Abstract
The tissue engineering field has developed in response to the shortcomings related to the replacement of the tissues lost to disease or trauma: donor tissue rejection, chronic inflammation and donor tissue shortages. The driving force behind the tissue engineering is to avoid the mentioned issues by creating the biological substitutes capable of replacing the damaged tissue. This is done by combining the scaffolds, cells and signals in order to create the living, physiological, three-dimensional tissues. A wide variety of skin substitutes are used in the treatment of full-thickness injuries. Substitutes made from skin can harbour the latent viruses, and artificial skin grafts can heal with the extensive scarring, failing to regenerate structures such as glands, nerves and hair follicles. New and practical skin scaffold materials remain to be developed. The current article describes the important information about wound healing scaffolds. The scaffold types which were used in these fields were classified according to the accepted guideline of the biological medicine. Moreover, the present article gave the brief overview on the fundamentals of the tissue engineering, biodegradable polymer properties and their application in skin wound healing. Also, the present review discusses the type of the tissue engineered skin substitutes and modern wound dressings which promote the wound healing.
Introduction
Diseases and disorders led to devastating consequences and organ failures which portray a life-threatening state. Two main approaches which were used to replace or repair the damaged or lost tissue and organs are autografting or allografting. Limitations on autografts, such as donor site morbidity and limited availability, are considered as the drawbacks of these approaches, and although allografts are not limited in supply, they show potential to provoke a strong response from the immune system and pose the transmission of disease risk, too [Citation1,Citation2].
It is important to consider that through the present time, tissue engineering, as an outstanding method for the repair/regeneration of damaged tissue, has been considered as an approach with the potential to transcend the limitations of both autologous and allogenic tissue repair [Citation3].
Biomaterials, as the 3D synthetic frameworks in tissue engineering, are commonly referred to as scaffolds, matrices or constructs and provide an opportunity for the cell attachment, proliferation and ingrowth ultimately leading to form the new tissue ().
Figure 4. The hybrid porous scaffolds of the PCL/PLLA/collagen I were fabricated by the freeze-drying method.
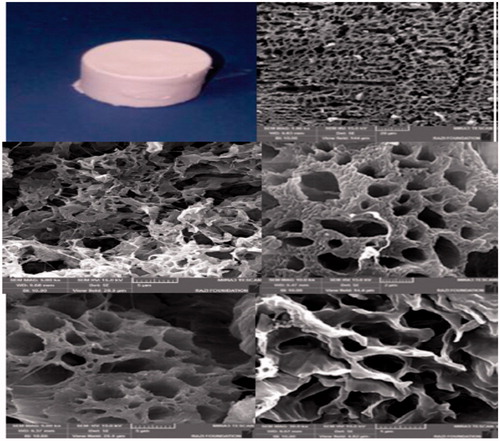
Figure 5. The hybrid porous scaffold of the PCL/collagen I was fabricated by the freeze-drying method.
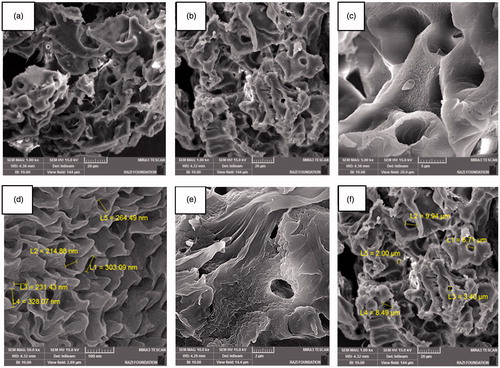
Materials used for fabricating the scaffold can be classified as the synthetic or natural and degradable or nondegradable, depending on the proposed usage. The properties of the polymers were influenced by some factors, including composition, structure and arrangement of their constituents [Citation4].
Both synthetic polymers and biological-based polymers, as the biodegradable polymeric biomaterials, have been investigated extensively [Citation5,Citation6]. The mentioned synthetic polymers included the suitable flexibility since the structure and composition could be tailored to the specific needs [Citation7]. Some innovative methods were established for the fabrication of the biomaterial-based three-dimensional scaffolds [Citation8–10]. The scaffolds with the high surface area to volume ratio helped the adhesion, proliferation, migration and differentiation of the cells, all of which were deemed as the extremely desired features for tissue engineering [Citation11,Citation12]. The present article was intended to illustrate the various polymers, natural and synthetic, which were used to produce the scaffolds in the field of skin tissue engineering. It covers the most commonly different hybrid scaffolds which were used for skin tissue engineering and wound healing. Also, through the present review, an attempt has been made to consolidate the different tissue-engineered skin substitutes and types of the wound dressings.
Biological polymers employed for skin tissue engineering
A large variety of skin substitutes were used in treating the full-thickness injuries. Substitutes derived from the skin might harbour the dormant viruses, and artificial skin grafts could heal with the hideous scarring, failing to the regenerate glands, nerves and hair follicles [Citation13]. Several three-dimensional porous scaffolds fabricated from the various kinds of biodegradable materials have been developed and used for many fields of the tissue engineering [Citation14–20]. The present part of the review summarizes the various properties of the biological polymers which were used in the skin tissue engineering.
Collagen
Collagen could be obtained from the animal tissues, and it could also be made by the recombinant methods [Citation21]. It was characterized by the high mechanical strength, adequate biocompatibility, low antigenicity and innate ability of being cross-linked, and tailored for its mechanical, degradation and water uptake features [Citation22,Citation23]. Drawbacks of the collagen comprised difficulty in processing and sterilization and also hard to regulate the extent and rate of degradability [Citation24]. Collagen as the major protein factor of the extracellular matrix (ECM) strongly supported the connective tissues such as skin, tendons, bones, cartilage, blood vessels and ligaments [Citation25–29]. Scaffold features could be varied by using the various concentrations of collagen [Citation30]. For instance, bilayered collagen gels, which were seeded with the human fibroblasts in the lower part and human keratinocytes in the upper layer, as the “dermal” matrix of an artificial skin product have been commercialized under the name of Apligraf® [Citation31]. Moreover, Biomend® was a collagen membrane that commonly used in the periodontal tissue regeneration [Citation32]. Chin et al. have reported an approach to build anatomically accurate, multicomponent skin substitutes in a cost-effective way [Citation33]. Glycosaminoglycans (GAGs), meanwhile, served a wide variety of functions such as linking collagen structures and binding growth factors [Citation34]. Integra is a bilaminate membrane consisting of a bovine collagen-based dermal analogue of glycosaminoglycans and chondroitin-6-sulphate and a temporary epidermal substitute layer of semipermeable silicone. It is the most widely used dermal substitute for burning the reconstruction. Glycosaminoglycan composition in this acellular dermal substitute was designed to control the rate of degradation [Citation35,Citation36]. In one of the studies about the Integra’s effectiveness in the treatment of the full-thickness and partial thickness injuries, Heimbach et al. used the Integra to treat 216 burn patients and assessed its safety and effectiveness [Citation37]. The results illustrated which Integra was a valuable, effective and safe treatment modality for managing challenging patients with the extensive burns.
Fibrin
Fibrin as the natural wound healing matrix, forms after injury and fibrinogen as a precursor of fibrin, could be obtained from the pooled plasma [Citation38]. Fibrin scaffolds skin to collagen contained some specific sites for the cell adhesion and the scaffold features varied depending on the concentration of fibrin used [Citation39,Citation40].
Fairly, rapid degradation is the most encountered drawback in the shape-specific scaffolds. Furthermore, fibrin could also be covalently modified to further change its properties [Citation41]. Fibrin is a versatile biological-based polymer which reveals an excellent potential in the tissue regeneration and wound healing because it, alone or in combination with other factors, has been considered as a biological scaffold for the stem or primary cells to regenerate bone, ligament, tendons, liver, cardiac tissue, cartilage, nervous tissue, ocular tissue and skin [Citation42].
Fibronectin
Fibronectin, derived from bovine or human plasma, is a glycoprotein with a high molecular weight, which can bind collagen, fibrin and heparin. Fibronectin could be found in its soluble form in blood and participates in the wound healing procedure [Citation43]. It can be aggregated to form mats, which could be applied as the scaffolds for the repair and regeneration of the neural tissue [Citation44,Citation45] ().
Gelatine
Gelatine, as a natural polymer, is derived from collagen [Citation42]. Under the specific circumstances, such as temperature, solvent or pH, gelatine macromolecules showed the adequate flexibility to come up to a great variety of the conformations. This made it feasible to vary also all the gelatine characteristics dependent on its molecular structure [Citation44]. Regarding the presence of both acidic and basic function at groups in gelatine macromolecules, it reveals largely the number of the structural variety than other synthetic polymers [Citation45]. Li et al. studied the gelatine with collagen, elastin and recombinant human tropoelastin in an experiment in which human embryonic palatal mesenchymal cells were seeded on all four scaffolds, and permitted to culture for 6 days. Gelatine helped cells to attach, migrate and proliferate for the duration of the test [Citation46]. Moreover, Huang et al. presented a bilayered gelatine chondroitin 6 sulphate–hyaluronic acid membrane with a different pore size. Chondroitin-6-sulfate and hyaluronic acid were combined within the gelatine membrane to mimic the skin composition and generated a suitable microenvironment for cell proliferation, differentiation and migration [Citation31].
Elastin
Elastin is an insoluble and hydrophobic protein which is utilized extensively in organs where shape and energy recovery are critical factors [Citation47]. Sell et al. produced electrospun 6 mm ID seamless tubes for testing the graft compliance. Dynamic compliance measurements created standards that ranged from 1.2 to 5.6%/100 mmHg for a set of three different mean arterial pressures, with the 50:50 ratios thoroughly mimicking the compliance of the natural femoral artery [Citation48]. Also, Jelena Rnjak-Kovacina et al. produced the composite scaffolds of collagen and tropoelastin by the electrospun technology for the dermal tissue engineering. This composite approach illustrated that the tropoelastin electrospinning system tended to modification and allowed for a range of anymore mechanical or biological properties to be engineered into the dermal substitute scaffolds [Citation49].
Chitosan
Chitosan has been the most plentiful polysaccharide found in environment, especially in the shell of crustacean, cuticles of insects and cell walls of fungi [Citation50]. Molecule of chitosan has amino and hydroxyl groups, and therefore, it can be modified chemically providing a chemical versatility and metabolized by the certain human enzymes [Citation51]. Chitosan can act as an ideal wound dressing as it exhibits a positive charge, film-forming capacity, mild gelation characteristics and a strong tissue adhesive property with an enhanced blood coagulation [Citation52]. It supports the wound healing by increasing the functions of inflammatory cells such as polymorph nuclear leukocytes, macrophages and fibroblasts [Citation53–55]. Chitosan has a potential use in skin repair and regeneration subsequent to injuries or burns because it could be cross-linked with the silica particles (SiO2) which used as a porogen agent and the extractions from the developed membranes demonstrated no cytotoxicity against L-929 cells 24 h after the culture. In addition, the macroporous membrane exhibited the excellent cellular adhesion and proliferation after 24 and 48 h of culturing [Citation56]. Chitin-based materials had also demonstrated their potential in keeping and exciting the cell phenotypes used in culturing the melanocytes, corneal keratinocytes and skin keratinocytes [Citation57,Citation58].
Keratin
Keratin is the main structural fibrous protein involved in hair, nails and skin [Citation59]. Cell adhesion sequences named RGD and LDV which are contained within the keratin protein might help the cell adhesion and growth on the keratin substrates [Citation60]. The immune response is reduced significantly when keratin is extracted from the human hair. In addition to the good biocompatibility, keratin source enjoyed the advantages of the readily available and less expensive [Citation61]. Additional detail investigations would raise the potential of the keratin as a beneficial biomaterial for using in skin tissue engineering [Citation62]. In the past decade, keratin-based research for the wound healing, drug delivery, tissue engineering, cosmetics and medical devices has been the most popular subjects [Citation4].
Alginate
Alginate was a natural polysaccharide illustrating very good biocompatibility and biodegradability, having many different applications in the field of biomedicine [Citation63]. Dressing was used to open wounds for centuries [Citation64]. It could prevent the wounds from more injury and bacterial infection [Citation65]. Alginate-based wound dressings such as sponges, hydrogels and electrospun mats were auspicious materials for the wound healing that had many advantages including haemostatic capability and gel-forming ability upon absorption of wound exudates [Citation66,Citation67]. Alginate was found to have many important features appropriate for a wound dressing such as good water absorptivity, conformability, optimal water vapour transmission rate and mild antiseptic properties coupled with the nontoxicity and biodegradability [Citation68]. It was recommended that the certain alginate dressings such as Kaltostat® could improve the wound healing by stimulating the monocytes to produce the raised levels of cytokines such as interleukin-6 and tumour necrosis factor-α [Citation69]. It has been also reported that an in situ forming hydrogel wound dressing could be prepared from gelatine and oxidized alginate in the presence of the small concentrations of borax [Citation70]. Moreover, concerning advantages of the alginate and water-soluble chitosan, a composite polysaccharide sponge was fabricated, resulting in an anti-adhesive and antimicrobial wound dressing [Citation71]. Also, alginates were extensively used for the tissue engineering scaffold fabrication [Citation72–74]. For example, the alginate microspheres [Citation75] or combination of the alginate, chitosan and chit oligosaccharides (alginate–chitosan–cos) and alginate chitosan, chit oligosaccharides with collagen (alginate–chitosan–cos–collagen) scaffold was used for the skin substitute and was illustrated that these scaffolds were improved the biocompatible nature that mimicked the functional environment of skin [Citation76–78].
Hyaluronic acid (hyaluronan)
Hyaluronic acid (HA) was a naturally derived, nonimmunogenic, nonadhesive glycosaminoglycan which played an important role in the various wound healing processes such as angiogenesis [Citation79]. Also, it promoted early inflammation which was the critical for initiating wound healing [Citation79]. Furthermore, hyaluronan presents unique advantages such as easy to produce and modify, hydrophilic, nonadhesive and naturally biodegradable. Because of these excellent specifications, HA found a number of usages in medicine and cosmetics; for example, the product Hyalgan manufactured by Fidia (Abano Terme, Italy) in the 1960 s was applied topically for the treatment of burns and skin ulcers [Citation80]. In addition to the abovementioned biological polymers, other natural polymers, such as C-phycocyanin (C-pc), also have been used in dermal wound healing [Citation81].
Nonbiological polymers employed for skin tissue engineering
Biological polymers could be considered as the first biodegradable biomaterials used clinically [Citation6]. Thereafter, the synthetic polymers were highly useful in the biomedical field since their properties (e.g. porosity, degradation time and mechanical characteristics) could be tailored for the specific applications [Citation82]. The current part of the article was discussed many synthetic polymer applications in the skin tissue engineering and wound healing.
POE
Poly(hydroxyortho esters) such as polyglycolic acid (PGA), polylactic acid (PLA) and their copolymers were applied for tissue engineering [Citation83]. Artificial skin frequently comprises animal material, such as collagen, which could contain the infectious substances [Citation24]. Biodegradable hydrogels were usually not physically robust enough to mimic to the epidermis. Polymer-based system frequently was used in skin scaffold synthesis and was described as a polyester-based lactic acid (PLA) and glycolic acid (PGA) [Citation84]. Nonetheless, these polyesters were revealed to degrade to their acidic components causing a high local acidity that might destroy proteins [Citation66]. The rapid degradation of this polymer could frequently hamper processing of the present material after exposure to aqueous media. To overcome these issues, the use of the PLA/PGA combinations was introduced. Blending of the poly-4-hydroxybutyrate with PLA and its copolymers was proven to increase the toughness and lower stiffness than PLA polymers or copolymers alone [Citation85]. Poly(d, l-lactic-co-glycolic acid) (PLGA), meanwhile an important amorphous biodegradable polyester, has been employed as the skeletal material since it enjoys the similar properties to those of the normal tissues [Citation86]. However, PLGA undergoes plastic deformation and failure when exposed to the long-term cyclic strain limiting use in the elastomeric tissue engineering [Citation87]. Three-dimensional (3D) scaffolds made from the synthetic and naturally derived biodegradable polymers such as PGA, PLA, PLGA and collagen have been commonly applied in the tissue engineering of the cartilage, bone, ligament and skin [Citation88].
PLA and PGA were striking objectives in the tissue engineering field for many reasons. They tended to “degrade” slowly, making them an attractive tool for using the internal fixation [Citation89]. “Degradation” in this manner is used to denote the mass loss because of resorption or dissolution of the biomaterial, precipitated or accompanied by the molecular weight decrease, structural variations and decline in the strength and stiffness [Citation90]. These materials offered a steady transfer of loads to the healing tissues [Citation91,Citation92]. Deependra Singh and Manju Rawat Singh optimized PLGA microspheres of gentamicin (GM) and serratiopeptidase (STP) have been incorporated into the PVA–gelatine slurry and casted into the films to prepare the multiphase hydrogel for the complete wound management. Their results suggested an accelerated re-epithelialization with the minimum disturbance of the wound bed [Citation93]. In the other work, Muthukumar Amirthalingam et al. fabricated the novel biocompatible PLGA–curcumin microparticle-embedded chitosan scaffold for wound healing application. Their results indicated that the existent scaffold could be used as a drug delivery system to treat the severe and chronic wounds [Citation94].
Polyethylene glycol
Polyethylene glycol (PEG), also recognized as polyethylene oxide (PEO) or polyoxyethylene (POE), was the most commercially important polyether, which referred to an oligomer or polymer of the ethylene oxide resisting protein adsorption and cell adhesion [Citation95]. These properties helped minimize the immune response following implantation [Citation96]. Moreover, the mentioned polymer could also be helpful to seal the cell membranes after injury, making it useful material for limiting cell death [Citation97]. Hydrophilic PEG hydrogels could be prepared through a range of cross-linking schemes to make the scaffolds with varying degradation as well as release rates [Citation98]. Further chemistry could be used to modify these gels to add the sites for cell adhesion or extracellular matrix (ECM) molecules to help cells to infiltrate into these scaffolds, extending their potential applications [Citation30]. Porous gelatine–poly(ethylene glycol) (PEG) was made by using the combination of freeze gelation and freeze extraction methods for the skin cells (fibroblast cells); finally, the high growth level of those cells and biocompatibility over the synthesized scaffold was seen [Citation99].
Poly-β-hydroxybutyrate
Poly-β-hydroxybutyrate (PHB) was considered as a linear head-to-tail homopolymer of (R)-β-hydroxybutiric acid which made the crystalline cytoplasmic granules in the large variety of bacteria [Citation100,Citation101]. The mentioned material was biodegradable and biocompatible microbial produced the polyester which, following implantation, degrades slowly at the body temperature and forms a nontoxic metabolite which was secreted in urine [Citation102]. PHB has been formerly used as a wound scaffolding device designed to support and protect a wound against more damage while promoting healing by encouraging the cellular growth on and within the device from the wound surface [Citation103]. Also, nanofibres were prepared by electrospinning from the blends of the polyvinyl alcohol and polyhydroxy butyrate and were potential scaffold material for the tissue engineering of skin [Citation104]. Saeed Heidari Keshel et al. has reported that the graft of the collagen-coated nanofibrous PHBV scaffold loaded with USSC (unrestricted somatic stem cells) showed the better results during the healing process of the skin defects in the rat model, so it was efficient skin grafts for the treatment of the acute full-thickness skin wounds [Citation105].
Poly(vinyl alcohol)
Due to poly(vinyl alcohol) (PVA) favourable properties such as nontoxicity, biocompatibility and biodegradability, it was used for a wide variety of the biomedical applications such as bone implants [Citation106] and artificial organs such as skin [Citation107]. Because PVA has well-known fibre forming the characteristics, many nanofibres of the blends of PVA and chitosan were produced by electrospinning for the tissue engineering and wound healing application [Citation108–110]. Also, nanofibres of PVA and polyhydroxy butyrate were used for the tissue engineering of skin [Citation104].
Polycaprolactone
Polycaprolactone (PCL) was somewhat inexpensive, extremely elastic polyester which revealed a lack of toxicity with the good mechanical properties and a slow degradation time (1–2 years) [Citation111–113]. Before coating electrospun PCL with the soluble collagen, the material was also found to show the suitable cellular interaction [Citation114]. Li et al. proved the potential of PCL as a scaffolding material for the cell-based multiphasic tissue engineering by seeding the human mesenchymal stem cells (hMSCs) on the scaffold prepared from polycaprolactone [Citation115]. Also, Hoda Bahrami et al. have reported the graft of nanofibrous PCL scaffolds loaded with the USSC(unrestricted somatic stem cells) showed the better results during the healing process of the skin defects in rat models [Citation116]. Moreover, PCL/collagen I scaffolds prepared using a layer-by-layer approach provided a favourable environment for the fibroblast survival and proliferation which were useful for skin regeneration because that method allowed to produce a composite scaffold consisting of both dermal and epidermal skin layers [Citation117,Citation118].
Surface modification
Currently, a wide variety of synthetic biodegradable polymers were used as the tissue engineering scaffolding materials. The disadvantage of these scaffolds was their lack of biological recognition on the material surface. Moreover, hydrophobic polymers did not provide the good environment for the cell–material interactions [Citation119]. Consequently, the surface modification of the polymeric scaffolds should have been considered as an active research area [Citation120,Citation121]. Various methods were being utilized to improve the surface such as incubation time, pH value and pre-treatment using aqueous solution [Citation122].
Huizhi Chen et al. fabricated the 3D fibrous scaffolds of PLGA by liquid-collecting electrospinning for chronic wound repair. Then, the surface modification with the native ECM component aims at providing the biological recognition for the cell growth. This work showed the significant progressive proliferation of the human dermal fibroblasts (HDFs) successfully infiltrated into the scaffolds immobilized with the collagen type I [Citation123].
Hybridization of synthetic biodegradable polymers with collagen for skin
The aforementioned two kinds of the biodegradable polymers had their respective advantages and disadvantages. The synthetic biodegradable polymers were easily produced into the desired shapes with somewhat good mechanical strength [Citation88]. Their degradation periods could also be manipulated by controlling the crystallinity, molecular weight and copolymer ratio [Citation82]. Despite these apparent advantages, the scaffolds derived from the synthetic polymers lack cell recognition signals, and their hydrophobic property hinders smooth cell seeding [Citation124]. In contrast, naturally derived collagen had the potential advantages of the specific cell interactions and hydrophilicity; however, the scaffolds which were constructed completely from collagen had poor mechanical strength. Consequently, those two kinds of biodegradable polymers were hybridized to combine the advantageous properties of both constitutes [Citation125–131].
The wettability of a polymer scaffold was deemed essential for the homogeneous and sufficient cell seeding in three dimensions [Citation4,Citation132]. Since biodegradable synthetic polymers were relatively hydrophobic, it is difficult to deliver a cell suspension in a way that evenly distributes the transplanted cells throughout their porous scaffolds [Citation133,Citation134]. Hybridization with collagen could enhance the wettability of the synthetic sponges with water, which facilitates cell seeding [Citation135]. It was reported that the hybrid sponges of the synthetic polymers and collagen possessed almost the same high degree of mechanical strength as those of the synthetic polymers much higher than that of the collagen sponges alone [Citation136].
It was also reported that to prepare the hybrid sponges of synthetic polymers and collagen, the sponges of synthetic polymers were immersed in a collagen solution under a vacuum to fill the sponge pores with a collagen solution. Subsequently, the synthetic polymer sponges containing the collagen solution were frozen and freeze-dried to let the formation of collagen microsponges in the sponge pores [Citation136]. The collagen microsponges can be further cross-linked by treatment with glutaraldehyde [Citation136,Citation137]. Following washing with deionized water and freeze-dried, the hybrid sponges of synthetic polymers and collagen were formed [Citation136] ().
Samples of hybrid scaffolds for skin tissue engineering
This part of review summarizes the various hybrids of the synthetic polymers and biological polymer applications in the skin tissue engineering and wound healing.
PLLA–collagen
Open skin wound needs to be repaired rapidly to prevent the infection [Citation138]. Using artificial skin substitutes for repairing avoids the complications of the grafts, but a perfect material for a scaffold which is strong and allows the regeneration of the skin tissue has to be found [Citation35]. Some existing scaffolds are made from collagen or gelatine, which are appropriate for promoting the tissue regeneration, but they are not mechanically strong [Citation139]. Others, which are made from biodegradable synthetic materials such as poly(l-lactic acid) (PLLA), are stronger, but not so suitable for the tissue growth [Citation140,Citation141]. Guoping Chen et al. at the National Institute for Materials Science, Japan, have made a hybrid scaffold that has all the essential properties. This team had also created “funnel-like” scaffolds with pores which were interconnected under the surface allowing cells to grow into the scaffold. Now, they have formed these funnel-like collagens or gelatine “sponges” on a PLLA mesh to create the hybrid scaffolds [Citation142]. Their results indicated that the funnel-like hybrid scaffolds could be used for the skin tissue regeneration.
Poly(ethylene oxide)–chitosan
Poly(ethylene oxide) (PEO) is also a biocompatible polymer which has been applied as the cartilage tissue repair [Citation143] and wound dressing [Citation144]. Many nanofibres of the blends of PEO and chitosan have also been produced by electrospinning [Citation145–150]. For instance, Klossner et al. [Citation145] made the defect-free nanofibres with the average diameters ranging from 62 ± 9 to 129 ± 16 nm by electrospinning the blended solutions of the chitosan and PEO in acetic acid. Their investigation revealed that as the total polymer concentration (chitosan + PEO) increased, the number of beads declined, and as chitosan concentration increased, fibre diameter reduced [Citation151].
Carboxyethyl chitosan/PVA
Zhou et al. [Citation152] reported the biocompatible carboxyethyl chitosan (CECS)/PVA nanofibres preparation made by electrospinning of aqueous CECS/PVA solution. The fibroblasts (L929) as the reference cell lines were used for analyzing the impact of using the electrospun by using the mentioned materials for the skin regenerations in in vitro environment. Indirect cytotoxicity assessment of the fibre mats revealed that the CECS/PVA electrospun mat was nontoxic to the L929 cell. Cell culture results showed that the fibrous mats were good in helping the cell attachment and proliferation. This new electrospun matrix would be used as the potential wound dressing for the skin regeneration [Citation152].
Chitosan/collagen/PEO
It was reported that the chitosan/collagen/PEO nanofibrous membrane fabricated by electrospinning and then cross-linked by glutaraldehyde vapour had potential as a wound dressing for the skin regeneration [Citation153]. This membrane revealed no cytotoxicity towards the growth of 3T3 fibroblasts and had good in vitro biocompatibility. From animal studies, it has been understood that the membrane was better than gauze and commercial collagen sponge wound dressing in wound healing rate [Citation153].
AgNPs/chitosan/PEO
A wound dressing material composed of silver nanoparticles (AgNPs) and chitosan has been invented by using a nanometer and self-assembly technology [Citation154], and it could significantly improve the rate of wound healing. To develop a better wound dressing, proper uniform AgNPs/chitosan/PEO ultrafine fibres were effectively prepared [Citation155]. Assessing the antimicrobial activities of the electrospun AgNPs/chitosan/PEO fibrous membrane against Escherichia coli revealed that the AgNPs in the ultrafine fibres meaningfully enhanced the inactivation of bacteria. The fibrous membrane was better than other wound dressing containing AgNPs in wound healing rate. Furthermore, the higher antibacterial activity was observed in the electrospun nonwoven mats of AgNPs/PVA/CS blends than in those of PVA/CS blends [Citation156]. The electrospinning meshes are mentioned as the excellent wound dressing.
PCL–collagen
The PCL scaffolds deal with its appropriate biostability and mechanical qualities which ensure its long-term presence and elasticity [Citation157]. One study in particular has reported the use of PCL for the skin grafts as a mix of collagen and PCL in a composite film [Citation158]. The aim was to monitor the degradation of the collagen in a cultured environment and monitored the cell adhesion over some days. The mentioned study followed the behaviour of the various variations of the mixture of PCL and collagen. The resorption rate of the about-one-year determined PCL altered it to the promises for the use for developing the skin grafts for burn victims [Citation158].
Pullulan–collagen
Pullulan–collagen composite hydrogel is another porous scaffold that is capable of the skin regeneration in the cutaneous wounds. Pullulan-based hydrogel is particularly integrated in the dermal wounds [Citation159].
Collagen–elastin
Collagen with the other natural scaffolds can be made from the several origins (traditional and salmon-derived). This scaffolding material was applied for seeding the fibroblast cells and better healing of the skin incisional injuries in animal models [Citation160]. Marine-based collagen–elastin scaffold is a better substitute for the bovine collagen–elastin scaffold as it inhibited the transmission of the prion disease [Citation161].
Nanofibrous scaffold–growth factor
Nanofibrous scaffold may be impregnated with the dual growth factors in the mesh-type structure [Citation162,Citation163]. Applications of the growth factors with the nanofibrous scaffolds are very distinguished through the recent studies [Citation164]. Coating the dermal wounds with this mesh-type scaffolding tissue was associated with the quick releasing of VEGF and slow releasing of PDGF-BB [Citation163]. These growth factors helped the full-thickness wound healing [Citation163].
Smart ultrashort aliphatic peptide hydrogel
Smart ultrashort aliphatic peptide hydrogel with the properties of noncytotoxic and nonimmunogen is another scaffold that has a main tendency to the helical fibre development [Citation165]. The mentioned scaffold promotes the epithelial and dermal regeneration with high closure rate of skin wound and burn repair. This scaffold is used without seeding the growth agents [Citation165].
Natural scaffold and wound infection
Using the natural scaffold for the treatment of wound infection was another wound healing problem. Gelatine/copper faujasite (CAF) composite scaffold was able to improve the wound oxygen supply and accelerate the cellular proliferation [Citation166]. Gelatine is a good natural scaffold for its biodegradability, low immunogenicity and biocompatibility and could be applied for the better viability of NIH3T3 fibroblast cells [Citation167]. In vivo study of gelatine/copper composite revealed the skin regeneration in 20 days after incisional wound creation [Citation166]. Silver ion-releasing poly(l-lactic acid) scaffold showed the wound repair maintaining the excellent viability of the fibroblast and keratinocyte cells. In this scaffold, silver ions were released from a polymeric binder [Citation167]. Another antibacterial scaffold which improved fibronectin and type І collagen expression was the new bilayer 3D activated carbon fibre with the suitable release of the incorporated gentamicin from its poly(γ-glutamic acid)/gelatine membrane [Citation168]. The excellent features of this bilayer novel scaffold were wound healing and antibacterial properties [Citation168].
Acellular porcine or Xe-Derma scaffold
Acellular porcine or Xe-Derma scaffold might be a different scaffold which had an main healing effect on the human skin wound and burn tissue [Citation169,Citation170]. Keratinocytes confluences occurring in 7–10 days and in vitro differentiation of the cultured epidermal cells to the normal epidermal cells were the greatest achieved with the Xe-Derma scaffold coating [Citation170]. Furthermore, porcine acellular dermal matrix (ADM) scaffold was applied with the numerous proportions of the human and rat keratinocytes for the full-thickness skin wound healing in the animal models [Citation171]. These scaffold tissues had frequent healing and regenerative function.
Tissue-engineered skin substitutes and wound dressings for biomedical applications
Two types of tissue-engineered substitutes available are human skin and dermal equivalent (HSE), one is cell-containing matrix which mimics the layer of skin composed of keratinocytes and fibroblast on the collagen matrix and second one is the acellular matrix which contains only the dermal elements with the fibroblast on the collagen matrix [Citation172]. Major mechanism of these substitutes is to stimulate and secrete the wound growth factor by which the epithelialization is achieved. They are able to release the growth factors and cytokines incorporated in dressings because bioengineered is capable of adapting to their environment [Citation173,Citation174]. Bioengineered dressings are appropriate for the venous leg ulcer [Citation175] and diabetic foot ulcer [Citation176]. For example, Apligraf consists of keratinocytes and fibroblast-seeded collagen for the venous ulcers is a FDA-approved skin equivalent substitute [Citation175]. Some skin substitutes commercially available include Integra™ artificial skin composed of collagen/chondroitin 6 sulphate matrix overlaid with a thin silicone sheet [Citation177] and Alloderm™ composed of the normal human fibroblasts with all cellular materials removed [Citation178]. Also, other substitutes are Laserskin™ [Citation179], Bioseed™ [Citation180], Biobrane™ [Citation181] and Hyalograft3-DTM [Citation182].
The other products that have clinical usage and promote wound healing are modern wound dressings. They are usually based on the synthetic polymers and are focused to keep the wound from dehydration and promote healing, so they have been developed to facilitate the function of the wound rather than just to cover it. Based on the type and cause of wound, various products are available in the market, but they are classified as passive, interactive and bioactive products, generally [Citation183–185].
Passive products, such as gauze and tulle dressings, are nonocclusive used to cover the wound to restore its function underneath [Citation186].
Interactive dressings, which are available in the forms of films, foams, hydrogels and hydrocolloids, are semi-occlusive or occlusive. The mentioned dressings act as a barrier against penetration of the bacteria in the wound environment [Citation187]. Semi-permeable film dressings are constructed from the transparent and adherent polyurethane that permits transmission of the water vapour, O2 and CO2 from the wound so that it provides the autolytic debridement of the eschar and impermeable to bacteria [Citation188]. These dressings are recommended for epithelializing wound, superficial wound and shallow wound with the low exudates, and examples of these dressings are Opsite™, Tegaderm™ and Bioclusive™ [Citation189,Citation190]. Semipermeable foam dressings are produced from the hydrophobic and hydrophilic foam with adhesive borders [Citation191], e.g., Lyofoam™, Allevyn™ and Tielle™ [Citation192,Citation193]. Hydrogel dressings are insoluble hydrophilic materials made from the synthetic polymers such as poly(methacrylates) and polyvinylpyrrolidone [Citation194]. These types of dressings are used for the dry chronic wounds, necrotic wounds, pressure ulcers and burn wounds [Citation195]. Some examples of hydrogels are Nu-gel™, Intrasite™, Aquaform™ polymers, sheet dressings, impregnated gauze and water-based gels [Citation196,Citation197]. Hydrocolloid dressings are made from the combination of the gel forming agents such as carboxymethyl cellulose, gelatine and pectin with other materials such as elastomers and adhesives [Citation198]. These dressings are among the most widely used interactive dressings and consist of two layers, inner colloidal layer and outer water-impermeable layer [Citation43]. Granuflex™, Comfeel™ and Tegasorb™ are some hydrocolloid dressings commercially available [Citation199,Citation200]. Alginate dressings are produced from the sodium and calcium salts comprising mannuronic and guluronic acid units [Citation201,Citation202], e.g., Sorbsan™, Kaltostat™ and Algisite™ [Citation200,Citation203].
Bioactive wound dressings, which are known for their biocompatibility, biodegradability and nontoxic nature, are the last type of the modern wound dressings [Citation204] and are derived generally from the natural tissues or artificial sources which play the serious role in healing process such as collagen [Citation205], hyaluronic acid [Citation206], chitosan [Citation207,Citation208], alginate and elastin. These materials are used alone or in combination depending on the nature and type of wound. In order to enhance the wound healing process, the polymers of these materials are sometimes incorporated with the growth factors and antimicrobials, too [Citation43,Citation209].
Conclusions
In sum, the tissue engineering is one of the most exciting interdisciplinary and cross-disciplinary research areas and is growing exponentially through the time. Scaffold materials and fabrication technologies play an essential role in tissue engineering. Currently, the numerous tissue scaffolds have been engineered in the field of wound healing. These nanostructures were vigorously examined in vitro and in vivo studies. The mentioned nanostructures were used for the traumatic wounds in the animal studies and investigations. Using these new scaffold tissues through the animal models was very prominent than in real patients. In this regard, safe applications of the nontoxic and nonmutagenic scaffolds need to be changed for the better compliance with the wound healing in patients. In the traumatic wounds, contractile cells (myofibroblasts) were responsible for a prominent decrease in the wound size. It is necessary to mention that the porous collagen helps skin wound healing and produces new dermal tissue with passing the wound contraction mechanism. The new porous collagen scaffold could be used in the traumatic skin wound for the better regeneration of the dermis; that is, developing tissue engineered the skin substitutes and dressing materials addressing the major interfering factors of the normal healing process will help the patients and wound care practitioners largely.
Acknowledgements
The authors thank the Department of Medical Nanotechnology, Faculty of Advanced Medical Science of Tabriz University for all supports provided. The present work was funded by 2017 Drug Applied Research Center, Tabriz University of Medical Sciences Grant.
Disclosure statement
No potential conflict of interest was reported by the authors.
Additional information
Funding
References
- Jayabalan M. Studies on poly (propylene fumarate-co-caprolactone diol) thermoset composites towards the development of biodegradable bone fixation devices. Int J Biomater. 2009;2009:486710.
- Do Kim H, Bae EH, Kwon IC, et al. Effect of PEG–PLLA diblock copolymer on macroporous PLLA scaffolds by thermally induced phase separation. Biomaterials. 2004;25:2319–2329.
- Khan Y, Yaszemski MJ, Mikos AG, et al. Tissue engineering of bone: material and matrix considerations. J Bone Joint Surg. 2008;90:36–42.
- Dhandayuthapani B, Yoshida Y, Maekawa T, et al. Polymeric scaffolds in tissue engineering application: a review. Int J Polymer Sci. 2011;2011:290602.
- Baldwin SP, Saltzman WM. Materials for protein delivery in tissue engineering. Adv Drug Deliv Rev. 1998;33:71–86.
- Nair LS, Laurencin CT. Biodegradable polymers as biomaterials. Progr Polymer Sci. 2007;32:762–798.
- Sravanthi R. Preparation and characterization of poly (ɛ-caprolactone) PCL scaffolds for tissue engineering applications [Thesis]. Rourkela (India): National Institute of Technology; 2009.
- Weigel T, Schinkel G, Lendlein A. Design and preparation of polymeric scaffolds for tissue engineering. Expert Rev Med Devices. 2006;3:835–851.
- Pilehvar-Soltanahmadi Y, Akbarzadeh A, Moazzez-Lalaklo N, et al. An update on clinical applications of electrospun nanofibers for skin bioengineering. Artif Cells Nanomed Biotechnol. 2016;44:1350–1364.
- Ahmadi-Aghkand F, Gholizadeh-Ghaleh Aziz S, Panahi Y, et al. Recent prospective of nanofiber scaffolds fabrication approaches for skin regeneration. Artif Cells Nanomed Biotechnol. 2016;44:1635–1641.
- Khang G, Lee SJ, Kim MS, Lee HB. Biomaterials: tissue engineering and scaffolds. In: Webster JG, editor. Encyclopedia of Medical Devices and Instrumentation. New York: Wiley; 2006.
- Chaignaud BE, Langer R, Vacanti JP. The history of tissue engineering using synthetic biodegradable polymer scaffolds and cells. In: Atala A, Mooney DJ, editors. Synthetic Biodegradable Polymer Scaffolds. Boston (MA): Springer; 1997. p. 1–14.
- Boyce ST. Cultured skin substitutes: a review. Tissue Eng. 1996;2:255–266.
- Kneser U, Kaufmann PM, Fiegel HC, et al. Long-term differentiated function of heterotopically transplanted hepatocytes on three-dimensional polymer matrices. J Biomed Mater Res. 1999;47:494–503.
- Monsour M, Mohammed R, Gorham S, et al. An assessment of a collagen/vicryl composite membrane to repair defects of the urinary bladder in rabbits. Urol Res. 1987;15:235–238.
- Hadlock T, Sundback C, Hunter D, et al. A polymer foam conduit seeded with Schwann cells promotes guided peripheral nerve regeneration. Tissue Eng. 2000;6:119–127.
- Hansbrough JF, Morgan JL, Greenleaf GE, et al. Composite grafts of human keratinocytes grown on a polyglactin mesh-cultured fibroblast dermal substitute function as a bilayer skin replacement in full-thickness wounds on athymic mice. J Burn Care Res. 1993;14:485–494.
- Ishaug-Riley SL, Crane-Kruger GM, Yaszemski MJ, et al. Three-dimensional culture of rat calvarial osteoblasts in porous biodegradable polymers. Biomaterials. 1998;19:1405–1412.
- Cao Y, Vacanti JP, Paige KT, et al. Transplantation of chondrocytes utilizing a polymer-cell construct to produce tissue-engineered cartilage in the shape of a human ear. Plast Reconstr Surg. 1997;100:297–302.
- Lin VS, Lee MC, O'Neal S, et al. Ligament tissue engineering using synthetic biodegradable fiber scaffolds. Tissue Eng. 1999;5:443–451.
- Massia SP, Holecko MM, Ehteshami GR. In vitro assessment of bioactive coatings for neural implant applications. J Biomed Mater Res A. 2004;68:177–186.
- Broderick E, Infanger S, Turner T, et al. Depressed bone mineralization following high dose TGF-β1 application in an orthopedic implant model. Calcif Tissue Int. 2005;76:379–384.
- McKinney L, Hollinger JO. A bone regeneration study: transforming growth factor-[beta] 1 and its delivery. J Craniofacial Surg. 1996;7:36–45.
- Critchlow M, Bland Y, Ashhurst D. The effect of exogenous transforming growth factor-β2 on healing fractures in the rabbit. Bone. 1995;16:521–527.
- Schmidmaier G, Lucke M, Schwabe P, et al. Collective review: bioactive implants coated with poly (D,L-lactide) and growth factors IGF-I, TGF-β1, or BMP-2 for stimulation of fracture healing. J Long Term Eff Med Implants. 2006;16:61–69.
- Street J, Bao M, Bunting S, et al. Vascular endothelial growth factor stimulates bone repair by promoting angiogenesis and bone turnover. Proc Natl Acad Sci. 2002;99:9656–9661.
- Becker C, Olde DL, Laeufer T, et al. 'UroMaix' scaffolds: novel collagen matrices for application in tissue engineering of the urinary tract. Int J Artif Organs. 2006;29:764–771.
- Urist MR. Bone: formation by autoinduction. Science. 1965;150:893–899.
- Wozney JM, Rosen V, Celeste AJ, et al. Novel regulators of bone formation: molecular clones and activities. Obstet Gynecol Survey. 1989;44:387.
- Willerth SM, Sakiyama-Elbert SE. Approaches to neural tissue engineering using scaffolds for drug delivery. Adv Drug Deliv Rev. 2007;59:325–338.
- Huang S, Deng T, Wang Y, et al. Multifunctional implantable particles for skin tissue regeneration: preparation, characterization, in vitro and in vivo studies. Acta Biomaterialia. 2008;4:1057–1066.
- Danielsson C, Ruault S, Basset-Dardare A, et al. Modified collagen fleece, a scaffold for transplantation of human bladder smooth muscle cells. Biomaterials. 2006;27:1054–1060.
- Chin CD, Khanna K, Sia SK. A microfabricated porous collagen-based scaffold as prototype for skin substitutes. Biomed Microdevices. 2008;10:459–467.
- Shields KJ, Beckman MJ, Bowlin GL, et al. Mechanical properties and cellular proliferation of electrospun collagen type II. Tissue Eng. 2004;10:1510–1517.
- Burke JF, Yannas IV, Quinby WC Jr, et al. Successful use of a physiologically acceptable artificial skin in the treatment of extensive burn injury. Ann Surg. 1981;194:413.
- Fitton AR, Drew P, Dickson WA. The use of a bilaminate artificial skin substitute (IntegraTM) in acute resurfacing of burns: an early experience. Br J Plastic Surg. 2001;54:208–212.
- Heimbach DM, Warden GD, Luterman A, et al. Multicenter postapproval clinical trial of Integra® dermal regeneration template for burn treatment. J Burn Care Res. 2003;24:42–48.
- Aper T, Schmidt A, Duchrow M, et al. Autologous blood vessels engineered from peripheral blood sample. Eur J Vasc Endovasc Surg. 2007;33:33–39.
- Tamada Y. New process to form a silk fibroin porous 3-D structure. Biomacromolecules. 2005;6:3100–3106.
- Herbert CB, Bittner GD, Hubbell JA. Effects of fibrinolysis on neurite growth from dorsal root ganglia cultured in two- and three-dimensional fibrin gels. J Comp Neurol. 1996;365:380–391.
- Sakiyama SE, Schense JC, Hubbell JA. Incorporation of heparin-binding peptides into fibrin gels enhances neurite extension: an example of designer matrices in tissue engineering. FASEB J. 1999;13:2214–2224.
- Ahmed TA, Dare EV, Hincke M. Fibrin: a versatile scaffold for tissue engineering applications. Tissue Eng Part B Rev. 2008;14:199–215.
- Boateng JS, Matthews KH, Stevens HN, et al. Wound healing dressings and drug delivery systems: a review. J Pharm Sci. 2008;97:2892–2923.
- Sofia S, McCarthy MB, Gronowicz G, et al. Functionalized silk-based biomaterials for bone formation. J Biomed Mater Res. 2001;54:139–148.
- Malafaya PB, Silva GA, Reis RL. Natural–origin polymers as carriers and scaffolds for biomolecules and cell delivery in tissue engineering applications. Adv Drug Deliv Rev. 2007;59:207–233.
- Li M, Mondrinos MJ, Gandhi MR, et al. Electrospun protein fibers as matrices for tissue engineering. Biomaterials. 2005;26:5999–6008.
- Daamen WF, Veerkamp J, Van Hest J, et al. Elastin as a biomaterial for tissue engineering. Biomaterials. 2007;28:4378–4398.
- Sell S, McClure MJ, Barnes CP, et al. Electrospun polydioxanone–elastin blends: potential for bioresorbable vascular grafts disclosure. Several authors have United States and International patents pending concerning technology presented in this article, and this technology has been licensed to NanoMatrix, Inc., in which several authors have a financial interest. Biomed Mater. 2006;1:72.
- Rnjak-Kovacina J, Wise SG, Li Z, et al. Electrospun synthetic human elastin: collagen composite scaffolds for dermal tissue engineering. Acta Biomaterialia. 2012;8:3714–3722.
- Kumar MR, Muzzarelli RA, Muzzarelli C, et al. Chitosan chemistry and pharmaceutical perspectives. Chem Rev. 2004;104:6017–6084.
- Muzzarelli R. Human enzymatic activities related to the therapeutic administration of chitin derivatives. Cell Mol Life Sci. 1997;53:131–140.
- Okamoto Y, Yano R, Miyatake K, et al. Effects of chitin and chitosan on blood coagulation. Carbohyd Polymers. 2003;53:337–342.
- Kweon D-K, Song S-B, Park Y-Y. Preparation of water-soluble chitosan/heparin complex and its application as wound healing accelerator. Biomaterials. 2003;24:1595–1601.
- Ueno H, Mori T, Fujinaga T. Topical formulations and wound healing applications of chitosan. Adv Drug Deliv Rev. 2001;52:105–115.
- Alemdaroğlu C, Değim Z, Çelebi N, et al. An investigation on burn wound healing in rats with chitosan gel formulation containing epidermal growth factor. Burns. 2006;32:319–327.
- Mei L, Hu D, Ma J, et al. Preparation, characterization and evaluation of chitosan macroporous for potential application in skin tissue engineering. Int J Biol Macromol. 2012;51:992–997.
- Choi JS, Yoo HS. Pluronic/chitosan hydrogels containing epidermal growth factor with wound-adhesive and photo-crosslinkable properties. J Biomed Mater Res A. 2010;95:564–573.
- Chen Y-H, Wang I-J, Young T-H. Formation of keratocyte spheroids on chitosan-coated surface can maintain keratocyte phenotypes. Tissue Eng Part A. 2009;15:2001–2013.
- Bragulla HH, Homberger DG. Structure and functions of keratin proteins in simple, stratified, keratinized and cornified epithelia. J Anat. 2009;214:516–559.
- Tachibana A, Furuta Y, Takeshima H, et al. Fabrication of wool keratin sponge scaffolds for long-term cell cultivation. J Biotechnol. 2002;93:165–170.
- Khalilov RI, Ahmadov IS, Kadirov SG. Two types of kinetics of membrane potential of water plant leaves illuminated by ultraviolet light. Bioelectrochemistry. 2002;58:189–191.
- Bhardwaj N, Sow WT, Devi D, et al. Silk fibroin–keratin based 3D scaffolds as a dermal substitute for skin tissue engineering. Integr Biol. 2015;7:53–63.
- Christensen B. Alginates as biomaterials in tissue engineering. In: Rauter AP, Lindhorst TK, editors. Carbohydrate chemistry: chemical and biological approaches. Vol. 37. Cambridge (UK): RSC Publishing; 2011. p. 227–258.
- Xu H, Ma L, Shi H, et al. Chitosan–hyaluronic acid hybrid film as a novel wound dressing: in vitro and in vivo studies. Polym Adv Technol. 2007;18:869–875.
- Wounds U. Best practice statement: the use of topical antiseptic/antimicrobial agents in wound management. London (UK): Wounds; 2010.
- Li X, Chen S, Zhang B, et al. In situ injectable nano-composite hydrogel composed of curcumin, N, O-carboxymethyl chitosan and oxidized alginate for wound healing application. Int J Pharm. 2012;437:(1):110–119.
- Hooper SJ, Percival SL, Hill KE, et al. The visualisation and speed of kill of wound isolates on a silver alginate dressing. Int Wound J. 2012;9:633–642.
- Barnett S, Varley S. The effects of calcium alginate on wound healing. Ann R Coll Surg Engl. 1987;69:153.
- Balakrishnan B, Mohanty M, Umashankar P, et al. Evaluation of an in situ forming hydrogel wound dressing based on oxidized alginate and gelatin. Biomaterials. 2005;26:6335–6342.
- Sun J, Tan H. Alginate-based biomaterials for regenerative medicine applications. Materials. 2013;6:1285–1309.
- Straccia MC, d'Ayala GG, Romano I, et al. Alginate hydrogels coated with chitosan for wound dressing. Mar Drugs. 2015;13:2890–2908.
- Khan F, Ahmad SR. Polysaccharides and their derivatives for versatile tissue engineering application. Macromol Biosci. 2013;13:395–421.
- Venkatesan J, Pallela R, Kim S-K. Dispersion of single walled carbon nanotubes in marine polysaccharides for bone tissue engineering. J Biomater Tissue Eng. 2014;4:501–505.
- Yuvarani I, Kumar SS, Venkatesan J, et al. Preparation and characterization of curcumin coated chitosan-alginate blend for wound dressing application. J Biomat Tissue Eng. 2012;2:54–60.
- Rath G, Johal E, Goyal AK. Development of serratiopeptidase and metronidazole based alginate microspheres for wound healing. Artif Cells Blood Substit Immobil Biotechnol. 2011;39:44–50.
- Chandika P, Ko S-C, Oh G-W, et al. Fish collagen/alginate/chitooligosaccharides integrated scaffold for skin tissue regeneration application. Int J Biol Macromol. 2015;81:504–513.
- Venkatesan J, Jayakumar R, Anil S, et al. Development of alginate-chitosan-collagen based hydrogels for tissue engineering. J Biomater Tissue Eng. 2015;5:458–464.
- Arthanari S, Mani G, Jang JH, et al. Preparation and characterization of gatifloxacin-loaded alginate/poly (vinyl alcohol) electrospun nanofibers. Artif Cells Nanomed Biotechnol. 2016;44:847–852.
- Chen WJ, Abatangelo G. Functions of hyaluronan in wound repair. Wound Repair Regen. 1999;7:79–89.
- Gravante G, Delogu D, Giordan N, et al. The use of hyalomatrix PA in the treatment of deep partial-thickness burns. J Burn Care Res. 2007;28:269–274.
- Madhyastha H, Madhyastha R, Nakajima Y, et al. Regulation of growth factors‐associated cell migration by C‐phycocyanin scaffold in dermal wound healing. Clin Exp Pharmacol Physiol. 2012;39:13–19.
- Gunatillake P, Mayadunne R, Adhikari R. Recent developments in biodegradable synthetic polymers. Biotechnol Annu Rev. 2006;12:301–347.
- Moran JM, Pazzano D, Bonassar LJ. Characterization of polylactic acid–polyglycolic acid composites for cartilage tissue engineering. Tissue Eng. 2003;9:63–70.
- Singhal A, Agrawal C, Athanasiou KA. Salient degradation features of a 50:50 PLA/PGA scaffold for tissue engineering. Tissue Eng. 1996;2:197–207.
- Khalilov RI, Khomutov GB, Tikhonov AN. Effect of ultraviolet radiation on structural-functional characteristics of the thylakoid membrane. Russ Plant Physiol. 1993;3:338–342.
- Zhang J, Wu L, Jing D, et al. A comparative study of porous scaffolds with cubic and spherical macropores. Polymer. 2005;46:4979–4985.
- Gupta V, Sengupta M, Prakash J, Tripathy BC. Tissue engineering and artificial organ. In: Basic and applied aspects of biotechnology. Singapore: Springer; 2017. p. 453–74.
- Chen G, Ushida T, Tateishi T. Scaffold design for tissue engineering. Macromol Biosci. 2002;2:67–77.
- Cheng Y-L, Lee M-L. Development of dynamic masking rapid prototyping system for application in tissue engineering. Rapid Prototyp J. 2009;15:29–41.
- Williams D, Mort E. Enzyme-accelerated hydrolysis of polyglycolic acid. J Bioeng. 1977;1:231–238.
- Li X, Yang Y, Fan Y, et al. Biocomposites reinforced by fibers or tubes as scaffolds for tissue engineering or regenerative medicine. J Biomed Mater Res A. 2014;102:1580–1594.
- Slivka MA, Leatherbury NC, Kieswetter K, et al. Porous, resorbable, fiber-reinforced scaffolds tailored for articular cartilage repair. Tissue Eng. 2001;7:767–780.
- Singh D, Singh MR. Development of antibiotic and debriding enzyme-loaded PLGA microspheres entrapped in PVA-gelatin hydrogel for complete wound management. Artif Cells Blood Subst Biotechnol. 2012;40:345–353.
- Amirthalingam M, Kasinathan N, Amuthan A, et al. Bioactive PLGA–curcumin microparticle-embedded chitosan scaffold: in vitro and in vivo evaluation. Artif Cells Blood Subst Biotechnol. 2017;45:233–241.
- Bryant SJ, Anseth KS. Hydrogel properties influence ECM production by chondrocytes photoencapsulated in poly (ethylene glycol) hydrogels. J Biomed Mater Res. 2002;59:63–72.
- Lee S-H, Moon JJ, Miller JS, et al. Poly (ethylene glycol) hydrogels conjugated with a collagenase-sensitive fluorogenic substrate to visualize collagenase activity during three-dimensional cell migration. Biomaterials. 2007;28:3163–3170.
- Mann BK, Gobin AS, Tsai AT, et al. Smooth muscle cell growth in photopolymerized hydrogels with cell adhesive and proteolytically degradable domains: synthetic ECM analogs for tissue engineering. Biomaterials. 2001;22:3045–3051.
- Veronese FM, Pasut G. PEGylation, successful approach to drug delivery. Drug Discov Today. 2005;10:1451–1458.
- Vahidi M, Frounchi M, Dadbin S. Porous gelatin/poly (ethylene glycol) scaffolds for skin cells. Soft Mater. 2016;15:95–102.
- Müller HM, Seebach D. Poly (hydroxyalkanoates): a fifth class of physiologically important organic biopolymers? Angew Chem Int Ed Engl. 1993;32:477–502.
- Philip S, Keshavarz T, Roy I. Polyhydroxyalkanoates: biodegradable polymers with a range of applications. J Chem Technol Biotechnol. 2007;82:233–247.
- Chen G-Q, Wu Q. The application of polyhydroxyalkanoates as tissue engineering materials. Biomaterials. 2005;26:6565–6578.
- Ljungberg C, Johansson‐Ruden G, Junemo Boström K, et al. Neuronal survival using a resorbable synthetic conduit as an alternative to primary nerve repair. Microsurgery. 1999;19:259–264.
- Asran AS, Razghandi K, Aggarwal N, et al. Nanofibers from blends of polyvinyl alcohol and polyhydroxy butyrate as potential scaffold material for tissue engineering of skin. Biomacromolecules. 2010;11:3413–3421.
- Keshel SH, Biazar E, Rezaei Tavirani M, et al. The healing effect of unrestricted somatic stem cells loaded in collagen-modified nanofibrous PHBV scaffold on full-thickness skin defects. Artif Cells Nanomed Biotechnol. 2014;42:210–216.
- Allen MJ, Schoonmaker JE, Bauer TW, et al. Preclinical evaluation of a poly (vinyl alcohol) hydrogel implant as a replacement for the nucleus pulposus. Spine. 2004;29:515–523.
- Chen DH, Leu JC, Huang TC. Transport and hydrolysis of urea in a reactor–separator combining an anion‐exchange membrane and immobilized urease. J Chem Technol Biotechnol. 1994;61:351–357.
- Zhou Y, Yang D, Nie J. Electrospinning of chitosan/poly (vinyl alcohol)/acrylic acid aqueous solutions. J Appl Polym Sci. 2006;102:5692–5697.
- Zheng H, Du Y, Yu J, et al. Preparation and characterization of chitosan/poly (vinyl alcohol) blend fibers. J Appl Polym Sci. 2001;80:2558–2565.
- Zhang Y, Huang X, Duan B, et al. Preparation of electrospun chitosan/poly (vinyl alcohol) membranes. Colloid Polym Sci. 2007;285:855–863.
- Youssef NA, Gurbanov EM, Haciyeva SR, et al. Antioxidant enzymes, fluctuating asymmetry and morphological changes of urban trees as an ecological indicator of heavy metal stress. Int J Pharm Sci Health Care. 2013;1:1–18.
- Zeng J, Chen X, Liang Q, et al. Enzymatic degradation of poly (L‐lactide) and poly (ɛ‐caprolactone) electrospun fibers. Macromol Biosci. 2004;4:1118–1125.
- Valizadeh A, Bakhtiary M, Akbarzadeh A, et al. Preparation and characterization of novel electrospun poly (ε-caprolactone)-based nanofibrous scaffolds. Artif Cells Nanomed Biotechnol. 2016;44:504–509.
- Yoshimoto H, Shin Y, Terai H, et al. A biodegradable nanofiber scaffold by electrospinning and its potential for bone tissue engineering. Biomaterials. 2003;24:2077–2082.
- Li W-J, Tuli R, Huang X, et al. Multilineage differentiation of human mesenchymal stem cells in a three-dimensional nanofibrous scaffold. Biomaterials. 2005;26:5158–5166.
- Bahrami H, Keshel SH, Chari AJ, et al. Human unrestricted somatic stem cells loaded in nanofibrous PCL scaffold and their healing effect on skin defects. Artif Cells Nanomed Biotechnol. 2016;44:1556–1560.
- Bonvallet PP, Culpepper BK, Bain JL, et al. Microporous dermal-like electrospun scaffolds promote accelerated skin regeneration. Tissue Eng Part A. 2014;20:2434–2445.
- Bonvallet PP, Schultz MJ, Mitchell EH, et al. Microporous dermal-mimetic electrospun scaffolds pre-seeded with fibroblasts promote tissue regeneration in full-thickness skin wounds. PLoS One. 2015;10:e0122359.
- Mikos AG, Lyman MD, Freed LE, et al. Wetting of poly(L-lactic acid) and poly(DL-lactic-co-glycolic acid) foams for tissue culture. Biomaterials. 1994;15:55–58.
- Ebrahimi E, Akbarzadeh A, Abbasi E, et al. Novel drug delivery system based on doxorubicin-encapsulated magnetic nanoparticles modified with PLGA-PEG1000 copolymer. Artif Cells Nanomed Biotechnol. 2016;44:290–297.
- Yamamoto M, Kato K, Ikada Y. Ultrastructure of the interface between cultured osteoblasts and surface-modified polymer substrates. J Biomed Mater Res. 1997;37:29–36.
- Zhang R, Ma PX. Porous poly (L-lactic acid)/apatite composites created by biomimetic process. J Biomed Mater Res. 1999;45:285–293.
- Chen H, Peng Y, Wu S, et al. Electrospun 3D fibrous scaffolds for chronic wound repair. Materials. 2016;9:272.
- Place ES, George JH, Williams CK, et al. Synthetic polymer scaffolds for tissue engineering. Chem Soc Rev. 2009;38:1139–1151.
- Kiyotani T, Teramachi M, Takimoto Y, et al. Nerve regeneration across a 25-mm gap bridged by a polyglycolic acid-collagen tube: a histological and electrophysiological evaluation of regenerated nerves. Brain Res. 1996;740:66–74.
- Miki H, Ando N, Ozawa S, et al. An artificial esophagus constructed of cultured human esophageal epithelial cells, fibroblasts, polyglycolic acid mesh, and collagen. ASAIO J. 1999;45:502–508.
- Saxena AK, Marler J, Benvenuto M, et al. Skeletal muscle tissue engineering using isolated myoblasts on synthetic biodegradable polymers: preliminary studies. Tissue Eng. 1999;5:525–531.
- Chen G, Ushida T, Tateishi T. Poly(DL-lactic-co-glycolic acid) sponge hybridized with collagen microsponges and deposited apatite particulates. J Biomed Mater Res. 2001;57:8–14.
- Khalilov RI, Nasibova AN, Serezhenkov VA, et al. Accumulation of magnetic nanoparticles in plants grown on soils of Apsheron peninsula. Biophysics. 2011;56:316–322.
- Chen G, Ushida T, Tateishi T. Hybrid biomaterials for tissue engineering: a preparative method for PLA or PLGA–collagen hybrid sponges. Adv Mater. 2000;12:455–457.
- Chen G, Sato T, Ushida T, et al. The use of a novel PLGA fiber/collagen composite web as a scaffold for engineering of articular cartilage tissue with adjustable thickness. J Biomed Mater Res. 2003;67:1170–1180.
- Colter K, Shen M, Bell A. Reduction of progesterone release rate through silicone membranes by plasma polymerization. Biomater Med Devices Artif Organs. 1977;5:13–24.
- Sodhi RN. Application of surface analytical and modification techniques to biomaterial research. J Electron Spectrosc Relat Phenomena. 1996;81:269–284.
- Brewis D, Briggs D. Adhesion to polyethylene and polypropylene. Polymer. 1981;22:7–16.
- Reis RL, Neves NM, Mano JF, Gomes ME, Marques AP, Azevedo HS. Natural-based polymers for biomedical applications. Amsterdam (The Netherlands): Elsevier; 2008.
- Chen G, Ushida T, Tateishi T. A hybrid network of synthetic polymer mesh and collagen sponge. Chem Commun. 2000;1505–1506.
- Bellincampi LD, Dunn MG. Effect of crosslinking method on collagen fiber-fibroblast interactions. J Appl Polym Sci. 1997;63:1493–1498.
- Yannas I, Burke JF. Design of an artificial skin. I. Basic design principles. J Biomed Mater Res. 1980;14:65–81.
- Trasciatti S, Podestà A, Bonaretti S, et al. In vitro effects of different formulations of bovine collagen on cultured human skin. Biomaterials. 1998;19:897–903.
- Cooper ML, Hansbrough JF, Spielvogel RL, et al. In vivo optimization of a living dermal substitute employing cultured human fibroblasts on a biodegradable polyglycolic acid or polyglactin mesh. Biomaterials. 1991;12:243–248.
- Yang W-S, Roh H-W, Lee WK, et al. Evaluation of functions and tissue compatibility of poly (D, L-lactic-co-glycolic acid) seeded with human dermal fibroblasts. J Biomater Sci Polymer Ed. 2006;17:151–162.
- Lu H, Oh HH, Kawazoe N, et al. PLLA–collagen and PLLA–gelatin hybrid scaffolds with funnel-like porous structure for skin tissue engineering. Sci Technol Adv Mater. 2012;13:064210.
- Sims DC, Butler PE, Casanova R, et al. Injectable cartilage using polyethylene oxide polymer substrates. Plastic Reconstruct Surg. 1996;98:843–850.
- Gorjikhah F, Azizi Jalalian F, Salehi R, et al. Preparation and characterization of PLGA-β-CD polymeric nanoparticles containing methotrexate and evaluation of their effects on T47D cell line. Artif Cells Nanomed Biotechnol. 2017;45:432–440.
- Klossner RR, Queen HA, Coughlin AJ, et al. Correlation of chitosan’s rheological properties and its ability to electrospin. Biomacromolecules. 2008;9:2947–2953.
- Bhattarai N, Edmondson D, Veiseh O, et al. Electrospun chitosan-based nanofibers and their cellular compatibility. Biomaterials. 2005;26:6176–6184.
- Kriegel C, Kit K, McClements DJ, et al. Electrospinning of chitosan–poly (ethylene oxide) blend nanofibers in the presence of micellar surfactant solutions. Polymer. 2009;50:189–200.
- Kriegel C, Kit K, McClements DJ, et al. Influence of surfactant type and concentration on electrospinning of chitosan–poly (ethylene oxide) blend nanofibers. Food Biophys. 2009;4:213–228.
- Zhang J-F, Yang D-Z, Xu F, et al. Electrospun core: shell structure nanofibers from homogeneous solution of poly (ethylene oxide)/chitosan. Macromolecules. 2009;42:5278–5284.
- Duan B, Dong C, Yuan X, et al. Electrospinning of chitosan solutions in acetic acid with poly(ethylene oxide). J Biomater Sci Polym Ed. 2004;15:797–811.
- Sun K, Li Z. Preparations, properties and applications of chitosan based nanofibers fabricated by electrospinning. Express Polym Lett. 2011;5:342–361.
- Zhou Y, Yang D, Chen X, et al. Electrospun water-soluble carboxyethyl chitosan/poly (vinyl alcohol) nanofibrous membrane as potential wound dressing for skin regeneration. Biomacromolecules. 2007;9:349–354.
- Chen J-P, Chang G-Y, Chen J-K. Electrospun collagen/chitosan nanofibrous membrane as wound dressing. Colloids Surfaces A. 2008;313:183–188.
- Lu S, Gao W, Gu HY. Construction, application and biosafety of silver nanocrystalline chitosan wound dressing. Burns. 2008;34:623–628.
- An J, Zhang H, Zhang J, et al. Preparation and antibacterial activity of electrospun chitosan/poly (ethylene oxide) membranes containing silver nanoparticles. Colloid Polym Sci. 2009;287:1425–1434.
- Hang AT, Tae B, Park JS. Non-woven mats of poly (vinyl alcohol)/chitosan blends containing silver nanoparticles: fabrication and characterization. Carbohyd Polym. 2010;82:472–479.
- Yoon CS, Ji DS. Effects of In Vitro degradation on the weight loss and tensile properties of PLA/LPCL/HPCL blend fibers. Fibers Polym. 2005;6:13–18.
- Dai N-T, Williamson M, Khammo N, et al. Composite cell support membranes based on collagen and polycaprolactone for tissue engineering of skin. Biomaterials. 2004;25:4263–4271.
- Wong VW, Rustad KC, Galvez MG, et al. Engineered pullulan–collagen composite dermal hydrogels improve early cutaneous wound healing. Tissue Eng Part A. 2010;17:631–644.
- Rnjak J, Wise SG, Mithieux SM, et al. Severe burn injuries and the role of elastin in the design of dermal substitutes. Tissue Eng Part B Rev. 2011;17:81–91.
- Aberoumandi SM, Mohammadhosseini M, Abasi E, et al. An update on applications of nanostructured drug delivery systems in cancer therapy. Artif Cells Nanomed Biotechnol. 2017;45:1058–1068.
- Gupta KC, Haider A, Choi Y-r, et al. Nanofibrous scaffolds in biomedical applications. Biomater Res. 2014;18:5.
- Xie Z, Paras CB, Weng H, et al. Dual growth factor releasing multi-functional nanofibers for wound healing. Acta Biomaterialia. 2013;9:9351–9359.
- Beachley V, Wen X. Polymer nanofibrous structures: Fabrication, biofunctionalization, and cell interactions. Progr Polym Sci. 2010;35:868–892.
- Loo Y, Wong Y-C, Cai EZ, et al. Ultrashort peptide nanofibrous hydrogels for the acceleration of healing of burn wounds. Biomaterials. 2014;35:4805–4814.
- Ninan N, Muthiah M, Yahaya NAB, et al. Antibacterial and wound healing analysis of gelatin/zeolite scaffolds. Colloids Surf B Biointerfaces. 2014;115:244–252.
- Dreesmann L, Ahlers M, Schlosshauer B. The pro-angiogenic characteristics of a cross-linked gelatin matrix. Biomaterials. 2007;28:5536–5543.
- Huang W-Y, Yeh C-L, Lin J-H, et al. Development of fibroblast culture in three-dimensional activated carbon fiber-based scaffold for wound healing. J Mater Sci. 2012;23:1465–1478.
- Matoušková E, Mestak O. The effect of different biologic and biosynthetic wound covers on keratinocyte growth, stratification and differentiation in vitro. J Tissue Eng. 2014;5:2041731414554966.
- Zajicek R, Mandys V, Mestak O, et al. Human keratinocyte growth and differentiation on acellular porcine dermal matrix in relation to wound healing potential. Sci World J. 2012;2012:727352.
- Shichu X, Shihui Z, Hengyu L, et al. Feasibility study of composite skin reconstructed by mixing keratinocytes and acellular dermal matrix for wound repair. Swiss Med Week. 2009;139:16.
- Auger FA, Valle CAL, Guignard R, et al. Skin equivalent produced with human collagen. In Vitro Cell Dev Biol Anim. 1995;31:432–439.
- El Ghalbzouri A, Hensbergen P, Gibbs S, et al. Fibroblasts facilitate re-epithelialization in wounded human skin equivalents. Lab Invest. 2004;84:102–112.
- Singh MR, Saraf S, Vyas A, et al. Innovative approaches in wound healing: trajectory and advances. Artif Cells Nanomed Biotechnol. 2013;41:202–212.
- Falanga V, Margolis D, Alvarez O, et al. Rapid healing of venous ulcers and lack of clinical rejection with an allogeneic cultured human skin equivalent. Human Skin Equivalent Investigators Group. Arch Dermatol. 1998;134:293–300.
- Brem H, Young J, Tomic-Canic M, et al. Clinical efficacy and mechanism of bilayered living human skin equivalent (HSE) in treatment of diabetic foot ulcers. Surg Technol Int. 2001;11:23–31.
- Kremer M, Lang E, Berger A. Evaluation of dermal—epidermal skin equivalents (‘composite-skin’) of human keratinocytes in a collagen-glycosaminoglycan matrix (integra™ artificial skin). Br J Plastic Surg. 2000;53:459–465.
- Supp DM, Boyce ST. Engineered skin substitutes: practices and potentials. Clin Dermatol. 2005;23:403–412.
- Lam PK, Chan ES, To EW, et al. Development and evaluation of a new composite Laserskin graft. J Trauma. 1999;47:918.
- Vanscheidt W, Ukat A, Horak V, et al. Treatment of recalcitrant venous leg ulcers with autologous keratinocytes in fibrin sealant: a multinational randomized controlled clinical trial. Wound Repair Regen. 2007;15:308–315.
- Hansbrough JF, Morgan J, Greenleaf G, et al. Development of a temporary living skin replacement composed of human neonatal fibroblasts cultured in Biobrane, a synthetic dressing material. Surgery. 1994;115:633–644.
- Dhivya S, Padma VV, Santhini E. Wound dressings: a review. BioMedicine (Taipei). 2015;5:22.
- Strecker-McGraw MK, Jones TR, Baer DG. Soft tissue wounds and principles of healing. Emergency Med Clin N Am. 2007;25:1–22.
- Rivera AE, Spencer JM. Clinical aspects of full-thickness wound healing. Clin Dermatol. 2007;25:39–48.
- Hunt TK, Hopf H, Hussain Z. Physiology of wound healing. Adv Skin Wound Care. 2000;13:6.
- Stashak TS, Farstvedt E, Othic A. Update on wound dressings: indications and best use. Clin Techn Equine Prac. 2004;3:148–163.
- Morin RJ, Tomaselli NL. Interactive dressings and topical agents. Clin Plast Surg. 2007;34:643–658.
- Moshakis V, Fordyce M, Griffiths J, et al. Tegadern versus gauze dressing in breast surgery. Br J Clin Pract. 1984;38:149
- Buchan I, Andrews J, Lang S, et al. Clinical and laboratory investigation of the composition and properties of human skin wound exudate under semi-permeable dressings. Burns. 1981;7:326–334.
- Rogozinski WJ. Modifiable, semi-permeable, wound dressing. Google Patents; 1993.
- Morgan D. Wounds-what should a dressings formulary include? Pharm J. 2009;9:261.
- Jones V, Grey JE, Harding KG. Wound dressings. BMJ. 2006;332:777.
- Ashford R, Freear N, Shippen J. An in-vitro study of the pressure-relieving properties of four wound dressings for foot ulcers. J Wound Care. 2001;10:34–38.
- Razzak MT, Darwis D. Irradiation of polyvinyl alcohol and polyvinyl pyrrolidone blended hydrogel for wound dressing. Radiat Phys Chem. 2001;62:107–113.
- Thomas DR, Goode PS, LaMaster K, et al. Acemannan hydrogel dressing versus saline dressing for pressure ulcers: a randomized, controlled trial. Adv Skin Wound Care. 1998;11:273–276.
- Boateng J, Catanzano O. Advanced therapeutic dressings for effective wound healing—a review. J Pharm Sci. 2015;104:3653–3680.
- Advanced wound dressing. (Srinagarind Med J). 2013;28:18–23.
- Pudner R. Hydrocolloid dressings. Pract Nurs. 1998;9:17–19.
- Cullum N. Leg ulcer treatments: a critical review (part 2). Nurs Std. 1994;9:32–36.
- Skórkowska-Telichowska K, Czemplik M, Kulma A, et al. The local treatment and available dressings designed for chronic wounds. J Am Acad Dermatol. 2013;68:e117–e126.
- Suzuki Y, Tanihara M, Nishimura Y, et al. In vivo evaluation of a novel alginate dressing. J Biomed Mater Res. 1999;48:522–527.
- Suzuki Y, Nishimura Y, Tanihara M, et al. Evaluation of a novel alginate gel dressing: Cytotoxicity to fibroblasts in vitro and foreign‐body reaction in pig skin in vivo. J Biomed Mater Res. 1998;39:317–322.
- Pendry E. The use of alginate dressings in the treatment of diabetic foot ulcers. Diabetic Foot. 2006;9:76–83.
- Dias A, Braga M, Seabra I, et al. Development of natural-based wound dressings impregnated with bioactive compounds and using supercritical carbon dioxide. Int J Pharm. 2011;408:9–19.
- Ramshaw JA, Werkmeister JA, Glattauer V. Collagen-based biomaterials. Biotechnol Genet Eng Rev. 1996;13:335–382.
- Doillon CJ, Silver FH. Collagen-based wound dressing: Effects of hyaluronic acid and firponectin on wound healing. Biomaterials. 1986;7:3–8.
- Ishihara M, Nakanishi K, Ono K, et al. Photocrosslinkable chitosan as a dressing for wound occlusion and accelerator in healing process. Biomaterials. 2002;23:833–840.
- Karahaliloglu Z, Kilicay E, Denkbas EB. Antibacterial chitosan/silk sericin 3D porous scaffolds as a wound dressing material. Artif Cells Nanomed Biotechnol. 2016;45:1–14.
- Wiegand C, Heinze T, Hipler UC. Comparative in vitro study on cytotoxicity, antimicrobial activity, and binding capacity for pathophysiological factors in chronic wounds of alginate and silver‐containing alginate. Wound Repair Regener. 2009;17:511–521.