Abstract
The aim of this study was to prove whether albumin-derived perfluorocarbon-based nanoparticles (capsules) can operate as a novel artificial oxygen carrier in a rat Langendorff-heart perfusion model. Hearts perfused with capsules showed increased left ventricular pressure and rate pressure product compared to hearts perfused with pure Krebs–Henseleit (KH)-buffer. The capsules prevented the myocardium from functional fail when in their absence a noxious ischemia was observed. Capsules did not change rheological properties of KH-buffer and could repeatedly reload with oxygen. This albumin-derived perfluorocarbon-based artificial oxygen carrier preserved the function of rat hearts due to the transport of oxygen in a satisfactory manner. Because of these positive results, the functionality of the applied capsules should be verified in living animals.
Introduction
In the last decades, the development of artificial oxygen carriers as red blood cell replacement gained in increasing importance (Chang Citation2003, Riess Citation2001, Weiskopf Citation2010). The option to replace blood by synthetic materials is necessary due to the fact that the willingness to donate blood is declining, while demand of blood transfusions is increasing. Advantages of synthetic materials compared to blood are for example the possibility to store the solutions for a long time without quality degradation, the decreased risk of infections and other side effects (Chang Citation2015). Furthermore, therapy of accident victims could be improved, as artificial oxygen carriers could be already available in the ambulance, hence the therapy could be started locally just in time (Chang Citation2015). Other possible applications of artificial oxygen carriers might be for example the therapy of hemorrhagic shock (Bian and Chang Citation2015, Chang Citation2015), cardiopulmonary bypass (Isaka et al. Citation2005), or transfusion to patients that refuse blood transfusion on religious grounds.
Among others, one promising strategy is the use of perfluorocarbons as an artificial oxygen carrier (Castro and Briceno Citation2010, Lowe Citation2003, Sloviter and Kamimoto Citation1967, Spiess, Citation2009). In 1966, Clark and Gollan (Clark and Gollan Citation1966) discover the property of perfluorocarbons to carry oxygen by preserving mammalian life due to liquid ventilation with perfluorocarbons. The physical dissolution of a large amount of the respiratory gases oxygen and carbon dioxide is the ingenious property of those molecules. Because of their biological inertness and the short organ retention times some perfluorocarbons, for example perfluorodecalin (PFD) or perfluorooctyl bromide, are auspicious for clinical use. However, for an intravenous use, perfluorocarbons have to be processed into blood compatible therapeutics in order to avoid their foaming in the lung (Flaim Citation1994). To solve this problem emulsification of perfluorocarbons displays one option, but the use of emulsifiers is associated with several side effects in vivo (Ingram et al. Citation1993, Mattrex et al. Citation1989, Vercellotti et al. Citation1982), finally resulting in biological incompatibility. Another promising option is the manufacture of an artificial oxygen carrier with a PFD core and an albumin shell.
Albumin is already discussed in the context of artificial oxygen carriers (Sloviter and Kamimoto Citation1967, Tsuchida et al. Citation2009). Because of its biocompatibility and amphiphilic character any synthetic emulsifiers can be safely neglected (Clark et al. Citation1970). Although other studies discovered that protein membranes are nearly gas tight (Phupoksakul et al. Citation2015, Sothornvit and Krochta Citation2000), our previous in vitro characterizations showed the capability of albumin-derived nanocapsules for the transport of oxygen (Laudien et al. Citation2017). The main aim of this study is to prove the functionality of albumin-derived perfluorocarbon-based artificial oxygen carriers in a physiological model. Furthermore, the competence of repeated loading with oxygen was to be demonstrated.
For the proof of the concept, we applied the capsules to an isolated retrograde perfused heart, that is the Langendorff-heart perfusion model.
Materials and methods
Materials
PFD was obtained from Fluorochem Chemicals (Derbyshire, UK). Bovine serum albumin (BSA), NaCl, KCl, and NaH2PO4·H2O were obtained from Carl Roth (Karlsruhe, Germany). MgCl2·6H2O, CaCl2·2H2O, NaHCO3 were purchased from Merck (Darmstadt, Germany) and glucose was purchased from Sigma Aldrich (Steinheim, Germany).
Methods
Synthesis of capsules
The synthesis of capsules was based on a method of Sloviter and Kamimoto (Citation1967), modified as previously described (Laudien et al. Citation2017). Briefly, 5 ml buffer and 1 ml PFD were combined in a reaction tube and sonicated for 90 s using a sonotrode. After synthesis, capsules were adjusted to 2, 4, or 6 vol% as described previously (Laudien et al. Citation2017).
Size distribution and capsule concentration were determined by dynamic light scattering with a NANO-flex® (Particle Metrix, Meerbusch, Germany). Analysis was performed at 25 °C with a detection angle of 180°.
Viscosity
The measurements of the dynamic viscosities were carried out by a rotational viscometer “Low Shear 40” from proRheo and a DIN 412 Coutte measurement system. To check differences between buffer and buffer containing 4 vol% capsules sixfold measurements were done for all samples (each of the six samples was measured onefold) at shear rates from 1 to 120 rad/s and 37 °C. Before each measurement, the sample was equilibrated for 1 min to obtain the desired temperature.
Oxygen capacity of capsules
To determine the oxygen capacities of the capsules, oxygen content of capsule dispersions was discovered using a respirometer (Oxygraph-2k, Oroboros Instruments, Innsbruck, Austria) as described previously (Laudien et al. Citation2017). Briefly, measuring chambers were filled with phosphate buffer (50 mM) and hermetically sealed. Oxygen resolved in buffer was removed by adding 96 μl yeast (83 mg/ml in phosphate buffer) and 50 μl glucose (1 M in phosphate buffer). Subsequently, 60 μl potassium cyanide (100 mM in phosphate buffer) was added to prevent aerobic metabolism of the yeast in the processing experiment.
To ensure oxygen charging of the capsule dispersion or buffer, a reaction vessel was sealed using a septum and flushed with carbogen gas for a minute to create the same atmosphere as in the Langendorff-apparatus. Subsequently, 2 ml of KH-buffer or KH-buffer containing 4 vol% capsules was injected into the reaction vessel and incubated at 37 °C in carbogen gas atmosphere while stirring. After oxygen loading, 50 μl of KH-buffer or KH-buffer containing 4 vol% capsules was applied to the oxygen-free measuring chambers of the Oxygraph 2-k under exclusion of air. Oxygen capacity was given in ml/dl.
Gas saturation
The NMR experiments were run on a Bruker DRX (Karlsruhe, Germany) 500 spectrometer at a fluorine resonance frequency of 500 Hz. Simple single-pulse excitation was used to obtain fluorine line spectra. An external standard was used for a reliable determination of the chemical shifts. Spin-lattice relaxation times were determined in a conventional inversion recovery experiment. For measurements under variation of the atmosphere, a home-built sample holder was used, which carries individual gas inlet capillaries for oxygen and nitrogen in order to enable an instantaneous switching between different atmospheres. The gas flow for oxygen and nitrogen was adjusted such that the overall flow rate amounted to 0.36–0.4 ml/min. The gas flow rate is a compromise between sufficient mixing of the liquid phase (in order to minimize gas concentration gradients in the liquid) and minimal sample inhomogeneity due to gas bubbles which causes broadening of the NMR signals. The saturation experiments with nitrogen and oxygen were carried out with a solution of 50% capsule suspension in D2O. The dilution is necessary in order to reduce foaming during the continuous gas flow. The exchange between oxygen and nitrogen atmosphere and vice versa was induced by simultaneous opening and closing of the respective valves. In the time resolved NMR measurement during the gas treatment, a single pulse experiment is repeated every 20 s. Each resulting free induction decay is Fourier transformed to yield a 19F NMR spectrum for the determination of the time-dependent chemical shift. The state of saturation was determined from the paramagnetic shift of the 19F trans-bridging fluorine signal at −194.5 ppm. At the end of each saturation measurement, the 19F-T1 relaxation time of the trans-bridging fluorine atoms was measured to characterize the maximum gas exchange. After the nitrogen saturation, a T1 value of 2.54 s was detected. This corresponds to an oxygen partial pressure of 45.8 h Pa. After the oxygen saturation, T1 decreased to 0.61 s. This corresponds to an oxygen partial pressure of 647.5 h Pa. The partial pressures were determined by means of a calibration curve of the oxygen saturation of pure PFD. The degree of oxygen saturation in the fluorinated hydrocarbon is then calculated from the chemical shift using data from independent calibration measurements.
Animals
A total number of 26 female Wistar rats (Rattus norvegicus, 225–275 g, 4 months old) were obtained from the central animal unit of the Justus-Liebig University. All animals received human care according to the standards of Annex III of the directive 2010/63/EU of the European Parliament and of the Council of 22 September 2010 on the protection of animals used for scientific purposes (EPaE Council Citation2010). The experimental protocol was announced to the local authorities, Germany.
Heart perfusion
Experiments were performed on isolated hearts from female Wistar rats as described before (Schreckenberg et al. Citation2015). Rats were anesthetized in an isoflurane chamber; 4–5% isoflurane. After deep anesthesia was achieved, the hearts were rapidly excised and the aorta was cannulated for retrograde perfusion with a 16-gauge needle connected to a Langendorff-perfusion system. A modified KH buffer (NaCl 105 mM; KCl 2.7 mM; NaH2PO4·H2O 0.4 mM; MgCl2·6H2O 2.0 mM; glucose 5.0 mM; CaCl2·2H2O 3.6 mM; NaHCO3 30 mM; BSA 0.75 mM) was used. The buffer was saturated with 95% O2 and 5% CO2. A polyvinyl chloride balloon was inserted into the left ventricle through the mitral valve and held in place by a suture tied around the left atrium. The intraventricular balloon was inflated to give a diastolic pressure of 10 mmHg and balloon volume was held constant thereafter. The other end of the tubing was connected to a pressure transducer for continuous measurement of left ventricular pressure. Left ventricular developed pressure (LVDP) was calculated as the difference between peak systolic pressure and diastolic pressure. Data are also given as rate pressure product (RPP = LVDP × heart rate). A second transducer connected to the perfusion line just before the heart was used to measure perfusion pressure. The flow was initially adjusted to a perfusion pressure of 50 mmHg (approximately 10 ml/min) and reduced to 50% to induce a low-flow ischemia.
Dose determination: To find the ideal capsule concentration different concentrations of capsules (2, 4, and 6 vol%) were tested. The control-heart was perfused with pure buffer. In the initial period of stabilization, both hearts were perfused with a perfusion flow of 100% and pure buffer for 10 min (, basal, 100% flow). Subsequently, the flow was reduced to 50% to initiate a moderate low-flow ischemia (, basal, 50% flow). After 10 min of stabilization the first treatment (pure buffer or buffer containing 2 vol% capsules) was applied for 10 min. After a wash-out period of 10 min with pure buffer, hearts were treated for 10 min with pure buffer or buffer containing 4 vol% capsules, respectively. A second wash-out period was followed by the last treatment (buffer or buffer containing 6 vol% capsules, respectively). At the end of the experiment, a final wash-out was performed. LVDP was measured to assess the heart vitality. This test was done with n = 1 control-heart and n = 1 capsule-heart.
Repetition: In this experiment, hearts were repeatedly perfused with 4 vol% capsules. After a stabilization period of 10 min with 100% flow and pure buffer, flow was reduced to 50% for 10 min. Thereafter, the first treatment with either pure buffer or buffer containing 4 vol% capsules was realized for 10 min. After a subsequent wash-out period with pure buffer for 10 min, the treatment was repeated. LVDP and RPP were measured to assess the heart vitality. This experiment was done with n = 6 control-hearts and n = 6 capsule-hearts.
Flow reduction: During this investigation, a stepwise flow reduction from 100% to 20% was performed before the initial flow of 100% was readjusted. At first hearts were stabilized for 10 min at 100% flow with buffer or buffer containing 4 vol% capsules, respectively, as perfusion medium. Every 10-min flow was reduced stepwise by 20% to 80%, 60%, 40%, and 20% of initial flow. At the end of the experiment, the initial flow of 100% was re-established for 10 min. LVDP and RPP were measured to assess the heart vitality. This investigation was done with n = 6 control-hearts and n = 6 capsule-hearts.
Statistical analyses
Data are expressed as mean values ± SD (if n > 1). O2 capacity test statistics were performed by unpaired t test; a P value <0.05 was considered to indicate significance. Heart perfusion comparisons among multiple groups were performed by multiple t test followed by Holm–Sidak post hoc analysis; a P value <0.05 was considered to indicate significance. All analyses were performed using the Graph Pad Prism (6.05) software package (Graph Pad Software Inc., La Jolla, CA).
Results
Physico-chemical characterization of the capsules
Before the capsules were used for perfusion, their physico-chemical properties were characterized. Capsules displayed an average hydrodynamic diameter of 292 ± 29 nm and the 4 vol% capsule dispersion contained about 1.03 × 1010 particles per milliliter. The corresponding dynamic viscosity of the buffer in the presence of 4 vol% capsules showed a slight increase as compared to their absence irrespective of the shear rate ().
Figure 1. Dynamic viscosity of buffer and buffer containing 4 vol% capsules. The viscosity of buffer containing 4 vol% capsules (spheres) as well as the viscosity of pure buffer (squares) was measured and compared to each other. Error bars represent standard deviations with n = 6.
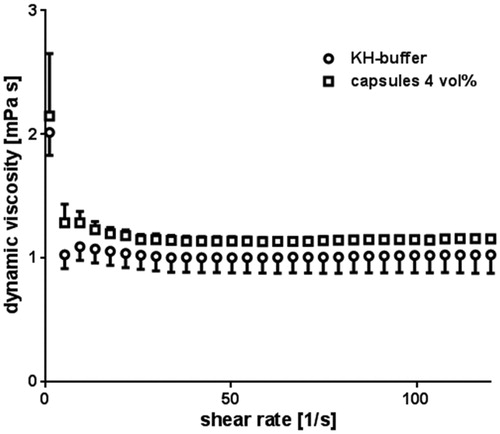
In vitro measurements of the oxygen capacities () revealed that 4 vol% capsules dispersed in KH-buffer accumulated 1.6-fold more O2 (4.79 ml O2/dl ±1.4 ml O2/dl) compared to capsule-free KH-buffer (3.05 ml/dl ±0.6 ml O2/dl).
Figure 2. Oxygen transport capacities of pure KH-buffer (dark grey) and KH-buffer containing 4 vol% capsules (light grey) were measured at pO2 = 713 mmHg and compared to each other with *P < 0.05. Error bars represent standard deviations with n = 25.
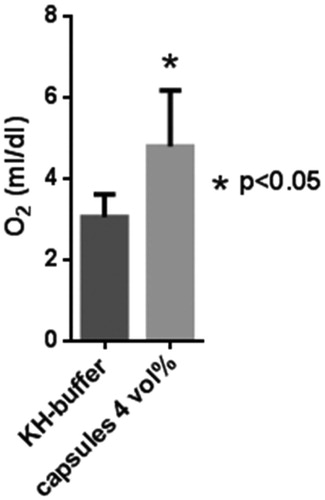
The NMR measurements monitored the degree of oxygen saturation after the dispersion was repeatedly purged with either nitrogen (N2, black dots) or oxygen (O2, dark grey squares, ). The light grey triangles in represent the 19F shift reflecting O2 saturation which, by means of a calibration plot, lead to values of relative oxygen saturation. The black dots showed the reduction of the oxygen saturation during the treatment with nitrogen, the dark grey squares in turn reflected the increasing oxygen saturation under oxygen. Overall, the plot revealed the periodicity of the oxygen loading and unloading of the capsules in the dispersion. Loading as well as unloading was completely reversible, which is documented by the constant values reached after each single purging step.
Figure 3. Gas exchange in a nanocapsule dispersion. Three cycles of oxygen-loading and release are shown over a time period of 300 min (x-axis). The data were observed under purging with O2 (dark grey squares) and with N2 (black dots) of a buffer solution containing 4 vol% capsules. The 19F chemical shift (right y-axis) was measured and related to the degree of O2 saturation (left y-axis) via a calibration curve.
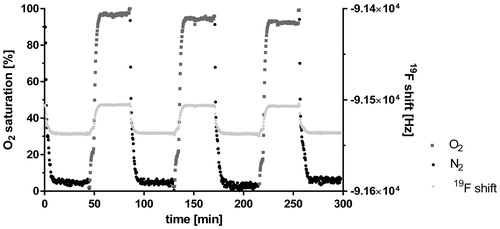
These data suggest a successful application of modified KH-buffer in the presence and absence of capsules in the Langendorff-heart perfusion model.
Heart perfusion
Dose determination
In this experiment, the necessary concentration of capsules for an optimal function of the rat heart under a reduced flow was evaluated. The LVDP reached with 100% flow and pure buffer during the basal stabilization phase was set to 100% (, dark grey background). In the following step (still both hearts were perfused with buffer), the flow was reduced to 50% and remained from now on at 50% till the end of the experiment (, light grey background). This flow reduction decreased the LVDP to 62.3% (control-heart) and 58.6% (capsule-heart). During the first treatment (2 vol% capsules or pure buffer, respectively), LVDP of the control-heart gained 71.0%, while in the capsule-heart LVDP reached 73.5%. During the first wash-out period, LVDP of control-hearts decreased to 49.0% and to 65.0% in the capsule-hearts. The second treatment period (4 vol% capsules) resulted in a LVDP of 49.9% (control-heart) and 110.4% (capsule-heart). In spite of the reduced flow (50%), the treatment with 4 vol% capsules originated a LVDP higher than the initial LVDP at 100% flow with pure buffer. After the next wash-out, LVDP decreased to 74.1% and 85.9% in the control- and capsule-hearts, respectively. During the treatment with 6 vol% capsules, LVDP of the control-heart fell down to 51.4%, whereas capsule-heart reached 123.3%. In the final wash-out step, LVDP in the control-heart was 50.1%, while the capsule-heart reached 61.9%. Because of these finding, a dose of 4 vol% capsules in buffer was applied in all following experiments.
Figure 4. Determination of the ideal dose. After the initial stabilization period (flow 100%), the flow was reduced (flow 50%). For dose determination, control-heart (dark grey) was perfused with pure buffer compared to capsule-heart (light grey), which was perfused with buffer containing 2 vol% (first treatment), 4 vol% (second treatment), and 6 vol% (third treatment) capsules. Wash-out steps were performed with pure buffer. The initial LVDP was set to 100%. n = 1.
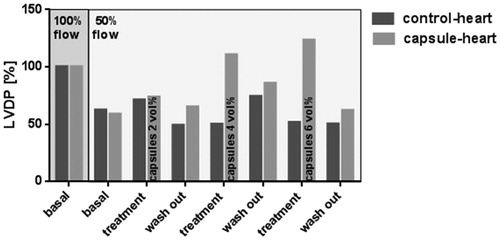
Repetition
After dose determination, the effect of recurring vitality was repeated twofold. The flow of 100% in the initial stabilization period (, dark grey background) led to a LVDP of 91.9 mmHg and a RPP of 13.0 mmHg*bpm*1000 in the control-hearts and revealed a LVDP of 86.3 mmHg and a RPP of 14.0 mmHg*bpm*1000 in the capsule-hearts. After flow reduction to 50% (, light grey background), control-hearts showed a LVDP of 57.0 mmHg and a RPP of 6.1 mmHg*bpm*1000 similar to the capsule-hearts, which reached a LVDP of 57.0 mmHg and a RPP of 5.6 mmHg*bpm*1000. The first treatment period resulted in a LVDP of 57.3 mmHg and a RPP of 6.4 mmHg*bpm*1000 in the control-hearts. The capsule-hearts gained a LVDP of 68.0 mmHg and a RPP of 8.5 mmHg*bpm*1000. The following wash-out period led to a LVDP of 63.2 mmHg and a RPP of 6.9 mmHg*bpm*1000 in the control-hearts, while the capsule-hearts reached a LVDP of 60.3 mmHg and a RPP of 6.3 mmHg*bpm*1000. The second treatment resulted in a LVDP of 63.8 mmHg and a RPP of 7.0 mmHg*bpm*1000 in the control-hearts and in a LVDP of 70.0 mmHg and a RPP of 10.3 mmHg*bpm*1000 in the capsule-hearts. To guarantee reliability, the experimental setting was successfully repeated twofold.
Figure 5. Effect of a repeated treatment on LVDP and RPP. After the initial stabilization period (flow 100%), the flow was reduced (flow 50%). Control-hearts (dark grey) were perfused with pure buffer and capsule-hearts (light grey) with buffer containing 4 vol% capsules. Wash-out periods were performed with pure buffer. LVDP and RPP were measured. Error bars represent standard deviations with n = 6.With P < 0.05 no significant differences were detected if compared to correspondent control-heart.
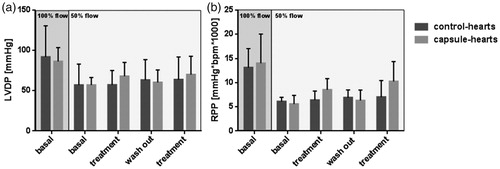
Flow reduction
In order to investigate the beneficial impact of the capsules a massive ischemia was induced by stepwise flow reduction. In the initial period (, 100% flow), the control-hearts developed a LVDP of 106.4 mmHg and a RPP of 23.1 mmHg*bpm*1000 compared to the capsule-hearts reaching a LVDP of 109.2 mmHg and a RPP of 22.0 mmHg*bpm*1000. The first flow reduction step (80% flow) led to a LVDP of 79.5 mmHg and a RPP of 16.0 mmHg*bpm*1000 in the control-hearts. The capsule-hearts gained a significant higher LVDP of 100.5 mmHg and a RPP of 18.0 mmHg*bpm*1000. The second flow reduction (60% flow) decreased LVDP and RPP to 72.8 mmHg and 12.2 mmHg*bpm*1000, respectively (control-hearts). The capsule-hearts reached a LVDP of 92.3 mmHg and a RPP of 14.4 mmHg*bpm*1000. The third flow reduction (40% flow) resulted in a LVDP of 48.2 mmHg and a RPP of 7.7 mmHg*bpm*1000 in the control-hearts. In contrast to the control-hearts, the capsule-hearts achieved a LVDP of 70.6 mmHg and a significant higher RPP of 11.2 mmHg*bpm*1000. During the fourth flow reduction (20% flow), LVDP of the control-hearts was 32.8 mmHg compared to the capsule-hearts, which reached a significant higher LVDP of 48.1 mmHg. In this period, RPP dropped down to 3.0 mmHg*bpm*1000 (control-hearts) and 4.0 mmHg*bpm*1000 (capsule-hearts). The final flow increase (100% flow) caused a LVDP of 104.4 mmHg in the control-hearts and a significant higher LVDP of 133.8 mmHg in the capsule-hearts. Compared to the initial phase of the experiment with a flow of 100% the final LVDP of the capsule-hearts (133.8 mmHg) exceeded the initial LVDP by 24.6 mmHg, whereas the control-hearts (104.4 mmHg) failed to reach their initial LVDP by 2 mmHg. At the same period, RPP of both groups returned to the initial level (control-hearts 23.6 mmHg*bpm*1000 and capsule-hearts 23.3 mmHg*bpm*1000). Thus, strongly ischemic hearts benefited impressively from the perfusion with capsules.
Figure 6. Consequence of a massive flow reduction on LVDP and RPP. After the initial stabilization period (flow 100%), the flow was reduced stepwise by 20% to 80%, 60%, 40% and 20%, respectively, of initial flow. At the end of the experiment, the initial flow of 100% was re-established. Control-hearts (dark grey) were perfused with pure buffer and capsule-hearts (light grey) with buffer containing 4 vol% capsules. LVDP and RPP were measured. Error bars represent standard deviations with n = 6, *P < 0.05 as compared to correspondent control-heart.
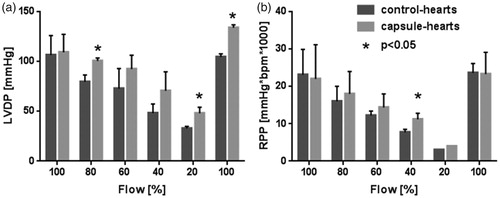
Discussion
After promising short-term in vivo toxicity studies with in vivo application of small amounts of albumin-derived perfluorocarbon-based nanocapsules (Laudien et al. Citation2017), for the first time, this study showed the in vitro functionality of albumin-derived perfluorocarbon-based nanocapsules, because the nanocapsules can preserve the performance of rat hearts due to the effective transport of oxygen.
Physico-chemical requirements of the capsules for a successful perfusion
The use of KH buffer for Langendorff-heart perfusion is well-established (Bell et al. Citation2011, Dhein et al. Citation2005, Krebs and Henseleit Citation1932, Sutherland and Hearse Citation2000). In order to exclude negative effects of the modified KH buffer, which was used in this study, a physico-chemical analysis in the absence and presence of capsules was done.
Concerning the rheological properties, the slight increase of the dynamic viscosity for the applied buffer containing 4 vol% capsules with regard to the capsule free buffer () could be explained by the fact that particles in solutions cause an increase of dynamic viscosity (Nguyen et al. Citation2008). In line with this conclusion, other studies showed similar effects of their HSA solutions with and without particles (Haruki et al. Citation2015). In addition, pure buffer acts as a Newtonian fluid, which means that its viscosity is independent from the present shear rate. depicts that the capsules did not influence this property; the buffer containing capsules still showed Newtonian flow. Therefore, usual flow rates could be applied, resulting in an adequate oxygenation of the heart at 100% flow, due to the amount of physically dissolved O2 in the pure buffer.
A potential embolization of small capillaries due to large capsules, as observed in other studies (Ashruf et al. Citation1999) seemed unlikely, because the DLS measurement of the hydrodynamic diameter of the applied capsules showed a mean size of only 292 nm. This was far below the critical diameter (1 μm) accounted for fast capsule degradation by the reticuloendothelial system in vivo (Keipert et al. Citation1994, Riess Citation2001, Schäfer et al. Citation1994, Sloviter and Kamimoto Citation1967). Other Langendorff-heart perfusion studies successfully used PFD-emulsions with similar particle sizes (Isaka et al. Citation2008, Segel et al. Citation1994). Consequentially, a sufficient increase of the intravascular half-life of the capsules can be predicted for in vivo experiments in the future. This indeed has been already foreshadowed by in vivo experiments exploring the biocompatibility with the same capsules (Laudien et al. Citation2017). Prolonged intravasal half-life of the capsules is probably not solely based on the small particle size but rather on the use of albumin as wall material, because albumin is commonly used by the pharmaceutical industry to increase half-life of particles in vivo (Haruki et al. Citation2015, Roser et al. Citation1998).
A prerequisite for a functional artificial oxygen carrier is its ability to take-up oxygen in the lung and to release it in the periphery tissue (Chang Citation2015). The measured oxygen capacity in the presence of the capsules () was significantly higher than in its absence. Thus perfluorocarbon-based artificial oxygen carriers did release oxygen once they had been loaded and therefore are suitable to supply the Langendorff-heart with oxygen during perfusion. This was far from self-evident, as in general proteinaceous membranes are nearly gas tight (Phupoksakul et al. Citation2015, Sothornvit and Krochta Citation2000). The repeated gas saturation () simulated physiological “breathing.” Importantly, it was shown that the capsules could be loaded and unloaded with different gases manifold. This property is not an obligatory prerequisite in the single-pass Langendorff-perfusion, but would be an important quality for recirculating organ perfusion and in vivo experiments. O2/CO2 loading and reloading in the lung is a mandatory function for a preparation designed to replace red blood cells (Chang Citation2015). Furthermore, this experiment confirmed again that the capsule membrane is not a dense accumulation of albumin molecules but more or less a flexible cobweb granting gases the opportunity to cross this barrier.
In summary, albumin-derived perfluorocarbon-based artificial oxygen carriers meet all the main requirements for a successful perfusion of the Langendorff-heart.
Dose determination – how much oxygen does the Langendorff-heart need?
Initially, it was important to determine the optimal dose of oxygen for the heart perfusion. Therefore, in a setting similar to other low flow ischemia studies (Assayang et al. Citation1998, Brunet et al. Citation1995, McCormack et al. Citation1996), different vol% of capsules were tested during a moderate low flow ischemia (). As common for the Langendorff-model (Sutherland and Hearse Citation2000), a flow of approximately 10 ml/min was initially adjusted to generate a normal working Langendorff-heart in the perfusion apparatus (100% flow, ). To assess the effect of the capsules, the LVDP resulting from this initial flow (flow 100%) was set to 100%. The flow reduction to 50% decreased LVDP as a result of the moderate ischemia (). The ischemia of the myocardium developed because the physically dissolved O2 in the buffer was too low for an appropriate oxygen supply in the absence of (artificial) oxygen carriers.
A capsule concentration of 2 vol% failed in restoring LVDP to initial levels at 100% flow. However, the situation after treatment with 4 vol% capsules was quite satisfactorily. In spite of the reduced flow (50% flow) the capsule-heart showed good vitality by slightly exceeding the initial LVDP due to sufficient O2 supply and removal of the accumulated respiratory gas CO2. As the increase of capsules did not further improve heart viability but resulted in an abnormal rise of LVDP, all following experiments were performed with 4 vol% capsules.
Repetition – can the langendorff-heart be repeatedly oxygenated?
After establishing the optimal dose of the capsules for successful oxygenation of the heart, the proof of reproducibility of this effect depicted consequentially the next step. indicates that with 100% flow the hearts reached RPP levels similar to those found in other designed flow studies (Pasdois et al. Citation2013). Consequently, a flow decrease by 50% resulted in a moderate ischemia that was highly visible in the decreased LVDP and RPP, respectively (). This decrease of vital parameters illustrated again the insufficient O2 supply by the capsule-free buffer ( and ). While control-hearts never recovered, hearts perfused with capsules showed a distinct trend to recuperation (). Especially, the RPP of the capsule-hearts during the treatment was clearly improved (). This positive trend was even more marked after the second treatment.
Thus, this experiment clearly demonstrated that the buffer containing 4 vol% capsules provided enough O2 to protect a heart repeatedly against moderate ischemia.
Flow reduction – do the artificial oxygen carriers preserve oxygenation of the Langendorff-heart when blood-flow is disturbed?
In this experiment, the flow was reduced stepwise resulting in a severe ischemia of the hearts at 20% flow (Assayang et al. Citation1998). This procedure was done to check what flow rate reduction the capsules could compensate for. In contrast to the other experiments ( and ) in this data set, the capsule-hearts were perfused with buffer containing 4 vol% capsules over the whole experimental period (), which extended the treatment period from 10 to 60 min. Although starting with similar LVDP and RPP initially, the first flow decrease to 80% flow was tolerated better in the presence of capsules as indicated by the significant higher LVDP and an improved RPP, if compared to capsule-free conditions. This situation persisted during the next two reduction steps (flow 60%, flow 40%), the hearts perfused with capsules showed again more vitality because of higher LVDP and RPP as compared to capsule free conditions. Importantly, even at a flow of 20%, meaning that the medium perfused the heart with only about 2 ml/min, the hearts showed much better functionality in the presence of capsules (). Surprisingly, a period of 40 min ischemia apparently had not caused permanent damage irrespectively of the presence of capsules, maybe due to the fact that free albumin (5%) was present as adjuvant in the buffer. It is already known that the presence of albumin improves stability and longevity of perfluorochemical-perfused hearts (Segel and Ensunsa Citation1988). In addition, hearts perfused with an albumin-enriched buffer benefit from its known oncotic capabilities (Sutherland and Hearse Citation2000). After the restoration of 100% flow, hearts under control conditions reached initial LVDP and RPP while in the presence of capsules hearts even exceeded the initial LVDP, thereby demonstrating an excellent power of the hearts. Other studies with KH-buffer in the Langendorff-heart perfusion model revealed less injured cells in hearts perfused with perfluorocarbons emulsions, based on significant lower lactate levels (Rahamathulla et al. Citation1985). Equally, this may be the case for our albumin-derived perfluorocarbon-based capsules and thereby could explain the better outcome of the hearts in the presence of capsules.
More clinically focused studies examined the use of perfluorocarbon emulsions as a therapeutic agent in cardiopulmonary bypass (Gale et al. Citation2007, Isaka et al. Citation2005, McDonagh et al. Citation2001, Rosoff et al. Citation1998). Because of the positive data presented here the albumin-derived perfluorocarbon nanocapsules seem to be a further promising aspirant for this indication. Even reduced ischemia-reperfusion injury is discussed as an advantage by the use of perfluorochemical emulsions as compared to the use of either normal saline in a model of abdominal surgery in rats (Fujino et al. Citation2003) or whole blood in an isolated porcine heart model (Martin et al. Citation1992). It is conceivable that the application of albumin-derived perfluorocarbon-based nanoparticles reduced ischemia-reperfusion injury too, because of the ability of the capsules to deliver a high amount of O2 to the tissue while removing simultaneously CO2 from the tissue.
All in all we can assume that even in the case of massive ischemia the buffer containing only 4 vol% capsules was able to deliver enough oxygen to prevent the myocardium from functional fail. Furthermore, a treatment with capsules over a time period of 60 min resulted in a completely functional Langendorff-heart without any signs of damage that potentially could have been caused by a treatment with nanoparticles. This is why many therapeutic areas open up for the use of albumin-derived perfluorocarbon nanoparticles.
Abbreviations | ||
LVDP | = | left ventricular developed pressure |
RPP | = | rate pressure product |
PFD | = | perfluorodecalin |
BSA | = | bovine serum albumin |
PFC | = | perfluorocarbon |
Acknowledgements
The technical assistance of Peter Volk is gratefully acknowledged.
Disclosure statement
The authors report no conflicts of interest. The authors alone are responsible for the content and writing of the paper.
References
- Ashruf JF, Ince C, Bruining HA. 1999. Regional ischemia in hypertrophic Langendorff-perfused rat hearts. Am J Physiol Heart Circ. 277:H1532–H1539.
- Assayang P, Charlemagne D, Marty I, Leiris J, Lompre AM, Boucher F, et al. 1998. Effects of sustained low-flow ischemia on myocardial function and calcium-regulating proteins in adult and senescent rat hearts. Cardiovasc Res. 38:169–180.
- Bell RM, Mocanu MM, Yellon DM. 2011. Retrograde heart perfusion: the Langendorff technique of isolated heart perfusion. J Mol Cell Cardiol. 50:940–950.
- Bian Y, Chang TMS. 2015. A novel nanobiotherapeutic poly-[hemoglobin-superoxide dismutase-catalase-carbonic anhydrase] with no cardiac toxicity for the resuscitation of a rat model with 90 minutes of sustained severe hemorrhagic shock with loss of 2/3 blood volume. Artif Cells Nanomed Biotechnol. 43:1–9.
- Brunet J, Boily MJ, Cordeau S, Des Rosiers C. 1995. Effects of N-acetylcysteine in the rat heart perfused after low-flow ischemia: evidence for a direct scavenging of hydroxyl radicals and nitric oxide-dependent increase in coronary flow. Free Radic Biol Med. 19:627–638.
- Castro CI, Briceno JC. 2010. Perfluorocarbon-based oxygen carriers: review of products and trials. Artif Organs. 34:622–634.
- Chang TMS. 2003. Future generations of red blood cell substitutes. J Intern Med. 253:527–535.
- Chang TMS. 2015. Red blood cell replacement, or nanobiotherapeutics with enhanced red blood cell functions? Artif Cells Nanomed Biotechnol. 43:145–147.
- Clark LC Jr, Kaplan S, Becattini F, Benzing G. 1970. Perfusion of whole animals with perfluorinated liquid emulsions using the Clark bubble-defoam heart-lung machine. Fed Proc. 29:1764–1770.
- Clark LC, Gollan F. 1966. Survival of mammals breathing organic liquids equilibrated with oxygen at atmospheric pressure. Science. 152:1755–1756.
- Dhein S, Mohr FW, Delmar M. 2005. The Langendorff Heart. Practical Methods in Cardiovascular Research. Berlin Heidelberg: Springer-Verlag, pp. 155–172.
- EPaE Council. 2010. European Commission (2010) Directive 2010/63/EU on Protection of Animals Used for Scientific Purposes. Ispra, Italy: Institute for Health and Consumer Protection.
- Flaim SF. 1994. Pharmacokinetics and side effects of perfluorocarbon-based blood substitutes. Artif Cells Blood Substit Immobil Biotechnol. 22:1043–1054.
- Fujino Y, Suzuki Y, Kakinoki K, Tanioka Y, Ku Y, Kuroda Y. 2003. Protection against experimental small intestinal ischaemia-reperfusion injury with oxygenated perfluorochemical. Br J Surg. 90:1015–1020.
- Gale SC, Gorman GD, Jack G, Jack BS, Copeland MD, McDonagh PF. 2007. Perflubron emulsion prevents PMN activation and improves myocardial functional recovery after cold ischemia and reperfusion. J Surg Res. 138:135–140.
- Haruki R, Kimura T, Iwasaki H, Yamada K, Kamiyama I, Kohno M. 2015. Safety evaluation of hemoglobin-albumin cluster ‘hemoact’ as a red blood cell substitute. Sci Rep. 5:12778.
- Ingram DA, Forman MB, Murray JJ. 1993. Activation of complement by fluosol attributable to the pluronic detergent micelle structure. J Cardiovasc Pharmacol. 22:456–461.
- Isaka M, Sakuma I, Imamura M, Makino Y, Fukushima S, Nakai K, et al. 2005. Experimental studies on artificial blood usage for hemodilution during cardiopulmonary bypass. Ann Thorac Cardiovasc Surg. 11:238–244.
- Isaka M, Sakuma I, Shiiya N, Fukushima S, Nakai K, Kitabatake A, et al. 2008. Experimental study of the relationship between perfluoro-octyl bromide emulsion and norepinephrine release in reperfusion arrhythmia: isolated guinea pig heart model. Ann Thorac Cardiovasc Surg. 14:363–368.
- Keipert P, Otto S, Flaim SF, Weers JG, Schutt EA, Pelura TJ, et al. 1994. Influence of perflubron emulsion particle size on blood half-life and febrile response in rats. Artif Cells Blood Substit Immobil Biotechnol. 22:1169–1174.
- Krebs HA, Henseleit K. 1932. Untersuchungen ueber die Harnstoffbildung im Tierkoerper. Hoppe-Seyler’s Z Physiol Chem. 210:33–36.
- Laudien J, Wrobeln A, Groß Heitfeld C, Linders J, Mayer C, Wilde B, et al. 2017. Albumin-derived perfluorocarbon-based artificial oxygen carriers: A physico-chemical characterization and first in vivo evaluation of biocompatibility. Eur J Pharm Biopharm, under revision.
- Lowe KC. 2003. Engineering blood: synthetic substitutes from fluorinated compounds. J Tissue Eng. 9:389–399.
- Martin SM, Laks H, Drinkwater DC, Stein DG, Barthel SW, Capouya ER, et al. 1992. Perfluorochemical reperfusion limits myocardial reperfusion injury after prolonged hypothermic global ischemia. Biomater Artif Cells Immobilization Biotechnol. 20:985–989.
- Mattrey RF, Hilpert PL, Long CD, Long DM, Mitten RM, Peterson T. 1989. Hemodynamic effects of intravenous lecithin-based perfluorocarbon emulsions in dogs. Crit Care Med. 17:652–656.
- McCormack JG, Barr RL, Wolff AA, Lopaschuk GD. 1996. Ranolazine stimulates glucose oxidation in normoxic, ischemic, and reperfused ischemic rat hearts. Circulation. 93:135–142.
- McDonagh P, Cerney K, Hokama JH, Lai G, Gonzales R, Davis-Gorman G, et al. 2001. Perflubron emulsion reduces inflammation during extracorporeal circulation. J Surg Res. 99:7–16.
- Nguyen CT, Desgranges F, Galanis N, Roy G, Mare T, Boucher S, et al. 2008. Viscosity data for Al2O3-water nanofluid-hysteresis: is heat transfer enhancement using nanofluids reliable? Int J Therm Sci. 47:103–111.
- Pasdois P, Parker JE, Griffiths EJ, Halestrap AP. 2013. Hexokinase II and reperfusion injury: TAT-HK2 peptide impairs vascular function in Langendorff-perfused rat hearts. Circ Res. 112:e3–e7.
- Phupoksakul T, Leuangsukrerk M, Numpiboonmarn P, Somwangthanaroj A, Janjarasskul T. 2015. Properties of poly(lactide)-whey protein isolate laminated films. J Sci Food Agric. 95:715–721.
- Rahamathulla PM, Watanabe K, Ashraf M, Millard RW. 1985. Prevention of lactate production and myocyte injury in isolated rat hearts perfused with perfluorochemical emulsion. Exp Pathol. 28:157–165.
- Riess JG. 2001. Oxygen carriers (“blood substitutes”) – raison d'etre, chemistry, and some physiology. Chem Rev. 101:2797–2920.
- Roser M, Fischer D, Kissel T. 1998. Surface-modified biodegradable albumin nano- and microspheres. II: effect of surface charge on in vitro phagocytosis and biodistribution in rats. Eur J Pharm Biopharm. 46:255–263.
- Rosoff JD, Soltow LO, Vocelka CR, Schmer G, Chandler WL, Cochran RP, et al. 1998. A second-generation blood substitute (perfluorodichlorooctane emulsion) does not activate complement during an ex vivo circulation model of bypass. J Cardiothorac Vasc Anesth. 12:397–401.
- Schäfer V, Briesen H, Rübsamen-Waigmann H, Steffan AM, Royer C, Kreuter J, et al. 1994. Phagocytosis and degradation of human serum albumin microspheres and nanoparticles in human macrophages. J Microencapsul. 11:261–269.
- Schreckenberg R, Rebelo M, Deten A, Weber M, Rohrbach S, Pipicz M, et al. 2015. Specific mechanisms underlying right heart failure: the missing upregulation of superoxide dismutase-2 and its decisive role in antioxidative defense. Antioxid Redox Signal. 23:1220–1232.
- Segel LD, Ensunsa JD. 1988. Albumin improves stability and longevity of perfluorochemical-perfused hearts. Am J Physiol. 254:H1105–H1112.
- Segel LD, Follette DM, Iguidbashian JP, Contino JP, Castellanos LM, Berkhoff HA, et al. 1994. Posttransplantation function of hearts preserved with fluorochemical emulsion. J Heart Lung Transplant. 13:669–680.
- Sloviter HA, Kamimoto T. 1967. Erythrocyte substitute for perfusion of brain. Nature. 216:458–460.
- Sothornvit R, Krochta JM. 2000. Oxygen permeability and mechanical properties of films from hydrolyzed whey protein. J Agric Food Chem. 48:3913–3916.
- Spiess BD. 2009. Perfluorocarbon emulsions as a promising technology: a review of tissue and vascular gas dynamics. J Appl Physiol. 106:1444–1452.
- Sutherland FJ, Hearse DJ. 2000. The isolated blood and perfusion fluid perfused heart. Pharmacol Res. 41:613–627.
- Tsuchida E, Sou K, Nakagawa A, Sakai H, Komatsu T, Kobayashi K. 2009. Artificial oxygen carriers, hemoglobin vesicles and albumin − hemes, based on bioconjugate chemistry. Bioconjugate Chem. 20:1419–1440.
- Vercellotti GM, Hammerschmidt DE, Craddock PR, Jacob HS. 1982. Activation of plasma complement by perfluorocarbon artificial blood: probable mechanism of adverse pulmonary reactions in treated patients and rationale for corticosteroids prophylaxis. Blood. 59:1299–1304.
- Weiskopf RB. 2010. Hemoglobin-based oxygen carriers: compassionate use and compassionate clinical trials. Anesth Analg. 110:659–662.