Abstract
Haemoglobin-based oxygen carrier (HBOC) is highly susceptible to autoxidation that renders a series of tissue and cellular toxicities. HBOC is prepared by chemical modification of haemoglobin (Hb), which typically increases the autoxidation rate of Hb. Thus, it is necessary to decrease the autoxidation of HBOC. In the present study, two dextran-bHb conjugates (dex20-bHb and dex40-bHb) were prepared by conjugation with 20 kDa or 40 kDa dextrans, where the thiol group of Cys-93(β) was reversibly protected. The autoxidation rate of bHb was decreased by conjugation with 20 kDa dextran and maintained by conjugation with 40 kDa dextran. In order to understand the low autoxidation rate of dex20-bHb, the effects of aldehyde modification and dextran on the autoxidation rate of dex20-bHb were investigated. The high oxygen affinity, high tetramer stability and dextran itself were found to decrease the autoxidation rate of dex20-bHb. The conjugated dextran had a predominant effect on decreasing the autoxidation rate of bHb, which was particularly promising for the potential development of safe HBOCs. Conjugation with dextran is of general significance to decrease the oxidation process of the heme-containing proteins, such as Hb and myoglobin.
Introduction
Haemoglobin (Hb)-based oxygen carrier (HBOC) has been clinically developed for emergency transfusion, which has the advantage of long-term storage without cross-matching [Citation1]. However, the clinical usefulness of HBOC has been hindered by its susceptibility to autoxidation [Citation2]. HBOC is readily autoxidized from the ferrous form (Hb-Fe2+) to the non-functional ferric met form (metHb-Fe3+) [Citation3]. Autoxidation of HBOC generates harmful reactive oxygen species (ROS) such as superoxide ion and H2O2, leading to a series of tissue and cellular toxicities [Citation4,Citation5]. MetHb is susceptible to heme loss and the released heme which is toxic to DNA, proteins and lipids [Citation6]. Thus, Hb autoxidation seriously compromises the function and the safety of HBOC.
HBOC is typically prepared by chemical modification of Hb by inert polymers such as polyethylene glycol (PEG) or polymerization with glutaraldehyde [Citation7]. Recent studies have found that chemical modification decreases the stability of Hb towards oxidation and enhances the autoxidation rates of HBOCs [Citation8]. For example, glutaraldehyde-polymerized Hb and PEGylated Hb show higher autoxidation rate than Hb [Citation9,Citation10]. HBOCs are highly prone to be oxidized in the absence of the antioxidant enzymatic system and in the H2O2-rich environment [Citation11,Citation12]. Thus, the oxidative processes of HBOCs should be slowed down to alleviate the potential tissue and cellular toxicities of HBOCs. Additions of antioxidant agents (e.g. ascorbate) and conjugation of Hb with antioxidant enzymes (e.g. CAT and SOD) have been used to decrease the autoxidation rate of HBOCs [Citation13,Citation14]. However, the resultant antioxidant effects of the added agents are very limited. The added agents may also render additional tissue and cellular toxicity.
The factors that increase the autoxidation rates of HBOC have been extensively investigated. Autoxidation rates of HBOC are inversely proportional to the oxygen affinity of Hb [Citation15]. For example, human adult Hb (HbA) with a P50 of ∼14 mmHg showed a lower autoxidation rate than DBBF-Hb (diaspirin cross-linked HbA at Lys-99(α)) with a P50 of ∼35 mmHg [Citation16]. HBOC with low tetramer stability showed higher autoxidation rate than the one with high tetramer stability [Citation17]. For example, Zhang et al. proposed that there was a 17-fold increase in the autoxidation rate when tetrameric oxyHb dissociated into dimers [Citation18]. Large solvent accessibility of heme pocket has also been demonstrated to increase the autoxidation rate of HBOC [Citation10]. For example, the PEGylated Hb at Cys-93(β) with a large solvent accessible area of heme pocket showed a higher autoxidation rate than the PEGylated Hb at Val-1(β) with a small area [Citation10]. Some modification reagents (e.g. PEG) were found to enhance the autoxidation rate of Hb by binding the water (a weak nucleophile) to attack heme.
Dextran is a polysaccharide consisting of d-glucose units, and has been used for transfusion as plasma volume expander [Citation19]. The various chemical groups of dextran make it possible for conjugation of Hb [Citation20,Citation21]. The dextran-Hb conjugate (dex-Hb) is a viable HBOC that serves the dual functions of plasma volume expansion and oxygen delivery [Citation22,Citation23]. However, the autoxidation of dex-Hb in the 50% exchange transfusion model was reported to reach up to 35% in the first 12 h after transfusion [Citation24]. Recently, a novel dex-bHb was prepared by conjugation of bovine Hb (bHb) with periodate-oxidized dextran (40 kDa), where the thiol of Cys-93(β) was reversibly protected by 4,4’-dithiodipyridine (4-PDS) [Citation25]. The autoxidation rate of dex-bHb was comparable to that of bHb and it could be possibly decreased by optimization of the preparation conditions for dex-bHb.
In the present study, two dextran-bHb conjugates (dex20-bHb and dex40-bHb) were prepared by conjugation with 20 kDa or 40 kDa dextrans, where the thiol group of Cys-93(β) was reversibly protected by 4-PDS. The autoxidation rate of bHb was decreased by conjugation with 20 kDa dextran and maintained by conjugation with 40 kDa dextran. In order to understand the low autoxidation rate of dex20-bHb, the effects of aldehyde modification and conjugated dextran on the autoxidation rate of dex20-bHb were investigated. The high oxygen affinity, high tetramer stability and the conjugated dextran were found to decrease the autoxidation rate of dex20-bHb. Conjugation with dextran is of general significance to decrease the oxidation process of the heme-containing proteins, such as Hb and myoglobin.
Materials and methods
Materials
Propionaldehyde, sodium cyanoborohydride (NaCNBH3), sodium periodate, sodium azide, tris (2-carboxyethyl) phosphine (TCEP), 4,4’-dithiodipyridine (4-PDS), superoxide dismutase (SOD) and catalase (CAT) were ordered from Sigma (Santa Clara, CA). Dextran with a molecular weight (Mw) of 20 kDa (dex20) and dextran with an Mw of 40 kDa (dex40) were purchased from Pharmacia (Uppsala, Sweden). Bovine whole blood was obtained from Beijing Created Biotechnology Co. (Beijing, China). Bovine Hb was purified from bovine whole blood as described previously [Citation26].
Preparation of dex20-bHb and dex40-bHb
Dex20 (10 mg/ml, 20 ml) and dex40 (20 mg/ml, 20 ml) were oxidized by 10 mM sodium periodate in 20 mM sodium acetate buffer (pH 5.8), respectively. The reaction mixtures were kept in dark condition for 1.5 h at room temperature and stopped by addition of excessive ethylene glycol. The mixtures were subjected to extensive dialysis against PBS buffer (pH 7.4) at 4 °C. Forty millilitres of bHb (16 mg/ml) was allowed to react with 4-fold molar excess of 4-PDS in PBS buffer (pH 7.4) for 3 h at 4 °C. The resultant bHb (320 mg) was incubated with the oxidized dex20 (160 mg) and NaCNBH3 (160 mg) overnight at 4 °C to obtain the dex20-bHb conjugate (dex20-bHb). The resultant bHb (320 mg) was incubated with the oxidized dex40 (320 mg) and NaCNBH3 (160 mg) overnight at 4 °C to obtain the dex40-bHb conjugate (dex40-bHb). Afterwards, 4-fold molar excess of TCEP over bHb was added to release the thiopyridyl moiety from the disulfide moiety for 0.5 h at 4 °C. The unreacted dextran and bHb were removed from the reaction mixtures by a tangential flow filtration instrument with a 50 kDa or 100 kDa cutoff membrane. The retentates (dex20-bHb and dex40-bHb) were collected and stored at −80 °C.
Preparation of Pr-bHb
BHb (320 mg) was incubated with 7.5 μl propionaldehyde (0.8 mg/μl) and sodium cyanoborohydride (20 mg) in PBS buffer (pH 7.4) overnight at 4 °C. The modified bHb (Pr-bHb) was dialyzed extensively against PBS buffer (pH 7.4), using a 10 kDa cutoff membrane.
Quantitative assay
Dextran contents of dex20-bHb and dex40-bHb were measured colorimetrically by anthrone method [Citation27]. The bHb contents of dex20-bHb and dex40-bHb were measured using their absorbances at 540 nm. The molar ratios of dextran to bHb for dex20-bHb and dex40-bHb were obtained by comparing the dextran and bHb contents.
Titration of the thiol groups
4-Thiopyridone was released from the reaction of 4-PDS with Cys-93(β) of the bHb samples. The absorbance of the reaction mixture at 324 nm was measured as a function of incubation time to monitor the released 4-thiopyridone [Citation28]. The thiol groups of Cys-93(β) in the bHb samples were calculated by evaluation of the 4-thiopyridone content.
SDS-PAGE
SDS-PAGE analysis of the bHb samples was carried out on a 12% polyacrylamide gel. The gel was stained with Coomassie Blue R-250.
Dynamic light scattering
The molecular radii of the bHb samples were measured by dynamic light scattering, using a DynaPro Titan TC instrument (Malvern, UK). The bHb samples were all at a protein concentration of 1.0 mg/ml in PBS buffer (pH 7.4).
Size exclusion chromatography
The bHb samples were analyzed by an analytical Superdex 200 column (1 cm × 30 cm, GE Healthcare, Sunnyvale, CA), based on size exclusion chromatography (SEC). The column was equilibrated and eluted by PBS buffer (pH 7.4) at a flow rate of 0.5 ml/min at room temperature. The effluent was monitored at 280 nm.
Resonance Raman spectroscopy
Resonance Raman spectroscopy of the bHb samples was carried out by a Renishaw inVia Raman microscope (inVia plus, Renishaw, Gloucestershire, UK) at room temperature. The laser wavelength was 514 nm and the laser power was 10 mW. Raman spectra were measured for three acquisitions with an exposure time of 10 s at room temperature. The laser was focused on 300 μm under the liquid level.
Circular dichroism spectroscopy
Circular dichroism (CD) spectra of the bHb samples were recorded by a Chirascan spectropolarimeter (UK) using a 1-mm light path cuvette at 25 °C. The Soret CD spectra (360–480 nm) were obtained at a protein concentration of 1.6 mg/ml in PBS buffer (pH 7.4).
Autoxidation experiments in PBS buffer
Autoxidation experiments were conducted by incubation of the bHb samples at 37 °C in sealed tubes. The bHb samples were all at a protein concentration of 1.6 mg/ml in PBS buffer (pH 7.4) containing 0.3 mM EDTA. Aliquots of the resultant samples were taken out at various time intervals. The absorbance values of the bHb samples at 560, 576, 630 and 700 nm were measured by a spectrophotometer (Shimadzu TCC-240 A). The metHb contents of the bHb samples were determined as described previously [Citation29]. The first-order autoxidation rate constant (kox) values of the bHb samples were calculated as described previously [Citation29].
Azide-mediated oxidation of the samples
The bHb samples were all at a protein concentration of 1.6 mg/ml in PBS buffer containing 0.1 M sodium azide and 0.3 mM EDTA (pH 7.4). The bHb samples were incubated at 37 °C in sealed tubes [Citation30]. Aliquots of the resultant samples were taken out at various time intervals. The absorbance values of the bHb samples at 560, 576, 630 and 700 nm were measured to calculate the metHb contents.
Autoxidation in the presence of CAT and SOD
The bHb samples were all at a bHb concentration of 1.6 mg/ml in PBS buffer (pH 7.4) containing CAT (30 μg/ml) or SOD (12.5 μg/ml). The bHb samples were incubated at 37 °C in sealed tubes. Aliquots of the resultant samples were taken out at various time intervals. The absorbance values of the bHb samples at 560, 576, 630 and 700 nm were measured to calculate the metHb contents.
Tetramer stability
The bHb samples in PBS buffer containing 0.9 M MgCl2 and 0.3 mM EDTA (pH 7.4, buffer A) which incubated for 30 min were analyzed by a Superdex 200 column (1 cm × 30 cm, GE Healthcare, USA). The column was equilibrated and eluted by buffer A at a flow rate of 0.5 ml/min at room temperature.
Autoxidation in the presence of 0.9 M MgCl2
The bHb samples were all at a protein concentration of 1.6 mg/ml in PBS buffer (pH 7.4) containing 0.9 M MgCl2 and 0.3 mM EDTA. The bHb samples were incubated at 37 °C in sealed tubes. Aliquots of the resultant samples were taken out at various time intervals. The absorbance values of the bHb samples at 560, 576, 630 and 700 nm were measured to calculate the metHb contents.
Oxygen affinity measurements
Oxygen equilibrium curves of the bHb samples were recorded by a Hemox analyzer (TCS Scientific, New Hope, PA) at 37 °C. The bHb samples were at a protein concentration of 1.5 mg/ml in the Hemox buffer (pH 7.4). The P50 (the oxygen pressure at which Hb is half saturated) and Hill coefficient were obtained from the oxygen equilibrium curves.
Results
Characterization of the bHb samples
Size exclusion chromatography
The bHb samples were analyzed by an analytical Superdex 200 column (1 cm × 30 cm). As shown in ) Hb was eluted as a single and symmetric peak at 15.6 ml. The elution peak of Pr-bHb was at 15.3 ml and was slightly left-shifted as compared with that of bHb. In contrast, dex20-bHb and dex40-bHb were both eluted as single and broad peaks at 11.0 ml and 10.2 ml, respectively. This indicated that conjugation with dextran significantly increased the hydrodynamic volume of bHb. Moreover, the hydrodynamic volume of dex20-bHb was slightly lower than that of dex40-bHb.
Figure 1. Characterization of the bHb samples. Size exclusion chromatography analysis (a) of the bHb samples was carried out on a Superdex 200 column at room temperature. SDS-PAGE analysis (b) was carried out on a 12% Tris-glycine gel. Lane 1, molecular standards; Lane 2, bHb; Lane 3, Pr-bHb; Lane 4, dex40-bHb; Lane 5, dex20-bHb. The thiol groups of the bHb samples (c) were measured by titration with 4-PDS. Dex20-bHb-1 and dex40-bHb-1 were dex20-bHb and dex40-bHb without protection of Cys-93(β), respectively. Dynamic light scattering analysis (d) of the bHb samples was carried out by a DynaPro Titan TC instrument.
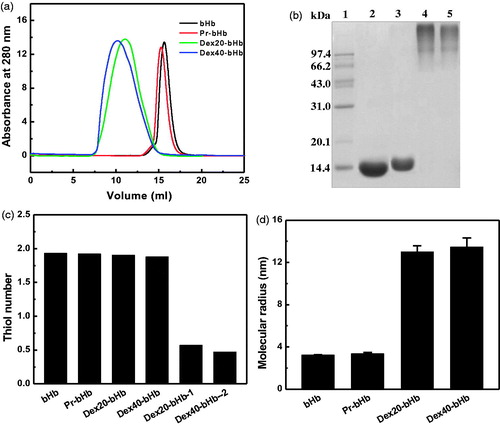
SDS-PAGE
As shown in , bHb (Lane 2) and Pr-bHb (Lane 3) both displayed a single electrophoresis band, corresponding to α- and β-subunits (∼16.0 kDa). In contrast, dex40-bHb (Lane 4) and dex20-bHb (Lane 5) both displayed a disperse electrophoresis band with much slower mobility, corresponding to an apparent Mw of over 120 kDa. The free bHb subunits were absent in dex20-bHb and dex40-bHb. This indicated that bHb tetramer (64 kDa) was intermolecularly and intramolecularly crosslinked with the multiple aldehyde moieties of dextran (20 or 40 kDa).
Quantity analysis
The dextran and bHb contents of dex20-bHb and dex40-bHb were quantitatively analyzed. The molar ratio and the mass ratio of dex20 to bHb in dex20-bHb were 1.19 and 0.37, respectively. The molar ratio and the mass ratio of dex40 to bHb in dex40-bHb were 0.62 and 0.39, respectively. Thus, the mass amount of dextran in dex20-bHb was slightly lower than that in dex40-bHb.
Thiol titration
The thiol number of the bHb samples was measured by titration with 4-PDS. As shown in , the thiol groups of the four bHb samples were all close to ∼2.0. In contrast, dex20-bHb and dex40-bHb without protection of Cys-93(β) contained 0.57 and 0.47 thiol groups per Hb tetramer, respectively. This revealed that the thiols of Cys-93(β) were essentially maintained in dex20-bHb and dex40-bHb by reversible protection of Cys-93(β) with 4-PDS.
Dynamic light scattering
DLS was used to measure the molecular radii of the bHb samples. As shown in , bHb and Pr-bHb showed the molecular radii of 3.22 and 3.34 nm, respectively. However, the molecular radius of dex20-bHb (12.99 nm) was slightly lower than that of dex40-bHb (13.46 nm). Thus, conjugation with dextran significantly increased the molecular radius of bHb, which agreed well with the results obtained from SEC and SDS-PAGE.
Structural characteristics
Resonance Raman spectroscopy
The Resonance Raman spectrum in the region of 1000–1700 cm−1 consisted of porphyrin in-plane vibrational modes, which reflected the coordination-state and spin-state of the heme iron [Citation31]. As shown in , a series of peaks were observed in the range of 1000–1700 cm−1 for the four bHb samples. BHb exhibited a mixture of six-coordinate high-spin and low-spin configurations, due to the presence of the ν2 modes at 1567 and 1589 cm−1. As compared with bHb, the other three bHb samples did not show significant difference in the coordination-state of the heme iron.
Circular dichroism spectroscopy
The CD spectrum of Hb in the Soret band region was primarily due to the interactions of the heme prosthetic group with the surrounding aromatic residues (e.g. Phe and Tyr residues). As shown in , bHb showed a maximal ellipticity at 420 nm in the Soret band region. As compared with bHb, Pr-bHb showed a slightly higher ellipticity without shift in the maximal wavelength. This suggested that chemical modification with propionaldehyde slightly perturbed the heme pocket of bHb. In contrast, dex20-bHb displayed higher ellipticity in the Soret band region than bHb, along with the maximum red shifted to 422 nm. This indicated that conjugation of bHb with dex20 facilitated the opening of heme pocket toward the solvent. Moreover, dex20-bHb showed a slightly lower ellipticity than dex40-bHb. This indicated that dex20-bHb showed a lower solvent accessible area of heme pocket than dex40-bHb.
Tetramer stability
Hb tetramers were extensively dissociated to Hb dimers in the presence of 0.9 M MgCl2 [Citation32]. Thus, the tetramer stability of the bHb samples was evaluated in the presence of 0.9 M MgCl2. As shown in , bHb and Pr-bHb in the presence of 0.9 M MgCl2 were both eluted as single elution peaks at 16.2 ml and 16.1 ml, respectively. The two elution peaks in the presence of 0.9 M MgCl2 were right-shifted as compared with bHb (15.6 ml) and Pr-bHb (15.3 ml) in the absence of 0.9 M MgCl2. The extensive tetramer dissociation of bHb and Pr-bHb reflected their low tetramer stability. The elution peaks of dex20-bHb and dex40-bHb were slightly right-shifted in the presence of 0.9 M MgCl2. This suggested that dex20-bHb and dex40-bHb both showed high tetramer stability. Moreover, the tetramer stability of dex20-bHb was higher than that of dex40-bHb, as reflected by the less peak shift of dex20-bHb (11.0 ml to 11.2 ml) than that of dex40-bHb (10.2 ml to 10.7 ml).
Figure 3. Tetramer stability of the bHb samples in the presence of 0.9 M MgCl2. bHb (1), Pr-bHb (3), dex20-bHb (5) and dex40-bHb (7) were loaded on a Superdex 200 column at room temperature, using PBS buffer containing 0.9 M MgCl2 (pH 7.4) as the eluent. bHb (2), Pr-bHb (4), dex20-bHb (6) and dex40-bHb (8) were loaded on a Superdex 200 column at room temperature, using PBS buffer (pH 7.4) as the eluent.
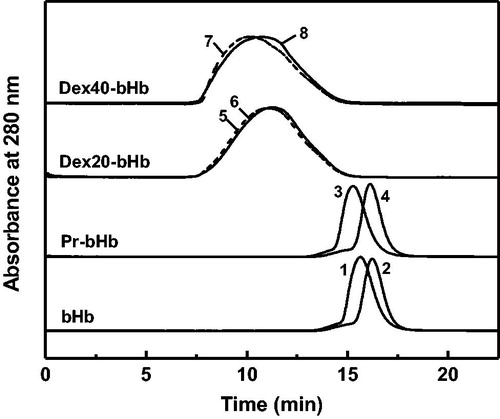
Autoxidation of the bHb samples
Autoxidation in PBS buffer
As shown in , the metHb content of bHb in PBS buffer (pH 7.4) increased as a function of incubation time at 37 °C. The kox of bHb was 0.031 h−1. The metHb content of Pr-bHb was slightly higher than those of bHb, along with a kox of 0.034 h−1. In contrast, the metHb contents of dex40-bHb were comparable to those of bHb and higher than those of dex20-bHb. The kox values of dex40-bHb and dex20-bHb were 0.031 h−1 and 0.024 h−1, respectively. This suggested that the autoxidation rate of bHb was decreased by conjugation with dex20 and maintained by conjugation with dex40.
Autoxidation in the presence of antioxidant enzymes
The autoxidation rates of the bHb samples were determined in the presence of the antioxidant enzymes, SOD and CAT. The kox values of the bHb samples in the absence of SOD and CAT were set as 100%. As shown in , the inclusion of CAT significantly decreased the autoxidation rate of the bHb samples. Further addition of SOD slightly inhibited the autoxidation rate of the bHb samples. This indicated that the produced ROS during the autoxidation process was mainly in the form of H2O2. Moreover, dex20-bHb showed the largest decrease in the kox among the four bHb samples. CAT was a powerful scavenger of H2O2 and CAT-mediated removal of H2O2 could significantly decrease the autoxidation rate of dex20-bHb.
Autoxidation in the presence of azide
Azide is a strong nucleophile that can facilitate the oxidation of bHb by stabilization of the heme in ferric state. The metHb contents of the four bHb samples in the presence of 0.1 M azide were measured as a function of incubation time. As shown in , the metHb contents of the four bHb samples were rapidly formed in the presence of 0.1 M azide, which were much higher than those in the absence of 0.1 M azide. This was due to that the azide-mediated oxidation overwhelmed the water-mediated oxidation of Hb. Pr-bHb showed a higher azide-induced oxidation rate constant (kaz, 0.150 h−1) than bHb (0.119 h−1). In contrast, the kaz values of dex20-bHb (0.441 h−1) and dex40-bHb (0.468 h−1) were much higher than that of Pr-bHb. Moreover, the metHb contents of dex20-bHb were slightly lower than those of dex40-bHb. Thus, the kaz values of the bHb samples were proportional to the solvent accessibility of heme pocket.
Autoxidation in the presence of 0.9 M MgCl2
The autoxidation rates of the four bHb samples were measured in the presence of 0.9 M MgCl2. As shown in , the metHb content of bHb increased rapidly in the presence of 0.9 M MgCl2 and was the highest among the four samples. The MgCl2-induced oxidation rate constant (kMgCl2) of bHb was 0.805 h−1. In contrast, the kMgCl2 values of Pr-bHb, dex20-bHb and dex40-bHb were 0.529, 0.057 and 0.062 h−1, respectively. Apparently, the kMgCl2 values of the bHb samples were reversely proportional to their tetramer stability. Thus, conjugation with dextran could decrease the autoxidation of bHb by increasing the tetramer stability of bHb.
Oxygen affinity
As shown in , the oxygen equilibrium curves of Pr-bHb, dex20-bHb and dex40-bHb were all left-shifted as compared with that of bHb. This indicated that the three bHb samples brought a tightened binding of oxygen. As compared with the P50 of bHb (29.21 mmHg), the P50 values of Pr-bHb, dex20-bHb and dex40-bHb were 9.14, 10.79 and 9.86 mmHg, respectively. The Hill coefficient (n) was an indicator of cooperativity in the oxygenation process of bHb. The Hill coefficient of Pr-Hb (2.13) was lower than that of bHb (2.69), indicating that aldehyde modification perturbed the subunit cooperativity of bHb. In contrast, the Hill coefficients of dex20-bHb (1.27) and dex40-bHb (1.23) were lower than that of Pr-bHb. This suggested that dextran conjugation further perturbed the subunit cooperativity of bHb.
Discussion
In the present study, the autoxidation rate of bHb was decreased by conjugation with 20 kDa dextran and maintained by conjugation with 40 kDa dextran. Pr-bHb was acted as a control for dex20-bHb and showed higher autoxidation rate than that of bHb. Dex-bHb could be considered as conjugation of bHb with dextran through propionaldehyde. Because Pr-bHb showed a kox value that was slightly higher than bHb and much lower than dex20-bHb, the conjugated dextran was deduced to have a predominant driving force to decrease the autoxidation rate of bHb.
It has been reported that PEGylation of Hb at Cys-93(β) enhanced the autoxidation rate of HbA [Citation10]. This was due to the increased solvent accessibility of heme pocket and the water molecules (a weak nucleophile) in the hydration layer of PEG that promoted the nucleophilic attack of heme iron. As revealed by the CD analysis (), the solvent accessibility of heme pocket of dex20-bHb was slightly lower than that of dex40-bHb. This could partially account for the lower autoxidation rate of dex20-bHb, even if dex20-bHb showed a larger solvent accessible area of heme pocket than bHb.
Typically, Hb with a low oxygen affinity (high P50) tended to autoxidize more readily than Hb with high oxygen affinity. Autoxidation could proceed via initial dissociation of O2 followed by binding of the nucleophile with subsequent oxidation of heme iron. Consistently, Nagababu et al. [Citation15] reported a good correlation between P50 and kox when using data obtained from HbA, bovine Hb, DBBF-Hb, bovine polymerized Hb. The effect of Hb deoxygenation on kox was suggested to be greater than any variability in the autoxidation rates. Dex20-bHb and dex40-bHb both showed lower P50 (higher oxygen affinity) than bHb. Thus, the high oxygen affinity of dex20-bHb and dex40-bHb was one of the important factor to decrease the autoxidation of bHb.
High tetramer stability has been demonstrated to decrease the autoxidation rate of Hb. Manjula et al. [Citation32] reported that intramolecular crosslink with HbA at Cys-93(β) stabilized the tetrameric structure of HbA against the presence of 0.9 M MgCl2. Hu et al. [Citation33] reported that propylbenzmethylation at Val-1(α) of HbA increased the tetramer stability and thus decreased the autoxidation of HbA. Dex20-bHb also showed extremely high tetramer stability, which was higher than that of dex40-bHb. Although the dextran mass of dex20-bHb was smaller than that of dex40-bHb, the larger molar amount of dex20 effectively stabilized the bHb tetramers through intermolecular and intramolecular crosslink of Hb subunits (). Autoxidation process of Hb was accompanied by the tetramer dissociation and subsequent globin denaturation. The high tetramer stability of dex20-bHb decreased the globin denaturation and reduced the extensive exposure of heme pocket to solvents during Hb autoxidation. Thus, the high tetramer stability of dex20-bHb was an important factor to decrease its autoxidation rate.
As a hydrophilic polysaccharide, dextran can bind the water molecules to form a hydration layer around bHb. However, the hydroxyl groups of dextran were oxidized by sodium periodate to a maximum extent, which weakened the water-binding ability of dextran. The water reservoir formed by the conjugated dextran is weaker than PEG and cannot efficiently attack the heme pocket of dex-bHb. On the other hand, the conjugated dextran could prevent the outer nucleophiles from entering the heme pocket. Moreover, dextran could structurally stabilize bHb to avoid the globin denaturation and decrease the autoxidation rate of bHb by altering the redox properties of bHb. For example, Tam et al. have proposed that the dextran moiety might hinder the steric expansion of Hb that would inhibit the denaturation rate of Hb [Citation34]. The distal His residue normally protects against autoxidation by stabilizing the bound O2 and by restricting entry of solvent water into the heme pocket. The steric shielding effect of dextran could prevent this residue from oxidative reaction by ROS.
Cys-93(β) is an important residue for the structure and function of Hb. Previously, Hu et al. [Citation10] reported that PEGylation at Cys-93(β) increased the kox of Hb by nearly ∼2.5-fold. Li et al. [Citation16] reported that reversible protection of Cys-93(β) could decrease the autoxidation rate of PEGylated Hb. In our study, the thiol group of bHb was fully protected after conjugation with dex20. Thus, protection of Cys-93(β) played a profound role in decreasing the autoxidation rate of dex-bHb. In addition, our study suggested that removal of H2O2 by addition of catalase could significantly decrease the autoxidation rate of dex20-bHb. Thus, conjugation of catalase with dex20-bHb provides a strategy to further decrease the autoxidation rate of dex20-bHb.
In summary, conjugation of bHb with 20 kDa dextran could decrease the autoxidation rate of bHb. The high oxygen affinity, high tetramer stability and the conjugated dextran were found to decrease the autoxidation rate of dex20-bHb. Thus, dex-bHb was particularly promising for the potential development of safe HBOCs. Conjugation of dextran was of general significance to decrease the oxidation process of the heme-containing proteins, such as Hb and myoglobin.
Disclosure statement
For all of the authors, no conflicts were declared.
Additional information
Funding
References
- Winslow RM. Current status of oxygen carriers ('blood substitutes'): 2006). Vox Sang. 2006;91:102–110.
- Nagababu E, Scott AV, Johnson DJ, et al. Oxidative stress and rheologic properties of stored red blood cells before and after transfusion to surgical patients. Transfusion. 2016;56:1101–1111.
- Alayash AI. Blood substitutes: why haven't we been more successful? Trends Biotechnol. 2014;32:177–185.
- Johnson RM, Goyette G Jr, Ravindranath Y, et al. Hemoglobin autoxidation and regulation of endogenous H2O2 levels in erythrocytes. Free Radic Biol Med. 2005;39:1407–1417.
- Nagababu E, Rifkind JM. Heme degradation by reactive oxygen species. Antioxid Redox Signal. 2004;6:967–978.
- Iwabuchi T, Yoshimoto C, Shigetomi H, et al. Oxidative stress and antioxidant defense in endometriosis and its malignant transformation. Oxid Med Cell Longev. 2015;2015:848595.
- Njoku M, St Peter D, Mackenzie CF. Haemoglobin-based oxygen carriers: indications and future applications. Br J Hosp Med. 2015;76:78–83.
- Jia Y, Wood F, Menu P, et al. Oxygen binding and oxidation reactions of human hemoglobin conjugated to carboxylate dextran. Biochim Biophys Acta. 2004;1672:164–173.
- Alayash AI, Summers AG, Wood F, et al. Effects of glutaraldehyde polymerization on oxygen transport and redox properties of bovine hemoglobin. Arch Biochem Biophys. 2001;391:225–234.
- Hu T, Li D, Manjula BN, et al. Autoxidation of the site-specifically PEGylated hemoglobins: role of the PEG chains and the sites of PEGylation in the autoxidation. Biochemistry. 2008;47:10981–10990.
- Hathazi D, Mot A, Vaida C, et al. Oxidative protection of hemoglobin and hemerythrin by cross-linking with a nonheme iron peroxidase: potentially improved oxygen carriers for use in blood substitutes. Biomacromolecules. 2014;15:1920–1927.
- Alayash AI. Oxygen therapeutics: can we tame haemoglobin? Nat Rev Drug Discov. 2004;3:152–159.
- Chen G, Duan Y, Liu J, et al. Antioxidant effects of vitamin C on hemoglobin-based oxygen carriers derived from human cord blood. Artif Cells Nanomed Biotechnol. 2016;44:56–61.
- Bian Y, Chang TM. A novel nanobiotherapeutic poly-[hemoglobin-superoxide dismutase-catalase-carbonic anhydrase] with no cardiac toxicity for the resuscitation of a rat model with 90 minutes of sustained severe hemorrhagic shock with loss of 2/3 blood volume. Artif Cells Nanomed Biotechnol. 2015;43:1–9.
- Nagababu E, Ramasamy S, Rifkind JM, et al. Site-specific crosslinking of human and bovine hemoglobins differentially alters oxygen binding and redox side reactions producing rhombic heme and heme degradation. Biochemistry. 2002;41:7407–7415.
- Li D, Hu T, Manjula BN, et al. Extension arm facilitated PEGylation of αα-hemoglobin with modifications targeted exclusively to amino groups: functional and structural advantages of free Cys-93(β) in the PEG-Hb adduct. Bioconjugate Chem. 2009;20:2062–2070.
- Hu T, Li D, Manjula BN, Brenowitz M, Prabhakaran M, Acharya AS. PEGylation of Val-1(alpha) destabilizes the tetrameric structure of hemoglobin. Biochemistry. 2009;48:608–616.
- Zhang L, Levy A, Rifkind JM. Autoxidation of hemoglobin enhanced by dissociation into dimers. J Biol Chem. 1991;266:24698–24701.
- Lamke LO, Liljedahl SO. Plasma volume changes after infusion of various plasma expanders. Resuscitation. 1976;5:93–102.
- Quellec P, Léonard M, Grandgeorge M, et al. Human hemoglobin conjugated to carboxylate dextran as a potential red blood cell substitute. I. Further physico-chemical characterization. Artif Cells Blood Substit Immobil Biotechnol. 1994;22:669–676.
- Bonneaux F, Dellacherie E, Labrude P, et al. Hemoglobin-dialdehyde dextran conjugates: improvement of their oxygen-binding properties with anionic groups. J Protein Chem. 1996;15:461–465.
- Tam SC, Blumenstein J, Wong JT. Soluble dextran-hemoglobin complex as a potential blood substitute. Proc Natl Acad Sci USA. 1976;73:2128–2131.
- Bonneaux F, Labrude P, Dellacherie E. Preparation and oxygen binding properties of soluble covalent hemoglobin-dextran conjugates. Experientia. 1981;37:884–886.
- Faivre B, Menu P, Labrude P, et al. Hemoglobin autooxidation/oxidation mechanisms and methemoglobin prevention or reduction processes in the bloodstream. Literature review and outline of autooxidation reaction. Artif Cells Blood Substit Immobil Biotechnol. 1998;26:17–26.
- Wang Y, Zhang S, Zhang J, et al. Structural, functional and physiochemical properties of dextran-bovine hemoglobin conjugate as a hemoglobin-based oxygen carrier. Process Biochem. 2017. DOI:10.1016/j.procbio.2017.05.021.
- Manjula BN, Acharya SA. Purification and molecular analysis of hemoglobin by high-performance liquid chromatography. Methods Mol Med. 2003;82:31–47.
- Scott RW, Moore WE, Effland MJ, et al. Ultraviolet spectrophotometric determination of hexoses, pentoses, and uronic acids after their reactions with concentrated sulfuric acid. Anal Biochem. 1967;21:68–80.
- Ampulski RS, Ayers VE, Morell SA. Determination of the reactive sulfhydryl groups in heme protein with 4,4′-dipyridinedisulfide. Anal Biochem. 1969;32:163–169.
- Benesch RE, Benesch R, Yung S. Equations for the spectrophotometric analysis of hemoglobin mixtures. Anal Biochem. 1973;55:245–248.
- Jeong ST, Ho NT, Hendrich MP, et al. Recombinant hemoglobin (α29leucine->phenylalanine, α96valine->tryptophan, β108asparagine->lysine) exhibits low oxygen affinity and high cooperativity combined with resistance to autoxidation. Biochemistry. 1999;38:13433–13442.
- Egawa T, Yeh SR. Structural and functional properties of hemoglobins from unicellular organisms as revealed by resonance Raman spectroscopy. J Inorg Biochem. 2005;99:72–96.
- Manjula BN, Malavalli A, Smith PK, et al. Cys-93-ββ-succinimidophenyl polyethylene glycol 2000 hemoglobin A. Intramolecular cross-bridging of hemoglobin outside the central cavity. J Biol Chem. 2000;275:5527–5534.
- HuLi T, Wang D, Wang J, Liang Q, Su Y, Ma Y, Su G, Wang ZS. Propylbenzmethylation at Val-1(α) markedly increases the tetramer stability of the PEGylated hemoglobin: a comparison with propylation at Val-1(α). Biochim Biophys Acta. 2012;1820:2044–2051.
- Tam SC, Wong JT. Modification of hemoglobin upon covalent coupling to dextran: enhanced stability against acid denaturation and reduced affinity for haptoglobin. Can J Biochem. 1980;58:732–736.