10, 50, 100, and 300 μg/mL) on adipose tissue derived mesenchymal stem cells which confirmed very low cytotoxic effects after 72 h ().
Cyclin D1 plays an important role in G1 phase of cell cycle progression. Forced expression of cyclin D1 results in reduction in cell size, shortens the G1 phase in cell cycle, and leads to the oncogenic transformation of the cells (Alao Citation2007). In addition to regulation of cell proliferation, cyclin D1 controls senescence, tumorigenesis, and apoptosis. The cyclin D1 over-expression can cause apoptosis resistance in cell lines (Roue et al. Citation2008). In this study, we investigated the expressions of cyclin D1 after each treatment in MCF-7 cell line to know if our nanocomposite can reduce cyclin D1 expression in accordance with its anti-proliferative and cytotoxic activities. The outcomes revealed significant down regulation (P < 0.05) of cyclin D1 () after both DOX and DOX@MTX nanocomposite treatments. Since in other study (Kaabinejadian et al. Citation2008) and in our control group, strong expression of cyclin D1 was observed in MCF-7 cells, we concluded that down regulation of cyclin D1 in cells treated either with free combination of drugs or with nanocomposite of drugs is due to cytotoxic effects of them. However, the statistical analysis showed significant reduction of expression levels in DOX@MTX-loaded P(NIPAAm-MAA-DMAEMAQ) &MSNs&PECGC (ZD) as compared to DOX@MTX (D) which verified remarkably higher anti-tumoral activity of our nanocomposite. Also, our data showed that higher doses of ZD and D showed lower cytotoxicity and lower cyclin D1 expression in comparison with higher doses.
Conclusions
In summary, a novel multifunctional nanocarrier was successfully synthesized, fully characterized, and conjugated with two anticancer drugs, DOX and MTX. This developed dual anticancer drug delivery system showed good antibacterial activity, excellent stimuli-responsive co-drug delivery ability, and remarkable anti-tumoral activity. As a result, this drug delivery vehicle makes antibacterial and anti-cancer therapeutic processes proceed spontaneously, which could contribute to potentiate drug delivery in cancer therapy and representing more efficient drug delivery system in nanomedicine.
Acknowledgements
The authors gratefully acknowledge the Research Council [Grant No. 214/d/16348] of Azarbaijan Shahid Madani University for financial support.
Disclosure statement
The authors have no conflict of interest.
References
- Ahmed F, Pakunlu RI, Brannan A, Bates F, Minko T, Discher DE. 2006. Biodegradable polymersomes loaded with both paclitaxel and doxorubicin permeate and shrink tumors, inducing apoptosis in proportion to accumulated drug. J Control Release. 116:150–158.
- Alamri A, El-Newehy MH, Al-Deyab SS. 2012. Biocidal polymers: synthesis and antimicrobial properties of benzaldehyde derivatives immobilized onto amine-terminated polyacrylonitrile. Chem Central J. 6:13.
- Alao JP. 2007. The regulation of cyclin D1 degradation: roles in cancer development and the potential for therapeutic invention. Mol Cancer. 6:24. Epub 2007/04/05.
- Alidadiyani N, Salehi R, Ghaderi S, Samadi N, Davaran S. 2016. Synergistic antiproliferative effects of methotrexate-loaded smart silica nanocomposites in MDA-MB-231 breast cancer cells. Artif Cells Nanomed Biotechnol. 44:603–609.
- Argentiere S, Blasi L, Morello G, Gigli G. 2011. A novel pH-responsive nanogel for the controlled uptake and release of hydrophobic and cationic solutes. J Phys Chem C. 115:16347–16353.
- Askari S, Salehi R, Zarghami N, Akbarzadeh A, Rahmati-yamchi M. 2016. The anticancer effects of biodegradable nanomagnetic dual natural components on the leptin gene expression in lung cancer. Artif Cells Nanomed Biotechnol. 44:1753–1763.
- Bazmi Zeynabad F, Salehi R, Alizadeh E, Samadi Kafil H, Mohammad Hassanzadeh A, Mahkam M. 2015. pH-controlled multiple-drug delivery by a novel antibacterial nanocomposite for combination therapy. RSC Adv. 5:105678–105691.
- Carmona-Ribeiro AM, de Melo Carrasco LD. 2013. Cationic antimicrobial polymers and their assemblies. Int J Mol Sci. 14:9906–9946.
- Castro E, Mosquera V, Katime I. 2012. Dual drug release of triamterene and aminophylline from poly(N-isopropylacrylamide) hydrogels. Drug Deliv. 1:11.
- Chang B, Sha X, Guo J, Jiao Y, Wang C, Yang W. 2011. Thermo and pH dual responsive, polymer shell coated, magnetic mesoporous silica nanoparticles for controlled drug release. J Mater Chem. 21:9239–9247.
- Engler AC, Wiradharma N, Ong ZY, Coady DJ, Hedrick JL, Yang Y-Y. 2012. Emerging trends in macromolecular antimicrobials to fight multi-drug-resistant infections. Nano Today. 7:201–222.
- Eriksen EF, Kassem M. 1992. Fluoride stimulates proliferation and differentiation of human marrow stromal cells. Bone Miner. 17:196.
- Guo S, Qiao Y, Wang W, He H, Deng L, Xing J, et al. 2010. Poly(ɛ-caprolactone)-graft-poly(2-(N, N-dimethylamino) ethyl methacrylate) nanoparticles: pH dependent thermo-sensitive multifunctional carriers for gene and drug delivery. J Mater Chem. 20:6935–6941.
- He J, Söderling E, Österblad M, Vallittu PK, Lassila LV. 2011. Synthesis of methacrylate monomers with antibacterial effects against S. mutans. Molecules. 16:9755–9763.
- Hu CM, Aryal S, Zhang L. 2010. Nanoparticle-assisted combination therapies for effective cancer treatment. Ther Deliv. 1:323–334.
- Jin Q, Qu F, Jiang J, Dong Y, Guo W, Lin H. 2013. A pH-sensitive controlled dual-drug release from meso-macroporous silica/multilayer-polyelectrolytes coated SBA-15 composites. J Sol-Gel Sci Technol. 66:466–471.
- Kaabinejadian S, Fouladdel Sh, Ramezani M, Azizi E. 2008. Molecular analysis of Bcl-2 and cyclin D1 expression in differentially expressing estrogen receptor breast cancer MCF7, T47D and MDA-MB-468 cell lines treated with adriamycin. DARU J Pharm Sci. 16:182–188.
- Kang L, Gao Z, Huang W, Jin M, Wang Q. 2015. Nanocarrier-mediated codelivery of chemotherapeutic drugs and gene agents for cancer treatment. Acta Pharm Sin B. 5:169–175.
- Karnoosh-Yamchi J, Mobasseri M, Akbarzadeh A, Davaran S, Ostad-Rahimi AR, Hamishehkar H, Salehi R, et al. 2014. Preparation of pH sensitive insulinloaded nano hydrogels and evaluation of insulin releasing in different pH conditions. Mol Biol Rep. 41:6705–6712.
- Kenawy E-R, Abdel-Hay FI, El-Shanshoury AE-RR, El-Newehy MH. 1998. Biologically active polymers: synthesis and antimicrobial activity of modified glycidyl methacrylate polymers having a quaternary ammonium and phosphonium groups. J Control Release. 50:145–152.
- Laurent S, Bridot J-L, Elst LV, Muller RN. 2010. Magnetic iron oxide nanoparticles for biomedical applications. Future Med Chem. 2:427–449.
- Lee JH, Nan A. 2012. Combination drug delivery approaches in metastatic breast cancer. J Drug Deliv. 2012:1–17.
- Liu J, Huang Y, Kumar A, Tan A, Jin S, Mozhi A, Liang X-J. 2014. pH-Sensitive nano-systems for drug delivery in cancer therapy. Biotechnol Adv. 32:693–710.
- Liu Y, Cao X, Luo M, Le Z, Xu W. 2009. Self-assembled micellar nanoparticles of a novel star copolymer for thermo and pH dual-responsive drug release. J Colloid Interface Sci. 329:244–252.
- Mohammed M, Tahar B, Aïcha D, Eddine HD. 2010. Antibacterial activity of quaternary ammonium salt from diethylaminoethyl methacrylate. J Chem. 7:S61–SS6.
- Muñoz-Bonilla A, Fernández-García M. 2012. Polymeric materials with antimicrobial activity. Prog Polym Sci. 37:281–339.
- Ng VW, Chan JM, Sardon H, Ono RJ, García JM, Yang YY, Hedrick JL. 2014. Antimicrobial hydrogels: a new weapon in the arsenal against multidrug-resistant infections. Adv Drug Deliv Rev. 78:46–62.
- Ouahab A, Shao C, Shen Y, Tu J. 2014. Development and characterization of stabilized double loaded mPEG-PDLLA micelles for simultaneous delivery of paclitaxel and docetaxel. Drug Dev Ind Pharm. 40:860–868.
- Rasouli S, Davaran S, Rasouli F, Mahkam M, Salehi R. 2013. Positively charged functionalized silica nanoparticles as nontoxic carriers for triggered anticancer drug release. Des Monom Polym. 17:227–237.
- Rasouli S, Davaran S, Rasouli F, Mahkam M, Salehi R. 2014. Synthesis, characterization and pH-controllable methotrexate release from biocompatible polymer/silica nanocomposite for anticancer drug delivery. Drug Deliv. 21:155–163.
- Rizzotto M. 2012. Metal Complexes as Antimicrobial Agents. INTECH Open Access Publisher. Faculty of Biochemistry and Pharmacy National University of Rosario, Argentina.
- Roue G, Pichereau V, Lincet H, Colomer D, Sola B. 2008. Cyclin D1 mediates resistance to apoptosis through upregulation of molecular chaperones and consequent redistribution of cell death regulators. Oncogene. 27:4909–4920. Epub 2008/04/29.
- Salehi R, Davaran S, Rashid MR, Entezami AA. 2009. Thermosensitive nanoparticles prepared from poly(N-isopropylacrylamide-acrylamide-vinylpyrrolidone) and its blend with poly(lactide-co-glycolide) for efficient drug delivery system. J Appl Polym Sci. 111:1905–1910. Epub 1910.
- Salehi R, Hamishehkar H, Eskandani M, Mahkam M, Davaran S. 2014. Development of dual responsive nanocomposite for simultaneous delivery of anticancer drugs. J Drug Target. 22:327–342.
- Salehi R, Irani M, Eskandani M, Nowruzi K, Davaran S, Haririan I. 2014. Interaction, controlled release, and antitumor activity of doxorubicin hydrochloride from pH-sensitive P(NIPAAm-MAA-VP) nanofibrous scaffolds prepared by green electrospinning. Int J Polym Mater Polym. 63:609–619.
- Salehi R, Rasouli S, Hamishehkar H. 2015. Smart thermo/pH responsive magnetic nanogels for the simultaneous delivery of doxorubicin and methotrexate. Int J Pharm. 487:274–284.
- Schleich N, Sibret P, Danhier P, Ucakar B, Laurent S, Muller RN, et al. 2013. Dual anticancer drug/superparamagnetic iron oxide-loaded PLGA-based nanoparticles for cancer therapy and magnetic resonance imaging. Int J Pharm. 447:94–101.
- Schwartz VB, Thétiot F, Ritz S, Pütz S, Choritz L, Lappas A, et al. 2012. Antibacterial surface coatings from zinc oxide nanoparticles embedded in poly(N‐isopropylacrylamide) hydrogel surface layers. Adv Funct Mater. 22:2376–2386.
- Shen J-M, Gao F-Y, Yin T, Zhang H-X, Ma M, Yang Y-J, Yue F. 2013. cRGD-functionalized polymeric magnetic nanoparticles as a dual-drug delivery system for safe targeted cancer therapy. Pharmacol Res. 70:102–115.
- Singh A, Dilnawaz F, Mewar S, Sharma U, Jagannathan NR, Sahoo SK. 2011. Composite polymeric magnetic nanoparticles for co-delivery of hydrophobic and hydrophilic anticancer drugs and MRI imaging for cancer therapy. ACS Appl Mater Interfaces. 3:842–856.
- Varadaraji D, Suban SS, Ramasamy VR, Kubendiran K, Raguraman J, Nalilu SK, Pati HN. 2010. Synthesis and evaluation of a series of 1-substituted tetrazole derivatives as antimicrobial agents. Org Commun. 3:45–56.
- Vigliotta G, Mella M, Rega D, Izzo L. 2012. Modulating antimicrobial activity by synthesis: dendritic copolymers based on nonquaternized 2-(dimethylamino) ethyl methacrylate by Cu-mediated ATRP. Biomacromolecules. 13:833–841.
- Wang C-F, Mäkilä EM, Kaasalainen MH, Hagström MV, Salonen JJ, Hirvonen JT, Santos HA. 2015. Dual-drug delivery by porous silicon nanoparticles for improved cellular uptake, sustained release, and combination therapy. Acta Biomater. 16:206–214.
- Wang M, Zhou C, Chen J, Xiao Y, Du J. 2015. Multifunctional biocompatible and biodegradable folic acid conjugated poly(ɛ-caprolactone)–polypeptide copolymer vesicles with excellent antibacterial activities. Bioconjug Chem. 26:725–734.
- Wei L, Cai C, Lin J, Chen T. 2009. Dual-drug delivery system based on hydrogel/micelle composites. Biomaterials. 30:2606.
- Xiong W, Wang W, Wang Y, Zhao Y, Chen H, Xu H, Yang X. 2011. Dual temperature/pH-sensitive drug delivery of poly(N-isopropylacrylamide-co-acrylic acid) nanogels conjugated with doxorubicin for potential application in tumor hyperthermia therapy. Colloids Surf B: Bioinform. 84:447–453.
- Zhang L, Guo R, Yang M, Jiang X, Liu B. 2007. Thermo and pH dual‐responsive nanoparticles for anti‐cancer drug delivery. Adv Mater. 19:2988–2992.
- Zhang Y, Jiang J, Chen Y. 1999. Synthesis and antimicrobial activity of polymeric guanidine and biguanidine salts. Polymer. 40:6189–6198.
- Zhou C, Wang M, Zou K, Chen J, Zhu Y, Du J. 2013. Antibacterial polypeptide-grafted chitosan-based nanocapsules as an “armed” carrier of anticancer and antiepileptic Drugs. ACS Macro Lett. 2:1021–1025.
- Zhu W, Li Y, Liu L, Zhang W, Chen Y, Xi F. 2011. Biamphiphilic triblock copolymer micelles as a multifunctional platform for anticancer drug delivery. J Biomed Mater Res A. 96:330–340.
- Zuidema J. 1985. Ethylenediamine, profile of a sensitizing excipient. Pharm Weekbl Sci. 7:134–140.