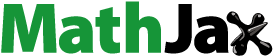
Abstract
Targeted entrance and accumulation of higher doses of drugs into malignant cells could help in intensification of tumor specific cytotoxicity. A dual-responsive nanogel, poly(N-isopropylacrylamide)-co-poly(N,N-(dimethylamino)ethyl methacrylate) [P(NIPAM-co-DMA)] containing N-isopropylacrylamide (NIPAM) as thermoresponsive monomer and N,N-(dimethylamino)ethyl methacrylate (DMA) as pH-responsive monomer and methylene-bis-acrylamide (MBA) as cross-linking agent, was synthesized by free radical emulsion polymerization. Cisplatin along with magnetic Fe3O4 nanoparticles (MNPs) was loaded into the nanogel by physically embedding the magnetic nanoparticles into hydrogel matrix after gelation to obtain drug-loaded magnetic nanocomposite [P(NIPAM-co-DMA)/Fe3O4]. Drug loading efficiencies and drug release profiles of cisplatin-loaded P(NIPAM-co-DMA) nanogel and P(NIPAM-co-DMA)/Fe3O4 nanocomposite were evaluated in vitro for controlled drug delivery in different temperature and pH conditions. Finally, the anticancer activity of P(NIPAM-co-DMA)/Fe3O4 nanocomposite on human liver HepG2 cells was evaluated. Nanogel and nanocomposite showed significantly higher (p < .05) cisplatin release at 40 °C compared to 37 °C and at pH 5.7 compared to pH 7.4, demonstrating their temperature and pH sensitivity, respectively. The cytotoxicity assay of drug free nanogel on HepG2 cell line indicated that the nanogel is biocompatible and suitable as drug carrier. Moreover, MTT assay revealed that the cisplatin-loaded nanocomposite represented significant superior cytotoxicity (p < .05) to HepG2 cells as compared with free cisplatin.
Introduction
Cisplatin, cis-diamminedichloroplatinum (II) (CDDP), is a chemotherapy drug used in the treatment of solid tumors [Citation1,Citation2]. It exerts biological activity when it enters the cell and subsequently binds to DNA [Citation3] but cisplatin shows significant dose-related side effects such as nephrotoxicity, neurotoxicity, nausea and vomiting [Citation4]. In order to maintain the efficacy, improve accumulation of drug, targeting tumor cells and reduce the undesired disadvantages of cisplatin, nanoparticle-based drug delivery systems were designed [Citation5,Citation6].
The nanoscale size of nanocarrier systems allows the permeability and retention of drugs in solid tumors. Macromolecules and nanoparticles because of the disorganized vasculature and absence of effective lymphatic drainage in solid tumours can accumulate more in tumours than in normal tissues (EPR effect) [Citation7,Citation8]. Among the various drug nanocarriers, synthetic polymer nanoparticles have devoted more attention for reason of their excellent properties including biocompatibility and biodegradability particularly easy chemical modification or surface functionalization [Citation9,Citation10].
A large number of these nanoparticles can respond to various stimulus such as temperature [Citation11], magnetic [Citation12], light [Citation13], pH [Citation14], enzymes [Citation15], glucose [Citation16], etc. These are termed as “smart” or “intelligent” [Citation17,Citation18]. Introducing stimulus-responsive property to synthetic polymer nanoparticles can lead to better therapeutic applications. Poly(N-isopropylacrylamide) (PNIPAM) is one of the well-known thermo-responsive polymers that exhibits a low critical solution temperature (LCST) in water. It undergoes a sharp coil–globule phase transition around the LCST, whereby the polymer becomes soluble below the LCST and precipitates over the LCST [Citation19–22]. Hydrogels are polymeric chains with either physical or chemical cross-links that can absorb high water content. Submicron-sized hydrogel particles are known nanogels [Citation23–25]. Advantages of a hydrogel such as biocompatibility, a large surface area and an interior network besides thermo-responsibility of PNIPAM have been caused the hydrogels based on PNIPAM to be most utilized in stimuli-responsive drug delivery systems [Citation26–29]. These hydrogels are swollen in aquous media under the LCST and can incorporate drug in their interior network however at the temperature higher than the LCST, they undergo sudden shrinkage and release it [Citation30].
It is well known that pH value in tumors – due to the high rate of glycolysis in cancer cells – is lower than in normal tissues. Therefore, pH-sensitive drug delivery systems have been most widely used in cancerous diseases [Citation31–33]. By using the monomers containing ionizable chemical groups in synthesis of a hydrogel, any change in pH medium can lead to change in its properties such as swelling ratio, resulting in drug release [Citation34,Citation35]. A dual pH/temperature-sensitive hydrogel can be synthesized using pH- and temperature-sensitive monomers [Citation36,Citation37].
In the present study, we synthesized the smart nanogel utilizing N,N-(dimethylamino)ethyl methacrylate and N-isopropylacrylamide (NIPAM) as pH and temperature responsive monomers, respectively. The properties of the synthesized nanogel such as structure and swelling ratio have been investigated. Cisplatin with and without magnetic Fe3O4 nanoparticles (MNPs) was loaded into the nanogel. We added magnetic nanoparticles to nanogl to obtain magnetic nanocomposite because the magnetic nanoparticles offer the benefit of utilizing both passive targeting (the EPR effect) and active targeting; in the presence of external magnets, the magnetic nanocomposite could be accumulated in specific regions, release the drug, and that inhibit cell growth [Citation38–49]. The release profiles of cisplatin were carried out by a dialysis method, and analysis with UV spectrophotometer in different temperature and pH conditions and the results were compared. Also we studied the cytotoxicity of cisplatin-loaded nanocomposite on human liver cancer cell line HepG2.
Experimental
Materials
N-isopropylacrylamide was purchased from Acros Organics (Morris Plains, NJ, USA), and purified by recrystallization in n-hexane. N,N-(dimethylamino)ethyl methacrylate (DMA), N,N′-methylene-bis-acrylamide (MBA) and potassium persulfate (KPS) were purchased from Merck (Darmstadt, Germany). Sodium dodecyl sulfate (SDS), sodium mono hydrogen phosphate and dihydrogen phosphate were received from Sigma-Aldrich (St. Louis, MO). Magnetic Fe3O4 nanoparticles were obtained from US Research Nanomaterials Inc. (Houston, TX). Cis-diaminedichloroplatinum (II) (CDDP) was obtained from Merck (Darmstadt, Germany). Also RPMI1640 (Gibco, Carlsbad, CA) and foetal bovin serum (FBS) (Gibco, Carlsbad, CA), Penstrep (Biosera, East Sussex, UK) and MTT (3-(4,5-dimethylthiazol-2-yl)-2,5-diphenyltetrazolium bromide) powder (Sigma, St. Louis, MO) as well as routine cell culture vessels were employed for in vitro studies. All other chemicals and solvents were commercially available and of analytical grade.
Preparation of P(NIPAM-co-DMA)
P(NIPAM-co-DMA) copolymer was prepared by free radical emulsion polymerization. NIPAM (3.80 g), DMA (0.369 g), cross-linking agent MBA (0.125 g, 3 wt% of total monomers), SDS (0.05 g/100 ml) as emulsifier and H2O (230 ml) were added to a 500 ml round-bottom flask with a magnetic stirrer, condenser and nitrogen inlet. The solution is degassed in room temperature for 30 min, then was heated at 70 °C. KPS (0.166 g) in 20 ml H2O was added to initiate polymerization. The polymerization reaction proceeded for 4 h at 68–70 °C. After polymerization, the product was dialysed for a week to remove the surfactant and unreacted molecules. Deionized water in a dialysis bag (molecular weight cut-off at 12 kDa, Sigma-Aldrich, St. Louis, MO) was exchanged three time in every day. The obtained copolymer was freeze-dried and stored for further uses.
Physicochemical characterization
Chemical structure of P(NIPAM-co-DMA)
The 1H NMR spectrum of P(NIPAM-co-DMA) was recorded on a Bruker-NMR 400 MHz spectrometer in deuterated chloroform (Billerica, MA). Also FTIR spectral analysis was carried out for structural characterization of copolymer. The FTIR spectrum of sample was recorded on a FTIR spectrometer in the range of 4000–400 cm−1 (Perkin Elmer 983 spectrometer, Waltham, MA).
Determination of LCST
To determine the LCST of P(NIPAM-co-DMA), optical transmittance of the copolymer solution (2 mg/ml) after complete swelling was measured at a wavelength of 500 nm with a UV spectrophotometer (2550 Shimadzu spectrophotometer, Kyoto, Japan) and recorded as a function of temperature from 25 to 50 °C. The LCST of the P(NIPAM-co-DMA) was defined as the temperature producing 50% in transmittance.
Morphological studies
Morphology of hydrogels with and without drug was studied by scanning electron microscopy (SEM). One drop of samples was placed on aluminum foil and dried. After vacuum coated with a thin layer of gold, the samples were examined at an acceleration voltage of 20 kV (MIRA3 FEG-SEM, Tescan, Brno, Czech Republic).
Vibrating sample magnetometry (VSM) analysis
A vibrating sample magnetometer (Meghnatis Daghigh Kavir Co., Kashan, Iran) was used to study the magnetic property of magnetic nanocomposite at room temperature.
Particle size and zeta potential measurements
Measurements of particle size and zeta potential of the samples were performed on a Zetasizer (Nano ZS ZEN 3600, Malvern Instruments, Malvern, UK) by dynamic light scattering measurements at room temprature. Samples were prepared as 0.5% (w/v). The zeta potential was used to characterize the surface charge properties of samples.
Measurement of swelling behaviour
The gravimetric method was used for the swelling measurements of copolymer. The samples of P(NIPAM-co-DMA) were submerged in distilled water at different levels of pH (7.4, 5.8) and temperature (37 °C, 40 °C), and left to equilibrate for 24 h. After removing excess water by filter paper, the samples were weighted. The swelling ratio was obtained using the following formula:
where WS is the weight of swollen gel and Wd is the weight of dry gel.
Drug loading
For preparation of cisplatin-loaded magnetic nanocomposite, 100 mg of freeze-dried P(NIPAM-co-DMA) floated in 2 ml H2O for 24 h at room temperature to swell. Then 10 mg cisplatin along with 2 mg of Fe3O4 nanoparticles were added and sonicated for 15 min at 0.5 cycle and 50% amplitude (Sonicator, Hielscher, Teltow, Germany). The solution was stirred for 72 h at room temperature. Then the solution was heated above LCST. Drug-loaded magnetic nanocomposite was collected, freeze-dried and stored for further applications. Cisplatin-loaded hydrogel was also prepared by the same method in the absence of magnetic nanoparticles. The supernatants were then analysed using UV spectrophotometer to determine the amount of unencapsulated cisplatin (λmax 295 nm). These values were utilized to obtain cisplatin-encapsulation efficiency of the hydrogel in the presence as well as absence of magnetic nanoparticles. Encapsulation efficiency (EE %) was calculated according to the following equation:
Release profiles of cisplatin as a function of temperature
Ten milligrams of lyophilized cisplatin-loaded samples were suspended in 8 ml of phosphate buffered saline (PBS) in test tubes with two pH values (7.4 and 5.8). The solutions were then incubated at temperatures 37 °C and 40 °C. At predetermined time intervals, the test tubes were centrifuged at 18,000×g for 10 min. Then 2 ml supernatants were withdrawn and analysed using UV spectrophotometer and replaced by the same quantities of fresh PBS solutions. The concentration of cisplatin released from the samples was expressed as a percentage of the total cisplatin and plotted against time.
Cell culture and cytotoxicity assessment
Human hepatocellular carcinoma cell line (HepG2) from Iranian Pasteur Institute Cell bank was cultivated in RPMI1640 medium, enriched with 10% FBS as well as standard concentrations of PenStrep in 5% CO2 humidified, 37 °C incubator.
For cytotoxicity MTT assay, the cells were grown to confluence, were trypsinized and harvested. Then, 8 × 103 cells were seeded in 96 well plates and after 24 h, treated with doses (1000, 800, 400 and 200 μg/ml) or (0.001, 0.0025, 0.005, 0.01, 0.025, 0.05, 0.1 and 0.25 μM) of cisplatin-loaded P(NIPAM-co-DMA)/Fe3O4 nanocomposite. After 24, 48 and 72 h incubation, the media containing drugs were removed, then replaced with 2 mg/ml of MTT solution and incubated for 4 h in 37 °C in dark. The furmazan crystals were dissolved by DMSO and finally the absorbance was measured in 570 nm using an Elisa Reader (Abnova, Taipei, Taiwan).
Statistical analysis
All experiments were done in duplicate or triplicates, the one-way ANOVA was employed for significance analysis using SPSS software (SPSS Inc., Chicago, IL).
Results and discussion
Copolymer synthesis and characterization
P(NIPAM-co-DMA) copolymer was synthesized via free radical emulsion polymerization of NIPAM and DMA in the presence of KPS as initiator and MBA as cross-linking agent (Scheme 1). The structure of copolymer was confirmed by 1H NMR and FTIR spectra. shows the 1H NMR spectrum of the copolymer. The chemical shifts of proton resonances were assigned as follows (δ ppm): 1.102 (CH(CH3)2), 2.053 (CHCO), 3.963 (CH(CH3)2) for NIPAM and 2.631 (CH2N), 4.088 (CH2O), 2.295 ((CH3)2 N) for DMA. The protons of copolymer backbone appeared at 1.565–1.970.
The FTIR spectrum of copolymer confirmed the presence of characteristic functional groups of components. As shown in , the characterstic peak of NIPAM amide group appears at 1640–1660 cm−1. The peaks at 3300–3500 cm−1 and 1375 cm−1 are assigned to the N–H and methyl groups of isopropyle in NIPAM, respectively. The peak at 1735 cm−1 is the stretching vibration of carbonyl group in DMA, and the absorbance band 2950 cm−1 is related to stretching aliphatic C–H bonds.
Thermal properties of copolymer
LCST of P(NIPAM-co-DMA) was measured by transmittance method. The LCST of P(NIPAM-co-DMA) was about 39 °C which was higher than the LCST of pure PNIPAM (32 °C). Introducing of hydrophilic monomer such as DMA can raise the LCST of NIPAM-based copolymers [Citation42]. The LCST of P(NIPAM-co-DMA) was raised to about 39 °C by adjusting the molar ratio of NIPAM to DMA (14:1). When the temperature increases above the LCST of P(NIPAM-co-DMA), it would undergo a shrinkage in the reason of interruption of the hydrogen bonds between polymer chains and water molecules.
Morphology and particle size studies
In order to study the morphology of synthesized cross-linked copolymer and drug-loaded magnetic nanocomposite, SEM images were used. shows the morphology of cross-linked copolymer. As shown in , both the cross-linked hydrogel copolymer and drug-loaded magnetic nanocomposite have spherical morphology.
Figure 3. SEM micrographs of P(NIPAM-co-DMA) nanogel (A) and cisplatin-loaded P(NIPAM-co-DMA)/Fe3O4 nanocomposite (B).
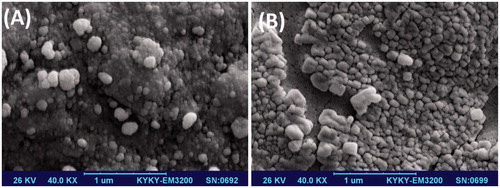
The average diameters of cross-linked copolymer hydrogel (nanogel) and cisplatin-loaded magnetic nanocomposite are about 90 nm and 150 nm, respectively. The increase of nanocomposite size comparing to nanogel, could be related to the incorporation of drug and magnetic nanoparticles into the nanogel matrix.
shows the particle size distribution of nanogel and cisplatin-loaded magnetic nanocomposite determined by DLS. The particle size and zeta potential of the P(NIPAM-co-DMA) nanogel without cisplatin loaded were about 167 nm and 70 mV, respectively. When cisplatin and magnetic nanoparticles were loaded into the P(NIPAM-co-DMA), the particle size increased to 189 nm and the zeta potential decreased to 0.7 mV. A positive charge is assigned to the existence of DMA moieties in the nanogel. Due to the swelling of nanogel and nanocomposite in solution, DLS technique shows larger average hydrodynamic particle size compared to SEM. This was because DLS determined the hydrodynamic diameter of the samples in solution, while SEM measured the diameter of dried samples.
Magnetization studies
The magnetic property of drug-loaded nanocomposite was investigated with a VSM. As shown in , saturation magnetization of cisplatin-loaded P(NIPAM-co-DMA)/Fe3O4 was found to be 1.2 emu/g. The obtained value is lower than the value of 74 emu/g that measured for MNPs as a reference value. illustrates the separation and redispersion behaviour of the cisplatin-loaded magnetic nanocomposite. In the absence of an external magnetic field, the dispersion of magnetic nanocomposite is dark and homogeneous. When the external magnetic field was applied, magnetic nanocomposite was attracted to the side wall of test tube and the dispersion became transparent. These results indicate that Fe3O4 nanoparticles have been successfully encapsulated in hydrogel matrix. When an external magnetic field was removed, the magnetic nanocomposite could be redispersed in water by shaking. Therefore, magnetic nanocomposite with magnetic responsivity can be used in targeted drug delivery systems.
Swelling behaviour
shows the swelling ratio of the P(NIPAM-co-DMA) nanogel at two pH and temperature values. As could be seen, the swelling ratio varies inversely with temperature and pH. When temperature increases above the LCST, the hydrophobic interactions in PNIPAM become dominant resulting in the decrease of hydrogen bonding between polymer and water, and hence the collapse of the polymer structure. Also with decreasing pH, the amine group in DMA is protonated and electrostatic repulsion between positively charged ammonium side groups led to more swelling of the nanogel.
Table 1. Swelling ratio of the P(NIPAM-co-DMA) nanogel at two different temperature and pH values.
Encapsulation efficiency and drug release behaviour
Cisplatin was loaded into the P(NIPAM-co-DMA) nanogel and its magnetic nanocomposite [P(NIPAM-co-DMA)/Fe3O4)] with encapsulation efficiencies of 26.03% and 40.3%, respectively. The incorporation of Fe3O4 magnetic nanoparticles increased the cisplatin encapsulation efficiency probably because of the presence of hydroxyl functional groups on Fe3O4 nanoparticles. ) shows the release curves of cisplatin-loaded P(NIPAM-co-DMA) nanogel and P(NIPAM-co-DMA)/Fe3O4 nanocomposite at two different temperature and pH values for 10 days (240 h). Both curves follow the same pattern. The higher cumulative drug release at 40 °C (above LCST) is the reason for the collapse of nanogel while at 37 °C the nanogel is still in a swollen state and cisplatin release occurs due to the diffusion, hence it is slow. Also at pH 5.8, the cumulative drug release is higher than pH 7.4. In acidic medium, the amino group of DMA is protonated, thus leading to more swelling of hydrogel and more drug release.
Cytotoxicity studies
The cell viability/toxicity assay is necessary for evaluation of a new drug delivery system in real samples. The biocompatibility and cytotoxicity of P(NIPAM-co-DMA) nanogel and cisplatin-loaded P(NIPAM-co-DMA)/Fe3O4 nanocomposite were studied using MTT assay. The data showed that cell viability was not affected by P(NIPAM-co-DMA) nanogel in different concentrations (), therefore, it can be considered safe for drug delivery applications. In 24 h, free cisplatin showed higher cell toxicity in concentrations of 0.025–0.25 μM () but after 48 or 72 h, the nanocomposite showed significant higher cytotoxicity (p < .05) even in doses as low as 0.0025 μM ()). IC50 of cisplatin and nanocomposite were 0.05 and 0.01, respectively ().
Figure 8. The HepG2 cells in cultivation medium (A). The cytocompatibility checking of P(NIPAM-co-DMA) nanogel using MTT assay which showed no significant cytotoxicity to HepG2 cells (B).
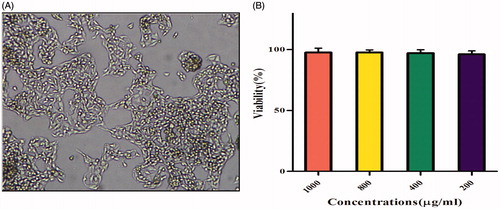
Figure 9. Growth inhibition rates by different concentration of free cisplatin and cisplatin-loaded P(NIPAM-co-DMA)/Fe3O4 nanocomposite after 24 (A), 48 (B) and 72 h (C). IC50 reduced to lower doses 72 h post incubation (D).
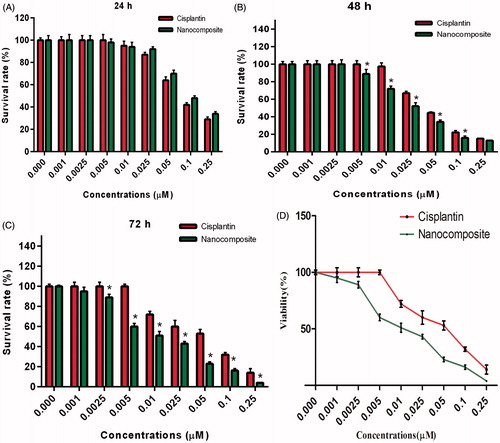
Reduced cell survival and induction of apoptosis by cisplatin-loaded P(NIPAM-co-DMA)/Fe3O4 nanocomposite in HepG2 tumor cells would be a benefit in lowering the dose of chemotherapeutic agents for patients, subsequently reduction of higher dose-dependent side effects. The nanocomposite improved delivery and accumulation of cisplatin into HepG2 cells.
Conclusions
The ultimate goal of nanocarrier-based cancer therapy is to develop nanocarriers and targeting moieties in which chemotherapeutic agents, could be loaded for smart, effective and safe treatments. In the present study, a pH/temprature-responsive P(NIPAM-co-DMA) nanogel was synthesized by free radical emulsion polymerization. Cisplatin along with MNPs was loaded into the nanogel, by physically embedding of them into the nanogel matrix after gelation. The results of VSM represented that the nanocomposite showed superparamagnetic property. In vitro drug release was higher at pH 5.8, 40 °C than pH 7.4, 37 °C (physiologic conditions). The in vitro cytotoxicity tests of P(NIPAM-co-DMA) nanogel demonstrated no significant toxic effects against HepG2 cell line, hence it could be suggested as a safe drug carrier. Furthermore, cisplatin-loaded P(NIPAM-co-DMA)/Fe3O4 nanocomposite showed a remarkably augmented efficiency because of its improved delivery and accumulation for causing apoptosis in HepG2 cells. Developed drug delivery system offers a smart release of anti-tumor drugs, higher drug loading and release, therefore, provides a promising delivery system to effectively stop tumor growth and reduce undesired side effects of the drugs over other healthy tissues.
Disclosure statement
No potential conflict of interest was reported by the authors.
References
- Li X, Li R, Qian X, et al. Superior antitumor efficiency of cisplatin-loaded nanoparticles by intratumoral delivery with decreased tumor metabolism rate. Eur J Pharm Biopharm. 2008;70:726–734.
- Wheate NJ, Walker S, Craig GE, et al. The status of platinum anticancer drugs in the clinic and in clinical trials. Dalton Trans. 2010;39:8113–8127.
- Cepeda V, Fuertes MA, Castilla J, et al. Biochemical mechanisms of cisplatin cytotoxicity. ACAMC. 2007;7:3–18.
- Yao X, Panichpisal K, Kurtzman N, et al. Cisplatin nephrotoxicity: a review. Am J Med Sci. 2007;334:115–124.
- Hu J, Johnston KP, Williams IIIRO. Nanoparticle engineering processes for enhancing the dissolution rates of poorly water soluble drugs. Drug Dev Ind Pharm. 2004;30:233–245.
- Eatemadi A, Darabi M, Afraidooni L, et al. Comparison, synthesis and evaluation of anticancer drug-loaded polymeric nanoparticles on breast cancer cell lines. Artif Cells Nanomed Biotechnol. 2016;44:1008–1017.
- Gaudreault J, Shiu V, Bricarello A, et al. Concomitant administration of bevacizumab, irinotecan, 5-fluorouracil, and leucovorin: nonclinical safety and pharmacokinetics. Int J Toxicol. 2005;24:357–363.
- Ebrahimi E, Akbarzadeh A, Abbasi E, et al. Novel drug delivery system based on doxorubicin-encapsulated magnetic nanoparticles modified with PLGA-PEG1000 copolymer. Artif Cells Nanomed Biotechnol. 2016;44: 290–297.
- Gorjikhah F, Azizi Jalalian FR, Salehi R, et al. Preparation and characterization of PLGA-β-CD polymeric nanoparticles containing methotrexate and evaluation of their effects on T47D cell line. Artif Cells Nanomed Biotechnol. 2017;45:432–440.
- Aberoumandi SM, Mohammadhosseini M, Abasi E, et al. An update on applications of nanostructured drug delivery systems in cancer therapy. Artif Cells Nanomed Biotechnol. 2016;45:1058–1068.
- Berndt I, Popescu C, Wortmann F-J, et al. Mechanics versus thermodynamics: swelling in multiple-temperature-sensitive core–shell microgels. Angew Chem Int Ed Engl. 2006;45:1081–1085.
- Rahimi M, Shojaei S, Safa KD, et al. Biocompatible magnetic tris(2-aminoethyl)amine functionalized nanocrystalline cellulose as a novel nanocarrier for anticancer drug delivery of methotrexate. New J Chem. 2017;41:2160–2168.
- Ercole F, Davis TP, Evans RA. Photo-responsive systems and biomaterials: photochromic polymers, light-triggered self-assembly, surface modification, fluorescence modulation and beyond. Polym Chem. 2010;1:37–54.
- Batrakova EV, Kabanov AV. Pluronic block copolymers: evolution of drug delivery concept from inert nanocarriers to biological response modifiers. J Control Release. 2008;130:98–106.
- Ulijn RV. Enzyme-responsive materials: a new class of smart biomaterials. J Mater Chem. 2006;16:2217–2225.
- Kang SI, Bae YH. A sulfonamide based glucose-responsive hydrogel with covalently immobilized glucose oxidase and catalase. J Control Release. 2003;86:115–121.
- Bazmi F, Salehi R, Alizadeh E, et al. pH-Controlled multiple-drug delivery by a novel antibacterial nanocomposite for combination therapy. RSC Adv. 2015;5:105678–105691.
- Gao GH, Li Y, Lee DS. Environmental pH-sensitive polymeric micelles for cancer diagnosis and targeted therapy. J Control Release. 2013;169:180–184.
- Davaran S, Akbarzadeh A, Nejati-Koshki K, et al. In vitro studies of NIPAAM-MAA-VP copolymer-coated magnetic nanoparticles for controlled anticancer drug release. JEAS. 2013;108–115.
- Huang G, Gao J, Hu Z, et al. Controlled drug release from hydrogel nanoparticle networks. J Control Release. 2004;94:303–311.
- Luo S, Xu J, Zhu Z, et al. Phase transition behavior of unimolecular micelles with thermoresponsive poly(N-isopropylacrylamide) coronas. J Phys Chem B. 2006;110:9132–9139.
- Wei H, Chen W-Q, Chang C, et al. Synthesis of star block, thermosensitive poly(l-lactide)-star block-poly(N-isopropylacrylamide-co-N-hydroxymethylacrylamide) copolymers and their self-assembled micelles for controlled release. J Phys Chem C. 2008;112:2888–2894.
- Peng J, Qi T, Liao J, et al. Controlled release of cisplatin from pH-thermal dual responsive nanogels. Biomaterials. 2013;34:8726–8740.
- Kurd K, Khandagi AA, Davaran S, et al. Cisplatin release from dual-responsive magnetic nanocomposites. Artif Cells Nanomed Biotechnol. 2016;44:1031–1039.
- Kanjickal D, Lopina S, Evancho-Chapman MM, et al. Improving delivery of hydrophobic drugs from hydrogels through cyclodextrins. J Biomed Mater Res A. 2005;74:454–460.
- Constantin M, Bucatariu S, Ascenzi P, et al. Poly(NIPAAm-co-β-cyclodextrin) microgels with drug hosting and temperature-dependent delivery properties. React Funct Polym. 2014;84:1–9.
- Li P, Xu R, Wang W, et al. Thermosensitive poly(N-isopropylacrylamide-co-glycidyl methacrylate) microgels for controlled drug release. Colloids Surf B Biointerfaces. 2013;101:251–255.
- Zhang X-Z, Yang Y-Y, Chung T-S, et al. of Fast response macroporous poly(N-isopropylacrylamide) hydrogels. Langmuir. 2001;17:6094–6099.
- Zeighamian V, Darabi M, Akbarzadeh A, et al. PNIPAAm-MAA nanoparticles as delivery vehicles for curcumin against MCF-7 breast cancer cells. Artif Cells Nanomed Biotechnol. 2016;44:735–742.
- Akbarzadeh A, Mikaeili H, Zarghami N, et al. Preparation and in vitro evaluation of doxorubicin-loaded Fe3O4 magnetic nanoparticles modified with biocompatible copolymers. Int J Nanomedicine. 2012;7:511–526.
- Du J-Z, Du X-J, Mao C-Q, et al. Tailor-made dual pH-sensitive polymer–doxorubicin nanoparticles for efficient anticancer drug delivery. J Am Chem Soc. 2011;133:17560–17563.
- Kim MS, Hwang SJ, Han JK, et al. pH-responsive peg-poly(β-amino ester) block copolymer micelles with a sharp transition. Macromol Rapid Commun. 2006;27:447–451.
- Song W, Tang Z, Li M, et al. Tunable pH-sensitive poly(β-amino ester)s synthesized from primary amines and diacrylates for intracellular drug delivery. Macromol Biosci. 2012;12:1375–1383.
- Das M, Mardyani S, Chan WCW, et al. Biofunctionalized pH-responsive microgels for cancer cell targeting: rational design. Adv Mater. 2006;18:80–83.
- Zhang K, Wang Z, Li Y, et al. Dual stimuli-responsive N-phthaloylchitosan-graft-(poly(N-isopropylacrylamide)-block-poly(acrylic acid)) copolymer prepared via RAFT polymerization. Carbohyd Polym. 2013;92:662–667.
- Bhattacharya D, Behera B, Sahu SK, et al. Design of dual stimuli responsive polymer modified magnetic nanoparticles for targeted anti-cancer drug delivery and enhanced MR imaging. New J Chem. 2016;40:545–557.
- Su S, Wang H, Liu X, et al. iRGD-coupled responsive fluorescent nanogel for targeted drug delivery. Biomaterials. 2013;34:3523–3533.
- Davaran S, Alimirzalu S, Nejati-Koshki K, et al. Physicochemical characteristics of Fe3O4 magnetic nanocomposites based on poly(N-isopropylacrylamide) for anti-cancer drug delivery. Asian Pac J Cancer Prev. 2014;15:49–54.
- Wang S, Zhou Y, Sun W. Preparation and characterization of antifouling thermosensitive magnetic nanoparticles for applications in biomedicine. Mater Sci Eng C. 2009;29:1196–1200.
- Wagstaff AJ, Brown SD, Holden MR, et al. Cisplatin drug delivery using gold-coated iron oxide nanoparticles for enhanced tumour targeting with external magnetic fields. Inorg Chim Acta. 2012;393:328–333.
- Kim E, Lee K, Huh Y-M, et al. Magnetic nanocomplexes and the physiological challenges associated with their use for cancer imaging and therapy. J Mater Chem B. 2013;1:729–739.
- Zhang J, Chen H, Xu L, et al. The targeted behavior of thermally responsive nanohydrogel evaluated by NIR system in mouse model. J Control Release. 2008;131:34–40.
- Khalilov RI, Ahmadov IS, Kadirov SG. Two types of kinetics of membrane potential of water plant leaves illuminated by ultraviolet light. Bioelectrochemistry. 2002;58:189–191.
- Khalilov RI, Khomutov GB, Tikhonov AN. Effect of ultraviolet radiation on structural-functional characteristics of the thylakoid membrane. Russian Plant Physiol. 1993;3:338–342.
- Youssef NA, Gurbanov EM, Haciyeva SR, et al. Antioxidant enzymes, fluctuating asymmetry and morphological changes of urban trees as an ecological indicator of heavy metal stress. Int J Pharm Sci Health Care. 2013;1:1–18.
- Khalilov RI, Nasibova AN, Serezhenkov VA, et al. Accumulation of magnetic nanoparticles in plants grown on soils of Apsheron peninsula. Biophysics. 2011;56:316–322.
- Khalilov RI, Akhmetov IS, Goldfeld MG. Binary response of the membrane-potential in the leaf-cells of vallisneria spiralis on pulsed UV excitation. Doklady Akad Nauk. 1992;4:850–852.
- Goldfeld MG, Timofeev VP, Khalilov RI. Effect of orientation in a magnetic-field on the ESR-II signal shape in photosynthetic systems. Doklady Akad Nauk SSSR. 1979;1:235–237.
- Rovshan K, Aygun N. The use of EPR signals of plants as bioindicative parametersin the study of environmental pollution. Int J Pharm Pharm Sci. 2015;7:172–175.