Abstract
Photodynamic therapy (PDT) is an effective and promising method for cancer treatment, which is proposed for more than one century. However, the specific delivery of photosensitizer to target carcinoma cells to reduce the side effect is still a great challenge. This work provides a strategy to deliver photosensitizers to cancer cells by utilizing pH-sensitive polyethylene glycol metal-phenolic network (PEG-MPN) capsules to encapsulate haematoporphyrin monomethyl ether (HMME). With the assistance of folic acid (FA), HMME-doped PEG-MPN capsules (MPN@HMMEs) accumulate in carcinoma cells selectively followed by releasing HMME in the lysosomes because of the physiologically relevant acidic pH environment. From the fluorescent ratiometric sensing and reactive oxygen species (ROS) regionality distribution of MPN@HMMEs, we demonstrated the encapsulated photosensitizers are diffused from lysosomes to cytoplasm. Under irradiation at 638 nm laser, ROS generated from the photosensitizers induced cancer cells undergoing apoptosis while normal cells survive. Therefore, MPN@HMME could be applied as a new strategy for targeted PDT against cancer and PEG-MPN capsules are expected to be general carries for drug delivering.
Introduction
Photodynamic therapy (PDT) employs ROS, especially the singlet oxygen (1O2) generated from photosensitizers, to eliminate tumour cells [Citation1–4]. Since Von Tappeiner and Jesionek sensitized tumour with eosin and proposed the principle of PDT in 1903 [Citation5], PDT for oncotherapy received much attention over one century. In 1976, Kelly and Snell first succeeded in bladder cancer treatment by PDT [Citation6], which encourages further research on PDT against cancer. Nowadays, PDT has been utilized as a promising strategy for tumour ablation [Citation3]. Great efforts have been invested in medical research and clinical treatment on PDT. But this procedure also has several challenges including targeted delivery of photosensitizer to cancer cells [Citation4]. Targeted delivery with controlled release is a developing method in precision medicine [Citation7–10]. Micro or nano probe helps people with cancer diagnosis and treatment, which could be mainly divided into three categories. The first, targeting molecules and drugs were coated on the surface of nanoparticles, e.g. quantum dots [Citation11,Citation12], superparamagnetic iron oxide nanoparticles [Citation13–15], gold nanoparticles [Citation16–18], silica nanoparticles [Citation19–21] and upconversion nanoparticles [Citation22]. After the particles accumulate in the carcinoma cells, the drugs separated from the particle surface by sorts of chemical or biological process and killed the cells. For example, Farokhzad modified DNA and PEG onto the surface of gold nanorods (GNRs) and doxorubicin intercalated between the DNA strands [Citation23]. The drug release together with cancer thermotherapy could be controlled by irradiating GNRs with near-infrared light, which has minimal absorbance by skin and tissue. The PEG layer endows the GNRs ability to escape recognition from the immune system and extends the circulation half-life of the NPs. The second, drugs were encapsulated into the mesoporous materials with chemical gates to prevent them overflowing [Citation24–26]. Once these particles were absorbed by targeted cells, the chemical gates were open by tumour markers and the drugs were released to fulfil their functions. For example, Martínez-Máñez utilized mesoporous silica nanoparticles (MSNs) to load Dox through electrostatic interaction, and then seal the pore with targeting T22 peptide analogue P by click reaction, which facilitates MSNs uptake by B-NHL cells via CXCR4 receptor [Citation27]. With the assistance of proteolytic enzymes in the lysosomes, peptide analogue P degraded and Dox was released to kill cancer cells. The third, the drugs were encapsulated into soft materials of high biocompatibility, e.g. polymer micelles [Citation28,Citation29], liposomes [Citation30–32], DNA nanocarriers [Citation33] and virus capsids [Citation34,Citation35]. The vesicle structure broke up with cargo releasing on pH changes, GSH increases or other environmental stimulations. Recently, Caruso et al. developed a facile strategy to assemble catechol complex for particle engineering which derived a polyethylene glycol metal-phenolic networks (PEG-MPN) capsule [Citation36,Citation37]. The PEG-MPN capsules release encapsulated cargo by disassembling at a physiologically relevant acidic pH, and have low cell association property endowed by the low-fouling PEG.
As inspired by Caruso’s work, we utilize this kind of capsules with modification of doping folic acid (FA) to load haematoporphyrin monomethyl ether (HMME), a water soluble photosensitizer for PDT [Citation38,Citation39], to obtain HMME-doped PEG-MPN capsules (MPN@HMMEs). This strategy pays more attention on PDT effect enhancement and improves the targeting ability to carcinoma cells of photosensitizer, leaving normal cells survived after PDT. As shown in Scheme 1, with the assistance of FA, MPN@HMMEs are absorbed by carcinoma cells via folate receptor (FR) pathway. Meanwhile, MPN@HMMEs are resistant to normal cells due to the low cell association property and low FR expression on normal cells. After FR-mediated endocytosis, the capsules are transferred to endosomes, followed by dissociation in lysosomes because of the physiologically relevant acidic pH environment. As a result, the encapsulated photosensitizers are released from lysosomes to cytoplasm. During irradiation of 638 nm laser, reactive oxygen species (ROS) generated from the photosensitizers induce the cell apoptosis.
Scheme 1. Schematic illustration of MPN@HMMEs synthesis and for selective PDT against cancer. HMME was encapsulated into PEG-MPN to form MPN@HMMEs. After FR-mediated endocytosis, MPN@HMMEs are dissociated in lysosomes to release photosensitizers to cytoplasm, followed by PDT with 638 nm laser to kill carcinoma cells.
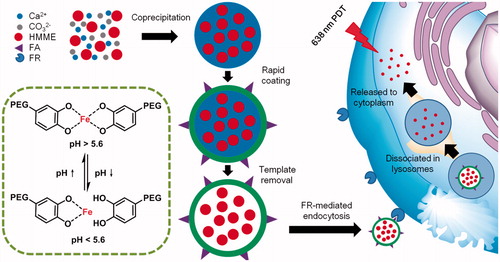
Materials and methods
Polyethylene glycol metal-phenolic networks capsules synthesis
Poly(ethylene glycol)–polyphenol (PEG-polyphenol) and FA-PEG-phenol was prepared according to a reported procedure (Scheme S1) [Citation37]. Briefly, FA-PEG-NHS (20 mg, 0.01 mmol) and dopamine hydrochloride (76 mg, 0.4 mmol) were separately dissolved in 1 mL of anhydrous dimethyl sulphoxide (DMSO) and 3 mL of anhydrous N,N-dimethylformamide (DMF). The two solutions were then mixed and degassed by N2. After stirring at room temperature under N2 protection for 2 h, the reaction mixture was purified by dialysis (1000 MWCO) for three days against 3 L of predegassed ultrapure water, followed by lyophilization to obtain FA-PEG-phenol as a light yellow powder.
HMME-doped CaCO3 templates were prepared according to a reported procedure with modification [Citation40]. To improve the stability of HMME contained capsules, HMME was firstly coupled to dextran through aqueous carbodiimide coupling chemistry. EDC (3.9 mg, 0.02 mmol) and NHS (2.3 mg, 0.02 mmol) were dissolved in 1 mL of anhydrous DMF, and then mixed with 1 mL of HMME solution (4 mg mL−1, 3.26 mM in DMF). After stirring for 30 min, 2 mL of dextran aqueous solution (2000 kDa, 5 mg mL−1) was added into the reacted bottle and stirred at room temperature for another 3 h to obtain HMME-dextran conjugates. Afterward, 625 μL of 1 M CaCl2 was added to the mixture, followed by 625 μL of 1 M Na2CO3. After vigorous stirring for 1 min, the precipitate was collected by centrifugation (800×g, 30 s) and washed three times with ultrapure water. This procedure led to form monodisperse HMME-doped CaCO3 particles.
The preparation of PEG-MPN on the surface of HMME-doped CaCO3 particles were according to a reported procedure with a little modification [Citation37]. Eight milligrams of HMME-doped CaCO3 particles with a diameter of 2.4 ± 0.3 μm were suspended in 800 μL of ultrapure water. A total of 800 μL of PEG–polyphenol solution (10 mg mL−1), 100 μL of FA-PEG-phenol (1 mg mL−1) and 1.72 mL of FeCl3·6H2O solution (1 mg mL−1) were successively added to the aqueous CaCO3 particle suspension. The suspension was vigorously stirred for 2 min after each addition of components. Subsequently, 4 mL of Tris buffer (50 mM, pH 8.5) was added to raise the pH, followed by vigorous stirring. Next, the particles were washed three times with ultrapure water by centrifugation (3000×g, 3 min) to remove impurities to harvest the PEG-MPN coated HMME-doped CaCO3 particles. Finally, the particles were washed with EDTA (200 mM, pH 8.0) to dissolve CaCO3 and then washed with ultrapure water by centrifugation (3000×g, 10 min) to obtain HMME-doped PEG-MPN capsules (MPN@HMMEs). MPN@HMMEs were redispersed in ultrapure water to a final volume of 800 μL with a concentration of 66 μg mL−1, ∼2.6 × 107 capsules mL−1, calculated by fluorescence working curve and hemocytometer. For fluorescent imaging, FITC-labelled PEG-polyphenol was used to prepare PEG-MPN capsules.
Cargo release
To explore the possibility of HMME releasing from capsules, the fluorescence intensity of MPN@HMME was monitored at an excitation wavelength of 392 nm. One millilitre of MPN@HMMEs (3 μg mL−1) in Na2HPO4 (50 mM, pH 7.4) or sodium acetate (50 mM, pH 5.0) was incubated in a thermostatic oscillation incubator (HZQ-X100, HDL, Hangzhou, China) at 37 °C with mild shaking (200 rpm). One millilitre of HMME (0.3 μg mL−1) was used as control. At designated time points, the fluorescence intensity was measured to determine the release of HMME from the capsules.
Fluorescence ratiometric properties for pH sensing
The pH-dependent fluorescence ratiometric properties of MPN@HMME were investigated by measuring the fluorescence intensities of MPN@HMMEs (3 μg mL−1) or only HMME (0.3 μg mL−1) at different pHs ranging from 4.0 to 8.5 at an excitation wavelength of 392 nm. For pH-dependent cell targeting, HeLa cells were seeded at ∼1 × 105 cells per well into six-well cell culture cluster (Thermo Fisher Scientific, Beijing, China) and incubated for 24 h. MPN@HMMEs (300 μL, 30 μg mL−1) were incubated with cells at a capsule-to-cell ratio of 3:1 for 2 h or 12 h. After rinsing with PBS twice, the cells were trypsinized and suspended in Na2HPO4 (50 mM, pH 7.4) or sodium acetate (50 mM, pH 5.0). The fluorescence intensities of cells were acquired by fluorescence spectroscopy. The same protocol without capsules incubation was performed as control.
Targeted PDT on FR+ cells
The target ability of MPN@HMMEs on FR+ cells was determined by CLSM measurements. HeLa (FR+), HaCaT (FR−) or A549 (FR+) cells were incubated with 30 μg mL−1 FITC-labelled MPN@HMME for 4 h at 37 °C [Citation41–43]. The cells absorbance circumstances of FITC-labelled capsules were observed by CLSM. To prove the selectively PDT ability of the capsules, HeLa, HaCaT or A549 cells were incubated with MPN@HMMEs and HMME, respectively, followed by irradiation with a 638 nm laser at a power of 33 mW cm−2 for 20 min. Afterward, the cells were stained with Annexin V-FITC/PI, and the cell apoptosis was visualized with CLSM.
MTT and phototoxicity assay
MTT assay was carried out to investigate the material toxicity and phototoxicity of MPN@HMMEs. HeLa cells were first seeded to three 96-well cell culture clusters at a seeding density of 1 × 104 cells per well in 200 µL complete medium, which was incubated at 37 °C for 24 h. After rinsing with PBS, two plates of HeLa cells were incubated with 200 µL culture media containing serial concentrations of FA-doped MPN@HMMEs for 4 h, another two were incubated with 200 µL culture media containing serial concentrations of MPN@HMMEs without FA-doping for 4 h. One FA-doped plate and one without FA-doping were irradiated using a 638 nm laser at a power of 30 mW cm−2 for 600 s, another two were kept in the dark for studying material toxicity. Afterward, the cells were grown for another 24 h. Then, 50 µL of 1 × MTT solution in binding buffer from MTT cell proliferation and cytotoxicity assay kit was added to each well. After 4 h incubation, the medium containing unreacted MTT was removed carefully, and 200 µL DMSO was added to each well to dissolve the produced formazan. After 1 h, the optical density (OD) at a wavelength of 490 nm was measured with microplate reader (Multiskan GO, Thermo Fisher Scientific, Beijing, China). Phototoxicity assay was further used to study the influence of radiation quantity on capsule-loaded cells. HeLa cells (1 × 105 per well) were seeded on six-well cell culture clusters and incubated in complete medium for 24 h at 37 °C. The medium was then replaced with fresh culture medium containing 30 μg mL−1 MPN@HMMEs to incubate for 4 h at 37 °C. The cells were irradiated with a 638 nm laser at a power of 33 mW cm−2 for 10, 20 and 40 min, respectively. Afterward, the cells were trypsinized, harvested, rinsed with PBS, resuspended, and stained with Annexin V-FITC/PI according to the manufacturer’s instruction, subjected to perform flow cytometric assay (BD Accuri C6, BD Biosciences, Shanghai, China). All experiments detected at least 10,000 cells.
Cellular ROS detection during irradiation
To obtain the level of intracellular ROS related to MPN@HMMEs treatment, HeLa cells were first incubated with MPN@HMMEs, followed by further incubation with 10 μM DCFH-DA for 20 min and irradiated with a 638 nm laser at a power of 28 mW cm−2 for 0, 3, 6 and 9 min to perform the fluorescence detection of DCF with the flow cytometer, respectively.
Vitamin C and NAC were also used in examining ROS induced by PDT. After the capsule-loaded cells were incubated with 2.5 mM vitamin C or NAC for 30 min and irradiated at a power of 33 mW cm−2 for 10 min, the cells were stained with Annexin V-FITC/PI to visualize the cell apoptosis with CLSM.
To investigate the subcellular localization of ROS generated by PDT, HeLa cells were incubated with 30 μg mL−1 MPN@HMMEs for 2 or 12 h, followed by staining with DCFH-DA and LysoTracker Blue for 15 min. After irradiation under 638 nm laser at a power of 33 mW cm−2 for 10 min, the cells were observed by CLSM.
Results and discussion
Synthesis and characterization of MPN@HMMEs
HMME-doped CaCO3 templates were prepared by a simple coprecipitation reaction. HMME was coupled to dextran through aqueous carbodiimide coupling chemistry to improve the homogeneity and stability of HMME contained capsules. The as-prepared HMME-doped CaCO3 nanoparticles were spherical with an average diameter of 2.4 ± 0.6 μm (Figures S1a and S2). Flat fluorescent spot shows that HMMEs are evenly distributed inside CaCO3 nanoparticles (Figure S1c). To maximize the drug loading capacity, we use different initial concentration of HMME ranging from 0.4 mg mL−1 to 3 mg mL−1 to synthesis CaCO3 templates. The drug loading capacity reaches maximum at the concentration of 1 mg mL−1, and the loaded HMME is about 7.1 pg capsule−1, calculated through HMME standard working curve (Figures S3 and S4).
To endow MPN@HMME with high resistant to nonspecific cell interaction, 8-arm-PEG was coupled to phenol to form PEG-polyphenol [Citation37], while FA-PEG was linked with phenol to form FA-PEG-phenol for targeting carcinoma cells (Scheme S1). The resulting PEG-polyphenol and FA-PEG-phenol (FITC-labelled PEG for fluorescence imaging) could be covered on the surface of HMME-doped CaCO3 nanoparticles through the strong surface binding affinity of catechol groups, which subsequently formed the metal-phenolic networks in the presence of FeIII. Scanning electron microscopy (SEM, ) and reversed biological microscopy () exhibited that the PEG-MPN coated CaCO3 particles are smoothly spherical and monodispersed. The coating of PEG-MPN on HMME-doped CaCO3 particles was proved by differential interference contrast (DIC, ) microscopy and fluorescence microscopy, where red cores assigned to HMME-doped CaCO3 nanoparticles () along with green shells corresponding to FITC-labelled PEG-MPNs () were clearly observed (). After removing the CaCO3 templates by EDTA, monodisperse, hollow () and spherical () MPN@HMMEs were obtained with a diameter of 2.3 ± 0.3 μm (Figure S5), which was slightly smaller than that of CaCO3 template contained due to the pinch effect of the metal-phenolic networks. Capsules present the vesicle structure by DIC (), and dextran-HMMEs were still in the capsules (). SEM exhibited MPN@HMMEs collapsed with folds and creases after air-drying, which are typical features of hollow polymer capsules (). Increased zeta potential from CaCO3 (−4.1 mV) to capsules (−8.2 mV) was caused by the hydrophilic nature of PEG. When the capsule was prepared by using CaCO3 without HMME doping, the zeta potential further decreased to −14.6 mV due to the electropositivity of HMME (Figure S6). The mass of capsule is about 0.254 pg capsule−1, calculated from the standard working curve of FITC-labelled PEG-polyphenol (Figure S7). The fluorescence quantum yields and singlet oxygen quantum yields of MPN@HMMEs are about half of HMMEs and affected a little by pH changes (Table S1 and Figure S8), while the fluorescence lifetime of both MPN@HMMEs and HMME remains 14.4 ns (Figure S9). In pH 5.0, the fluorescence quantum yield is a little higher than that in pH 7.4. This phenomenon may be caused by the PEG-MSN encapsulation of HMME, which has larger absorption at 392 nm in pH 7.4 than that in pH 5.0 [Citation37]. The acidic environment facilitates the protonation of pyrrole nitrogen atoms in HMME and changes the symmetry of the conjugated ring and the absorbance of both Soret band and Q band, leading to a little lower singlet oxygen quantum yields of MPN@HMMEs in pH 5.0 [Citation44].
pH-dependent cargo release
Ideal carriers are stable at physiological pH and tend to disassemble in the acidic intracellular compartments, for example, endosomes and lysosomes for drug delivery. Previous studies demonstrated that the stability of the metal-phenolic networks depended on pH value and the cross-linked metal-phenolic networks by catechol groups and FeIII can be cleaved by low pH, which fulfil the function of pH-dependent cargo release of MPN@HMMEs [Citation37]. The cargo release capacity of MPN@HMMEs was determined by the normalized fluorescence intensity which was calibrated by the fluorescence decay of HMME releasing from MPN@HMMEs at different pHs. As shown in , for the first 5 h, the HMME contents of MPN@HMME declined gently at pH 7.4 while dropped abruptly at pH 5.0. After 22 h incubation, the HMME remained in the capsule was about 60% at pH 7.4 while 8% at pH 5.0. This was caused by the disassembly of the capsules in acidic environment. The cleaver of MPN@HMME was demonstrated by dynamic light scattering of the capsules at different pHs (Figure S5). The size of MPN@HMME capsules was 1.2 μm incubated at pH 7.4 while 0.6 μm at pH 5.0, exhibiting low pH environment smashed capsules into smaller pieces by breaking up the coordinate bond between catechol groups and FeIII.
Figure 2. (a) Release of encapsulated HMME from MPN@HMMEs (3 μg mL−1) at pH 7.4 and 5.0 in 37 °C, respectively. The normalized fluorescence intensity from HMME in MPN@HMMEs was monitored by fluorescent spectrometry, λex = 392 nm, λem = 620 nm; (b) plots of fluorescence ratio of I620 nm/I670 nm versus pH, λex = 392 nm.
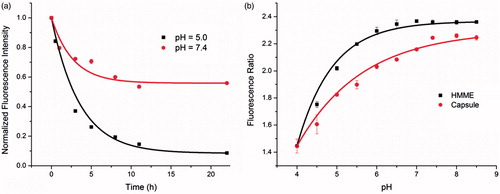
Fluorescence ratiometric properties for pH sensing
The fluorescence intensity of MPN@HMME capsules changes with both pH (Figure S10a) and concentration (Figure S10b), but the fluorescence ratio of I620 nm/I670 nm, which is invariant with concentration and time (Figures S11a and S11b), only depends on pH changes especially on the pH ranges from 4.4 to 7.4 (). According to the corresponding ratio value, the MPN@HMME capsule (red curve) or HMME itself (black curve) has the ability to indicate pH ranging from 4.0 to 8.5. This property could be utilized to detect the location of the capsules exist in HeLa cells. HeLa cells were incubated with capsules for 2 or 12 h, followed by trypsinizing and suspending in pH 5.0 and pH 7.4 buffers, respectively (Figure S12). Fluorescence ratio exhibited that the capsules stayed in acidic organelles (4.54 and 4.34 at environment pH 5.0 and 7.4, respectively) like endosomes or lysosomes after 2 h incubation, while dispersed in neutral cytoplasm (6.69 and 6.16 at environment pH 5.0 and 7.4, respectively) after 12 h incubation. This also demonstrated that different environment pHs had a little influence on the regionality distribution of capsules in HeLa cells.
Cytotoxicity of PEG-MPN capsules
The cytotoxicity of MPN@HMME capsules to HeLa cells was investigated using MTT assay (). Due to excellent anti-fouling ability of PEG, the capsules without FA-doping were basically noncytotoxic in the absence of light with the cell viability of 90% (red curve). As to the FA-doped capsules, the cell viability decreased slightly to 80% approximately (blue curve), which may attribute to the cellular uptake of MPN@HMME capsules. During two days incubation of MPN@HMME capsules, the cell viability remained at 90% (Figure S13), indicating MPN@HMME capsules possess a good biocompatibility. FA-doped capsules exhibited higher phototoxicity under irradiation of 18 J cm−2 (black curve) than capsules without FA-doping (magenta curve), and the phototoxicity increased along with the concentration of capsules with a half lethal dose (IC50) of 20 μg mL−1. Moreover, the cell apoptosis induced by the capsule-mediated PDT were determined with the dual fluorescence of Annexin V-FITC/PI by flow cytometry. The cell populations at different phases stands for viable cells (AnnexinV-FITC−/PI−), early apoptosis (Annexin V-FITC+/PI−), necrosis (AnnexinV-FITC−/PI+), and late-stage apoptosis (Annexin V-FITC+/PI+) are shown in with different treatments. When the cells were treated with either laser or capsules alone, most of the cells keep viability with a cell mortality rate below 10% (), further confirming the low material cytotoxicity of MPN@HMME capsules. The cell mortality rate and late-stage apoptotic rate increased upon the combining utilization of MPN@HMME capsules and increasing dose of laser from 20 J cm−2 to 80 J cm−2 (), implying the application of MPN@HMME capsules in PDT against cancer is promising.
Figure 3. Viability of HeLa cells treated with capsules or FA-doped capsules under different concentration before and after PDT with irradiation dose of 18 J cm−2.
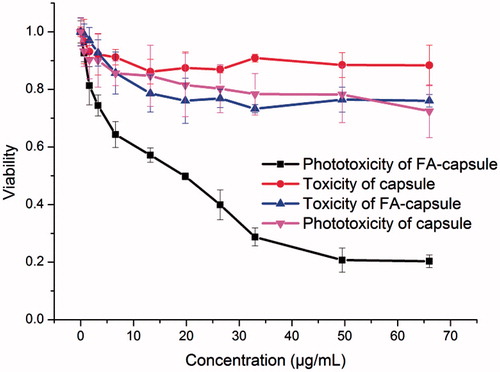
Figure 4. Flow cytometric detection of HeLa cell apoptosis induced by capsule-mediated PDT. HeLa cells (1 × 105) per well were seeded on six-well cell culture clusters and incubated in complete medium for 24 h at 37 °C, then incubated with MPN@HMMEs (30 μg mL−1) for 4 h at 37 °C. The cells were irradiated with a 638 nm laser at an energy density of 0 (c), 20 (d), 40 (e) and 80 (f) J cm−2, respectively. The HeLa cells untreated (a) and irradiated (b) used as controls.
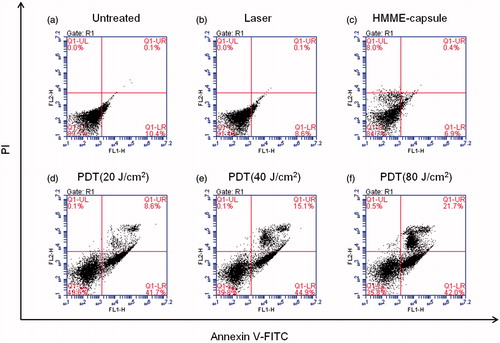
Selectivity of PEG-MPN capsules
To investigate the selectivity of MPN@HMME capsules to carcinoma cells, HeLa cells were incubated with MPN@HMME capsules without FA-doping or FA-doped MPN@HMME capsules for 4 h and then irradiated with a 638 nm laser at a power of 33 mW cm−2 for 10 min. After staining with Annexin V-FITC/PI, the cell apoptosis was visualized by CLSM. The capsules without FA-doping made cells display invisible fluorescence of Annexin V-FITC/PI, while FA-doped capsules made cells exhibit intense fluorescence with apoptotic characteristics (Figure S14). Thus HeLa cells uptake the FA-doped MPN@HMME capsules via FR-mediated endocytosis which could distinguish cancer from normal cells. Further, quantitative data of cellular uptake were investigated by comparing the fluorescence intensity of FA-MPN@HMME capsules before and after incubated with HeLa cells (Figure S15). The uptake percentage of capsules approaches saturation upon 3.3 μg mL−1, while the saturated cellular uptake quantity is about 2.3–3.1 cap per cell. On the other hand, when incubation of HeLa, HaCaT and A549 cells occur with FITC-labelled HMME-loaded capsules, respectively, the capsule fragments (green) and HMME components (red) were dispersed in FR+ cells (HeLa and A549), while not spread in FR− cells (HaCaT), manifesting the selective uptake of the capsules to carcinoma cells (). To investigate the selectable capsules injury to cancer cells during PDT, HeLa, HaCaT and A549 cells were incubated with MPN@HMME capsules () or HMME (), followed by PDT with a 638 nm laser at a power of 33 mW cm−2 for 10 min and staining with Annexin V-FITC/PI. Only FR− cells (HaCaT) loaded with MPN@HMME capsules showed weak apoptotic fluorescence, indicating MPN@HMME capsules do not injure normal cells and have more advantages than HMME on PDT in selectivity.
Figure 5. CLSM analysis of capsules’ targeting ability. HeLa (FR+), HaCaT (FR−) or A549 (FR+) cells were incubated with 30 μg mL−1 FITC-labelled MPN@HMMEs (a) for 4 h at 37 °C; HeLa, HaCaT or A549 cells were incubated with 30 μg mL−1 of MPN@HMMEs (b) and HMME (c), respectively, followed by irradiation with a 638 nm laser at energy density of 40 J cm−2 and Annexin V-FITC/PI staining. Scale bars are 30 μm.
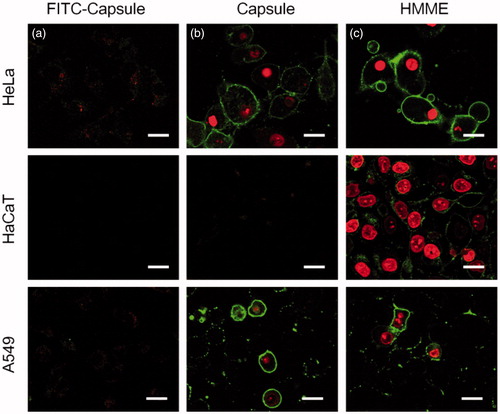
Contribution of reactive oxygen species to PDT
We stained the capsule-loaded HeLa cells with DCFH-DA to clarify the role of ROS in the capsule-mediated PDT, where non-fluorescent DCFH-DA transformed into green fluorescent DCF upon reacting with ROS. As shown in , ROS detection by a flow cytometry revealed that the production of ROS was induced by MPN@HMME capsules with PDT. With the increase of irradiation dose from 0 to 15 J cm−2, the relative mean fluorescent intensity of DCF was enhanced from 1.2 to 5.9, to deduce that the amount of ROS was dependent on the irradiation dose (, inset graph).
Figure 6. Flow cytometric detection of ROS generated during capsule-mediated PDT with a ROS probe, DCFH-DA. HeLa cells (1 × 105) per well were seeded on six-well cell culture clusters and incubated in complete medium for 24 h at 37 °C, then incubated with MPN@HMMEs (30 μg mL−1) for 4 h at 37 °C. HeLa cells were incubated with 10 μM DCFH-DA for 20 min and irradiated with a 638 nm laser at energy densities of 0, 5, 10, 15 J cm−2; and plot of ROS generation versus irradiation dose of 638 nm laser, ROS generation was defined as the ratio of mean fluorescent intensity of DCF at different irradiation dose to the control (inset graph).
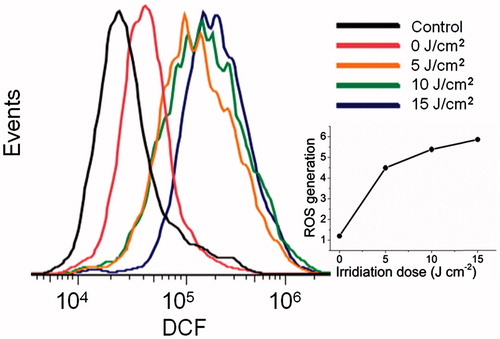
Vitamin C or NAC is common ROS scavengers, which could efficiently inhibit cell apoptosis originated from PDT [Citation45]. To clarify whether ROS was responsible for the cell apoptosis in the capsule-mediated PDT, the capsule-loaded HeLa cells were incubated with vitamin C or NAC followed by irradiation with 20 J cm−2 638 nm laser. After PDT, the HeLa cells showed cell shrinkage and Annexin V-FITC+/PI+ staining () indicating cell apoptosis. In the presence of vitamin C or NAC in the incubation solution, the HeLa cells exhibited AnnexinV-FITC−/PI− staining (), indicating cell survival with the protection of vitamin C or NAC. This also illustrated that the generated ROS in capsule-mediated PDT was responsible for the cell apoptosis.
Figure 7. Confocal fluorescence images of Annexin V-FITC/PI stained HeLa cells with different treatments: (a) the capsule-loaded HeLa cells were incubated without (b) or with NAC (c) or vitamin C (d) followed by irradiation with 20 J cm−2 638 nm laser. Untreated HeLa cells set as control. Scale bars are 30 μm.
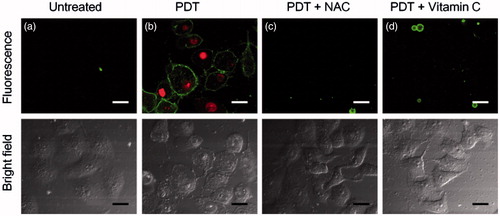
To elucidate the mechanism of capsule-mediated PDT, the subcellular ROS localization was observed by CLSM. Before irradiation, the HeLa cells loaded with MPN@HMME capsules showed little self-originating ROS generation (). Upon irradiation, the fluorescence of DCF enhanced significantly and well overlapped with the lysosomal tracker (LysoBlue in ) for HeLa cells incubated with capsules for 2 h, indicating that ROS was originated in lysosomes. This suggested that the acidic pH environment (4.5–5.5) in lysosomes helps releasing HMME from capsules for PDT and the cell apoptosis induced by the capsule-mediated PDT involved a lysosome-associated pathway. For cells incubated with capsules for 12 h (), the ROS location was separated from lysosomes and distributed in cytoplasm, indicating HMME was released from lysosomes to cytoplasm. In summary, MPN@HMME capsules get into HeLa cells via FR-mediated endocytosis and accumulate in endosomes and lysosomes, which is good constant with the detection of fluorescence ratio for the time-dependent regionality distribution of capsules in HeLa cells. Due to the acidic environment, HMME release from the capsules to lysosomes after 2 h or to cytoplasm after 12 h. ROS are generated in either lysosomes or cytoplasm upon irradiation and induce cell apoptosis to achieve PDT.
Conclusions
MPN@HMMEs have been synthesized by encapsulating HMME in the PEG-MPN capsules to achieve selective and efficient PDT for cancer treatment. The MPN@HMME capsules play a role as an acidic pH-activatable carrier for controllable photosensitizer release in PDT. The pH-controllable activity is caused by the metal-phenolic networks decomposing under acidic environment. MPN@HMMEs have low cytotoxicity and high bio-security due to the anti-fouling ability of PEG-MPN. By FA doping on the capsule surface, MPN@HMMEs target to FR overexpressed carcinoma cells and ignore normal cells. After endocytosis, MPN@HMMEs release photosensitizer in lysosomes and cytoplasm, which is demonstrated by fluorescent ratiometric and imaging measurements. During irradiation, HMMEs induce the generation of 1O2 in lysosomes and cytoplasm to kill carcinoma cells. After all, this work provides a new strategy to deliver photosensitizer to carcinoma cells, overcoming the low selectivity of HMME in PDT.
Yuanqing_et_al._Supplementary_material.docx
Download MS Word (4.9 MB)Disclosure statement
The authors declare no competing financial interest.
Additional information
Funding
References
- Moan J, Peng Q. An outline of the hundred-year history of PDT. Anticancer Res. 2003;23:3591–3600.
- Castano AP, Mroz P, Hamblin MR. Photodynamic therapy and anti-tumour immunity. Nat Rev Cancer. 2006;6:535–545.
- Lovell JF, Liu TWB, Chen J, et al. Activatable photosensitizers for imaging and therapy. Chem Rev. 2010;110:2839–2857.
- Zhou Z, Song J, Nie L, et al. Reactive oxygen species generating systems meeting challenges of photodynamic cancer therapy. Chem Soc Rev. 2016;45:6597–6626.
- Von Tappeiner H, Jesionek A. Therapeutische versuche mit fluoreszierenden stoffen. Münch Med Wochenschr. 1903;47:2042–2044.
- Kelly JF, Snell ME. Hematoporphyrin derivative: a possible aid in the diagnosis and therapy of carcinoma of the bladder. J Urol. 1976;115:150–151.
- Kim BYS, Rutka JT, Chan WCW. Nanomedicine. N Engl J Med. 2010;363:2434–2443.
- Hubbell JA, Chilkoti A. Chemistry. Nanomaterials for drug delivery. Science. 2012;337:303–305.
- Mitragotri S, Lahann J. Materials for drug delivery: innovative solutions to address complex biological hurdles. Adv Mater Weinheim. 2012;24:3717–3723.
- Sun T, Zhang YS, Pang B, et al. Engineered nanoparticles for drug delivery in cancer therapy. Angew Chem Int Ed Engl. 2014;53:12320–12364.
- Probst CE, Zrazhevskiy P, Bagalkot V, et al. Quantum dots as a platform for nanoparticle drug delivery vehicle design. Adv Drug Deliv Rev. 2013;65:703–718.
- Bajwa N, Mehra NK, Jain K, et al. Pharmaceutical and biomedical applications of quantum dots. Artif Cells Nanomed Biotechnol. 2016;44:758–768.
- Wang D, Fei B, Halig LV, et al. Targeted iron-oxide nanoparticle for photodynamic therapy and imaging of head and neck cancer. ACS Nano. 2014;8:6620–6632.
- Wei Z, Wu Y, Zhao Y, et al. Multifunctional nanoprobe for cancer cell targeting and simultaneous fluorescence/magnetic resonance imaging. Anal Chim Acta. 2016;938:156–164.
- Ebrahimi E, Akbarzadeh A, Abbasi E, et al. Novel drug delivery system based on doxorubicin-encapsulated magnetic nanoparticles modified with PLGA-PEG(1000) copolymer. Artif Cell Nanomed Biotechnol. 2016;44:290–297.
- Ghosh P, Han G, De M, et al. Gold nanoparticles in delivery applications. Adv Drug Deliv Rev. 2008;60:1307–1315.
- Giljohann DA, Seferos DS, Daniel WL, et al. Gold nanoparticles for biology and medicine. Angew Chem Int Ed Engl. 2010;49:3280–3294.
- Daraee H, Eatemadi A, Abbasi E, et al. Application of gold nanoparticles in biomedical and drug delivery. Artif Cells Nanomed Biotechnol. 2016;44:410–422.
- Wu YF, Zhou H, Wei W, et al. Signal amplification cytosensor for evaluation of drug-induced cancer cell apoptosis. Anal Chem. 2012;84:1894–1899.
- Li HN, Mu YW, Lu JS, et al. Target-cell-specific fluorescence silica nanoprobes for imaging and theranostics of cancer cells. Anal Chem. 2014;86:3602–3609.
- Li H, Mu Y, Qian S, et al. Synthesis of fluorescent dye-doped silica nanoparticles for target-cell-specific delivery and intracellular MicroRNA imaging. Analyst. 2015;140:567–573.
- Shen J, Zhao L, Han G. Lanthanide-doped upconverting luminescent nanoparticle platforms for optical imaging-guided drug delivery and therapy. Adv Drug Deliv Rev. 2013;65:744–755.
- Xiao Z, Ji C, Shi J, et al. DNA self-assembly of targeted near-infrared-responsive gold nanoparticles for cancer thermo-chemotherapy. Angew Chem Int Ed Engl. 2012;51:11853–11857.
- Li ZX, Barnes JC, Bosoy A, et al. Mesoporous silica nanoparticles in biomedical applications. Chem Soc Rev. 2012;41:2590–2605.
- Coll C, Bernardos A, Martínez-Máñez R, et al. Gated silica mesoporous supports for controlled release and signaling applications. Acc Chem Res. 2013;46:339–349.
- Aznar E, Oroval M, Pascual L, et al. Gated materials for on-command release of guest molecules. Chem Rev. 2016;116:561–718.
- de la Torre C, Casanova I, Acosta G, et al. Gated mesoporous silica nanoparticles using a double-role circular peptide for the controlled and target-preferential release of doxorubicin in CXCR4-expresing lymphoma cells. Adv Funct Mater. 2015;25:687–695.
- Tanner P, Baumann P, Enea R, et al. Polymeric vesicles: from drug carriers to nanoreactors and artificial organelles. Acc Chem Res. 2011;44:1039–1049.
- Nicolas J, Mura S, Brambilla D, et al. Design, functionalization strategies and biomedical applications of targeted biodegradable/biocompatible polymer-based nanocarriers for drug delivery. Chem Soc Rev. 2013;42:1147–1235.
- Mikhaylov G, Mikac U, Magaeva AA, et al. Ferri-liposomes as an MRI-visible drug-delivery system for targeting tumours and their microenvironment. Nat Nanotechnol. 2011;6:594–602.
- Allen TM, Cullis PR. Liposomal drug delivery systems: from concept to clinical applications. Adv Drug Deliv Rev. 2013;65:36–48.
- Yadav S, Gupta S. Development and in vitro characterization of docetaxel-loaded ligand appended solid fat nanoemulsions for potential use in breast cancer therapy. Artif Cells Nanomed Biotechnol. 2015;43:93–102.
- Bhatia D, Surana S, Chakraborty S, et al. A synthetic icosahedral DNA-based host-cargo complex for functional in vivo imaging. Nat Commun. 2011;2:339–346.
- Yoo J-W, Irvine DJ, Discher DE, et al. Bio-inspired, bioengineered and biomimetic drug delivery carriers. Nat Rev Drug Discov. 2011;10:521–535.
- Li J, Fan C, Pei H, et al. Smart drug delivery nanocarriers with self-assembled DNA nanostructures. Adv Mater Weinheim. 2013;25:4386–4396.
- Ejima H, Richardson JJ, Liang K, et al. One-step assembly of coordination complexes for versatile film and particle engineering. Science. 2013;341:154–157.
- Ju Y, Cui J, Müllner M, et al. Engineering low-fouling and pH-degradable capsules through the assembly of metal-phenolic networks. Biomacromolecules. 2015;16:807–814.
- Ding X, Xu Q, Liu F, et al. Hematoporphyrin monomethyl ether photodynamic damage on HeLa cells by means of reactive oxygen species production and cytosolic free calcium concentration elevation. Cancer Lett. 2004;216:43–54.
- Lei TC, Glazner GF, Duffy M, et al. Optical properties of hematoporphyrin monomethyl ether (HMME), a PDT photosensitizer. Photodiagn Photodyn Ther. 2012;9:232–242.
- Petrov AI, Volodkin DV, Sukhorukov GB. Protein-calcium carbonate coprecipitation: a tool for protein encapsulation. Biotechnol Prog. 2005;21:918–925.
- Chiani M, Norouzian D, Shokrgozar MA, et al. Folic acid conjugated nanoliposomes as promising carriers for targeted delivery of bleomycin. Artif Cells Nanomed Biotechnol. 2017; [2017 Jun 23]; [7 p.]. DOI:10.1080/21691401.2017.1337029
- Kaittanis C, Santra S, Perez JM. Role of nanoparticle valency in the nondestructive magnetic-relaxation-mediated detection and magnetic isolation of cells in complex media. J Am Chem Soc. 2009;131:12780–12791.
- Santra S, Kaittanis C, Santiesteban OJ, et al. Cell-specific, activatable, and theranostic prodrug for dual-targeted cancer imaging and therapy. J Am Chem Soc. 2011;133:16680–16688.
- Al-Khaza’leh KA, Omar K, Jaafar MS, et al. Effect of acidification on the absorption and fluorescence properties of Sn(IV) chlorin e6 dichloride trisodium salt. Arab J Sci Eng. 2011;36:597–606.
- Tian J, Ding L, Xu H-J, et al. Cell-specific and pH-activatable rubyrin-loaded nanoparticles for highly selective near-infrared photodynamic therapy against cancer. J Am Chem Soc. 2013;135:18850–18858.