Abstract
Cationic polymers are characterized as the macromolecules that possess positive charges, which can be either inherently in the polymer side chains and/or its backbone. Based on their origins, cationic polymers are divided in two category including natural and synthetic, in which the possessed positive charges are as result of primary, secondary or tertiary amine functional groups that could be protonated in particular situations. Cationic polymers have been employed commonly as drug delivery agents due to their superior encapsulation efficacy, enhanced bioavailability, low toxicity and improved release profile. In this paper, we focus on the most prominent examples of cationic polymers which have been revealed to be applicable in drug delivery systems and we also discuss their general synthesis and surface modification methods as well as their controlled release profile in drug delivery.
Introduction
In recent years, nanotechnology is commonly applied in numerous applications including fibre and textiles, forensic science, electronics and medical therapeutics [Citation1–4]. However, polymeric nanoparticles are currently employed to enhance the therapeutic efficacy of numerous water soluble/insoluble drugs and bioactive molecules by increasing their solubility, retention time and stability [Citation5]. Capsulation of drugs into nanoparticles improves their tolerability, therapeutic efficacy and the therapeutic index of corresponding drugs and prevents immediate degradation and enhances absorption of drugs into the selected tissue. Nanomedicine formulation relies on the select of appropriate polymeric system with higher encapsulation efficiency, better retention time and bioavailability. Nowadays, creating the novel carriers for delivery of therapeutic agents to target cells, passively or actively, is absolutely a critical issue for the useful drug doses to attain the illness area of interest with no harmful effect on the surrounding health cells. This idea includes a delivery system with nanometric size which is capable of targeting specific tumour tissue and avoids premature degradation [Citation6,Citation7]. Particle size, surface modification, hydrophobicity and surface charge are the significant parameters which determine targeting abilities of nanocarriers and influence their passage across the physiological barriers. Surface charge, or zeta potential of nanocarriers impacts their interactions with cells membrane or blood proteins. A wide range of experiments has been developed based on negatively charged particles, while, comparatively slight attention has been given to positively charged nanocarriers. The idea of facilitating cellular uptake of cationic vectors has been proven in the case of gene therapy with cationic micelles. Employing of cationic nanocarriers, allows loading of hydrophobic drugs or DNA molecules which are non-permeable to the cell membrane and results in facile endocytosis. In recent years, cationic polymers nanoparticles and nanogels showed superior properties including strong interaction with DNA and the ability to provide oriented bonds with proteins, which introduce them as ideal choices in emerging biomedical applications. Current anionic pH-sensitive polymeric particles which are synthesized as drug delivery vehicles show swelling behaviour at high pH which is not suitable for delivery to the acidic surroundings present in tumour tissues. In this case, cationic monomers exhibit acid-swellable behaviour which makes them a good candidate for drug carriers. Unprotected cationic nanoparticles with size of 100–200 nm have a higher removal velocity than those with negative charge. From the point of view of enhancing circulation time and long existence in the blood, the surface of conventional nanoparticles can be modified with various molecules such as hydrophilic polymers [Citation8,Citation9], which enhances their hydrophilicity at the physiological environment. At the following, we discuss the most common synthesis methods and surface modification techniques of both natural and synthetic cationic polymers. We also focus on the most recent studies that employed cationic polymers as drug delivery agents and highlight their results.
Natural cationic polymers (NCPs)
Natural cationic polymers (NCPs) own intrinsic positive charges and are originated from renewable sources and also characterized as biodegradable structures which have low toxicity and low immunogenicity. Through facile modification of reactive sites existing at the most NCPs, their physicochemical properties can be easily improved. These properties make NCP nanoparticles as a promising candidate for various therapeutic applications including drug delivery, gene therapy and tissue engineering. Here in this section, we discuss synthesis methods and drug encapsulation procedures of NCPs. We summarized the chemical structures of the most recognized NCPs and their related biomedical applications in .
Table 1. Summary of the chemical structures of the most widely applied NCPs and their related applications in biomedicine.
Cationic gelatin
Gelatin is an inexpensive, biodegradable and biocompatible natural polymer which is originated from collagen and widely employed for pharmaceutical and biomedical applications [Citation10,Citation23–25]. Gelatin contains 18 non-uniformly dispersed amino acids with both negative and positive charges in which the arginine and lysine residues cause the intrinsic cationic property of gelatin. Based on reports, the crosslinking degree of gelatin determines its thermal properties, swelling behaviour and mechanical properties. Gelatin can be obtained by the alkaline and acidic treatment of collagen. The alkaline procedure aims the amide groups of glutamine and asparagine and hydrolysis them into carboxyl groups, resulting in gelatin B with a higher amount of carboxyl groups (high negative charge) and an isoelectric point of 4.7–5.4. In contrast, acidic process results in gelatin A with an isoelectric point of 6–9, in which the amide groups remain comparatively unaffected and gelatin possesses almost positive charge (). Gelatin has been approved by the US Food and Drug Administration (FDA) as a safe material, which is widely applied as a constituent of different biomaterials.
Morimoto et al. [Citation26] have produced cationic gelatins by the reaction of native gelatin with 1, 2-ethylenediamine. They dissolved cationic gelatin in 1, 2-ethylenediamine and phosphate buffer (pH 5.3), which was added under stirring, then the PH was adjusted to 5.0 using 5 M HCl and 1-ethyl-3-(3-dimethyl-aminopropyl)-carbodiimide hydrochloride. As a result, the amino group density of the native gelatin and aminated gelatin were 0.33 and 0.64 mmol/g, respectively [Citation26]. Gelatin nanoparticles (GNPs) can be obtained via various method including desolvation [Citation27], emulsification-solvent evaporation [Citation28], coacervation-phase separation [Citation29] and reverse phase microemulsion [Citation30]. In two-step desolvation technique, gelatin type A was dissolved in 25 ml water under heating and then 25 ml of acetone was added to the solution in order to accomplish the desolvation and fast sedimentation of the gelatin. The low molecular gelatin portions existing in the supernatant were discarded by decanting. In the second step, the high molecular portions existing in the sediment were redesolved and then desolvated again at pH 2.5 and then the obtained particles were easily purified by centrifugation and redispersion.
Pharmaceutical applications of GNPs
In recent years, GNPs have been widely applied for the delivery of both hydrophobic and hydrophilic anti-cancer drugs including cisplatin [Citation31], paclitaxel [Citation32], doxorubicin (DOX) [Citation33] and methotrexate [Citation34] due to their improved release profile, anti-tumour efficacy and insignificant drug side effects. Passive targeting ability of GNPs through the enhanced permeability and retention (EPR) effect is another significant feature of GNPs, through which the nanoparticles accumulate and remain at the tumour site for a longer period of time, which causes the accumulation of anti-cancer drug at tumour site with high local concentration. Based on a recent study, compared to the commercial Cremophor/EtOH formulation, the paclitaxel-loaded GNPs presented 2.6 times higher bladder tumour tissue concentrations after intravesical administration into dogs [Citation35]. Also, X-ray diffraction analysis showed that the encapsulated paclitaxel was existing in an amorphous state, which has higher water solubility compared to the crystalline state [Citation35]. Based on experimental reports, the higher efficacy of anti-cancer drug-loaded GNPs compared to the free drug was revealed both in tumour-bearing animal models and in vitro. Young et al. [Citation24] developed biodegradable nanoparticles based on recombinant human gelatin (rHG) modified with alphatocopheryl succinate (TOS). Subsequently, 17-AAG (17-allylamino-17-demethoxygeldanamycin), a small molecular anticancer drug, has been encapsulated within rHG-TOS nanoparticles. They reported that, the tumour volume in the rats injected with 17-AAG/rHG-TOS nanoparticles was increased 15 times compared to the initial volume, whereas that of treated with free 17-AAG was increased 20 times, and also the 17-AAG-loaded nanoparticles were non-immunogenic [Citation24]. In another study, Lee et al. [Citation36] designed gelatin-doxorubicin (GD) and PEGylated gelatin-doxorubicin (PGD) nanoparticles and evaluated their feasibilities as an anti-cancer drug. They reported that compared to free DOX, both GD and PGD showed much lower cytotoxicity in vitro and in vivo at identical concentrations. However, PGD showed significant tumour growth inhibition than GD compared to the control and DOX treated group. Due to anti-tumour and anti-metastatic effects of these nanocomplexes, they showed that the obtained PGD nanoparticles are promising therapeutics for the cancer treatment. As cytotoxicity assessments of anti-tumour drug-loaded GNPs against various cancer cell lines, these particles exhibited enhanced anti-tumour activity compared to pure drug which might be associated with higher uptake of GNPs in cancer cells in an endocytosis manner. Jitender et al. [Citation37] synthesized noscapine-enveloped poly(ethylene glycol)-grafted gelatin nanoparticles (NOS-PEG-GN) and performed cytotoxicity analysis against MCF-7 cell line, in which the IC50 value of the obtained particles was equivalent to 20.8 μmol/L, which was significantly lower than noscapine-loaded gelatin nanoparticles (NOS-GN) (26.3 µmol/L) and free NOS (40.5 μmol/L). Furthermore, in vitro release kinetic results showed a first-order release of noscapine (85.1%) from gelatin nanoparticles with a release rate constant of 7.611 × 10−3. Besides, pharmacokinetic profiles of NOS-PEG-GN, NOS-GN, and NOS indicated that NOS-PEG-GN has a significantly higher half-life compared to NOS-GN and NOS as a result of stearic repulsion effect of PEG on nanoparticles [Citation37].
Cationic chitosan
Chitosan, a modified natural cationic copolymer comprising randomly distributed d-glucosamine and N-acetyl glucosamine, which varies in molecular chain length, sequence and composition. Chitosan possesses superior properties including biodegradability, biocompatibility, mucoadhesive properties and antibacterial activity which make chitosan an excellent candidate in tissue engineering, drug delivery and gene transfection. Chitosan is a pH-sensitive polymer (with pKa around 6.5), due to variation of charge density at the pH range of 6–6.5, which is useful for wide range of therapeutic applications. As mentioned before pKa value for chitosan is near neutral which causes soluble-insoluble transition at pH between 6 and 6.5, which is beneficial for biomedical applications () [Citation38]. At pH levels beneath the pKa, high charge density of chitosan results in polyelectrolyte formation, in contrast at neutral pH, the low charge density of chitosan eases the intracellular release of biomolecules and contributes to its low cytotoxicity. However, the low charge density results in poor solubility, accumulation and the low stability of chitosan-based formulations [Citation39]. The molecular weight (Mw) and degree of deacetylation (DD) [Citation40] are the major factors that determine the positive charge density and effect on cationic properties and cell-dependent transfection efficiency of chitosan [Citation41]. The cationic charge of chitosan facilitates the establishment of polyelectrolyte complexes with negatively charged surfaces, which improves the interaction with cell membranes and provides more effective transfection agents. However, a high degree of swelling at an aqueous media that results in rapid drug release and also low solubility at physiological pH are considered as major limitations of chitosan in therapeutic applications [Citation42]. Though, chemical modification of three reactive sites of chitosan, including one primary amine as well as two primary or secondary hydroxyl groups per glycosides’ unit [Citation43], is the common procedure to address the mentioned limitations.
Figure 2. Chitosan’s inherent cationic nature below its pKa. At lower pH about 6 chitosan’s amine groups are protonated giving poly-cationic behaviour to chitosan (Right). At pH about 6.5, chitosan’s amines are deprontonated and result in insoluble chitosan (left).
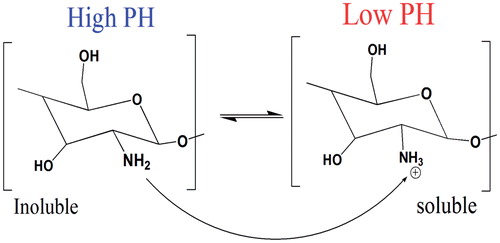
It is worth noting that, these modifications introduce the new properties without affecting on the fundamental properties of chitosan. Ionic gelation [Citation44], polyelectrolyte complex [Citation45] and emulsion cross-linking [Citation46] are three major methods for the preparation of chitosan nanoparticles. Ionic gelation method is an organic solvent-free, non-toxic, biocompatible and facile procedure which has been widely applied in different studies [Citation47]. This method is based on the ionic interaction between the negatively charged groups of a polyanion as a cross-linking agent and the positively charged primary amino groups of chitosan. Preparation of mono-disperse, low molecular weight (LMW) chitosan nanoparticles by ionic gelation applying sodium tripolyphosphate (TPP) as the cross-linking agent has been investigated [Citation48]. As reported, the narrow size distribution of nanoparticles was obtained by decreasing the concentration of acetic acid and lowering the temperature of reaction chamber [Citation48]. Emulsion cross-linking is another preparation method for chitosan nanoparticles in which a water-in-oil (w/o) emulsion is prepared by emulsifying the chitosan aqueous solution in the oil phase [Citation38]. This method requires a proper surfactant to stabilize the aqueous droplets. Afterwards, in order to hardening the droplets, a suitable cross-linking agent is required and at the last step obtained particles have to be filtered, washed repeatedly with alcohol and dried in vacuum [Citation49]. In this method, the amount of applied cross-linking agent and the speed of stirring are two major factors which determine the exact size of nanoparticles. However, this technique is a time consuming process and there might be residual of unreacted cross-linking agents. Bodnar et al. [Citation50] reported a facile technique to obtain chitosan nanoparticles, using covalent cross-linking through the amino groups of the chitosan chain with natural di- or tricarboxylic acids in an aqueous environment at the room temperature. As they reported, cross-linked chitosan nanoparticles were stable at aqueous environment at acidic, neutral, and mild alkaline conditions and the pH was the main determining factor of particle size.
Chitosan-based nanoparticles in drug delivery systems
Curcumin (Cur) is one of the most applied anti-cancer drugs, which suffers from a few drawbacks such as poor absorption, low aqueous solubility and quick metabolism, consequently its oral bioavailability and treatment efficacy are limited. Recently, Liu et al. [Citation51] developed a new Cur-loaded amphiphilic chitosan (CS) nanoparticles modified with hydrophobic acrylonitrile (AN) and hydrophilic arginine (Arg) for oral curcumin delivery. The Cur was encapsulated into AN–CS–Arg nanoparticles (AN–CS–Arg/Cur) (with average diameter of 218 nm) by a simple sonication technique which showed a significant enhancement of aqueous solubility of drug molecule. Based on results, AN–CS–Arg/Cur showed sustained release pattern in typical biological buffers as its in vitro release profile [Citation51]. Also, AN–CS–Arg/Cur nanoparticles exhibited stronger dose-dependent cytotoxicity against HT-29 cells compared to free Cur solution (). In addition, the in vivo pharmacokinetic studies in rats revealed that the AN–CS–Arg/Cur nanoparticles could significantly enhance the oral bioavailability of employed model drug.
Figure 3. Cytotoxicity effect of different concentrations of AN–CS–Arg/Cur nanoparticles in HT-29 cells. (Republished with permission of [DOVE Medical Press], from Ref. [Citation51] Copyright (2016); permission conveyed through Copyright Clearance Centre, Inc.).
![Figure 3. Cytotoxicity effect of different concentrations of AN–CS–Arg/Cur nanoparticles in HT-29 cells. (Republished with permission of [DOVE Medical Press], from Ref. [Citation51] Copyright (2016); permission conveyed through Copyright Clearance Centre, Inc.).](/cms/asset/9d5e3ea1-5a4a-4a96-bb4c-acf8f252c41e/ianb_a_1395344_f0003_c.jpg)
In another study, PEG-modified chitosan nanoparticles functionalized with transferrin (Tf) were produced for targeted delivery of paclitaxel (PTX) to cancer cells [Citation52]. This system was designed based on active targeting of cancer cells which overexpressed the transferrin receptors. PTX-loaded chitosan nanoparticles (PTX-NPs) were obtained by solvent evaporation technique, followed by PEGylation and Tf conjugation process carried out at the surface of PTX-NPs (PTX-NPs-PEG-Tf). It was revealed that, the uptake of NPs-PEG-Tf into cancer cells was significantly higher than non-targeted NPs, indicating the key role of transferrin in the receptor-mediated endocytosis [Citation52]. Compared to pure PTX, PTX-NPs-PEG-Tf exhibited enhanced cytotoxicity to human non-small cell lung cancer cell lines (HOP-62), higher intracellular uptake especially in nuclei, poor haemolytic toxicity and improved retention time in the lungs as well as blood circulation. In another experiment, propranolol-HCl-loaded chitosan nanoparticles were fabricated as transdermal drug delivery agents in order to enhance the systemic bioavailability of propranolol-HCl drug [Citation53]. The chitosan nanoparticles were obtained by ionic gelation method employing TPP as a cross-linking agent. As reported, the cationic nature of chitosan nanoparticles (zeta potential above ±30) provided a stable suspension and prevented the aggregation of particles. The obtained nanoparticles showed a sustain drug release behaviour and enhanced the systemic bioavailability and the therapeutic efficacy of propranolol-HCl. Karmakar et al. [Citation54] developed a targeted delivery system of metal-diketo complex, “bis(2,4- pentanedionato) copper(II)” to breast cancer cells (MCF-7) using chitosan nanoparticles modified with folic acid or her-2 specific peptide (KCCYSL) (). This metal-organic complex induced ROS and caused DNA damage as well as mitochondrial membrane depolarization and subsequently, activated the apoptosis as result of mitochondria rupture. It was also found that, those of cells that were expressing the specific receptor were significantly vulnerable to the drug, while, non-expressing isogenic cells remained almost unharmed and as toxicity studies, the mice treating with targeted drug, did not exhibit any sign of liver enzymes damage besides other histology changes [Citation54].
Figure 4. (A) Particle size determination of Chitosan nanoparticle conjugated with folic acid (CSF)/conjugated with peptide (CSP) by high resolution transmission electron microscope (HR-TEM), scanning electron microscope (FESEM), dynamic light scattering. (B) surface charge determination by zeta potential, (C) percentage of drug release by the CSF and CSP NPs. (Reprinted from Ref. [Citation54] Copyright 2016, with permission from Elsevier.)
![Figure 4. (A) Particle size determination of Chitosan nanoparticle conjugated with folic acid (CSF)/conjugated with peptide (CSP) by high resolution transmission electron microscope (HR-TEM), scanning electron microscope (FESEM), dynamic light scattering. (B) surface charge determination by zeta potential, (C) percentage of drug release by the CSF and CSP NPs. (Reprinted from Ref. [Citation54] Copyright 2016, with permission from Elsevier.)](/cms/asset/af8b939b-b00e-4d60-be40-7378cc9b5174/ianb_a_1395344_f0004_b.jpg)
Cationic cellulose
Cellulose, a linear chain of ringed β-1,4-d-glucan molecules, as the main constituent of the cell wall of plants, is the most plentiful polymer and also the most abundant organic material scattered out all over the world. Cellulose benefits various useful properties such as biodegradability, biocompatibility, and antibacterial properties emerging as an ideal choice for various therapeutic applications [Citation17,Citation18]. Also, it is good to know that, cellulose is poorly soluble in both polar and non-polar solvents due to robust intra- and intermolecular hydrogen bonding between cellulose molecules. To address this negative aspect, a few hydrophilic cellulose derivatives including hydroxyethyl cellulose (HEC) and hydroxypropyl cellulose (HPC), have been prepared which are FDA-approved and are commonly applied for various food and drug formulations [Citation55]. Cellulose can be obtained from a wide range of plants, and there is a broad range of cellulose particle types that are being studied for numerous commercial and biomedical applications. Acid hydrolysis [Citation56], enzymatic hydrolysis [Citation57] and electrospinning are the main preparation processes in order to obtain cellulose nanostructure. Acid hydrolysis applies chemical treatments such as acid hydrolysis. Rochas et al. [Citation58] obtained cellulose nanoparticles via sulfuric acid hydrolysis method. In this study, cotton linters (as a material source), was treated with 65% sulfuric acid during 30 min at four different temperatures, namely 45, 54, 63, and 72 °C. At the following, the obtained suspensions were washed by centrifugation, dialyzed to neutrality against distilled water, and ultrasonicated for 4 min and then the suspensions were filtered through 8 μm and 1 μm cellulose nitrate membranes and stocked with mixed bed resin in order to remove residual electrolytes [Citation58]. The obtained cellulose nanofibers had a length ranging 100–300 nm. Cellulose nanoparticles also obtained by enzymatic hydrolysis [Citation59], in which the carboxymethyl cellulose (CMC) (0.5 mg) was dissolved in water (100 ml) and then attuned to pH 5.6 using HCl. CMC solution was hydrolyzed with cellulase (cellulose enzyme) (5 mg) at 45 °C for 3 h. Hydrolysis of CMC was halted by thermal inactivation of cellulase. Finally, by mixing the prepared solution and similarly chitosan hydrolyzate solution, the chitosan-CMC nanoparticles were produced.
Cellulosic nanostructures in drug delivery
Either in traditional pharmacy or in advanced drug delivery systems, cellulosic structures have a defined position. At the following, we highlight several recent investigations, in which cellulose acted as drug delivery agents. Akbari et al. [Citation60] developed a new pH-sensitive bionanocomposite beads based on carboxymethyl cellulose (CMC) and ZnO nanoparticles as a carrier for propranolol hydrochloride (PPN) (). In this study, Fe3+ acted as a crosslinking agent to prepare ionic cross-linked bio-nanocomposite hydrogel beads. Also, sodium carboxymethyl cellulose, an anionic and water-soluble cellulose derivative that can form spherical gel beads, was employed as a matrix for ZnO nanoparticles. The swelling proportion of the CMC/ZnO bio-nanocomposite hydrogel beads was significantly higher than pure CMC hydrogel beads, indicating impact of different amounts of ZnO nanoparticles on the swelling and drug release profiles of bio-nanocomposite hydrogel beads. This complex exhibited a pH sensitive swelling behaviour with maximum water absorbing at pH 7.4. The CMC/ZnO bio-nanocomposite beads also showed a sustained and controlled release pattern of propranolol in the simulated gastro-intestinal conditions which might be an excellent choice for oral drug delivery system [Citation60]. In another study, pH sensitive theophylline-loaded cellulose nanocrystals (CNCs)-gelatin nanocomposite was designed, in which CNCs was applied as a reinforcing material in gelatin hydrogels for controlled drug delivery [Citation61]. Different amounts of CNCs (0, 5, 10, 15, 20, and 25%) were used to investigate the effects of CNCs on the dynamic and mechanical properties and swelling behaviour of gelatin-based hydrogels. Based on results, gelatin hydrogels reinforced with 15% CNC were to be a promising nominees for controlled drug delivery system.
Figure 5. SEM images of pure ZnO nanoparticles (A), pure CMC hydrogel beads (B) and CMC/ZnO-PPN bionanocomposite beads (C). (Reprinted from Ref. [Citation60] Copyright 2016, with permission from Elsevier.)
![Figure 5. SEM images of pure ZnO nanoparticles (A), pure CMC hydrogel beads (B) and CMC/ZnO-PPN bionanocomposite beads (C). (Reprinted from Ref. [Citation60] Copyright 2016, with permission from Elsevier.)](/cms/asset/dd5ece64-030d-4499-9ac8-f53504711902/ianb_a_1395344_f0005_c.jpg)
It was also revealed that, the drug loading efficiency and drug release properties of hydrogels were associated with the swelling ratio of hydrogels. As they reported, the highest drug loading and drug release rate and also the maximum swelling ratio were obtained by a gelatin hydrogel reinforced with 5% CNC at pH 3 [Citation61]. Sol et al. [Citation62] produced curcumin-loaded cellulose nanocrystals (CNCx) functionalized with cationic β-cyclodextrin (CD) (Cur–CD/CNCx). This system was designed to encapsulate and examine the anti-proliferative effect of curcumin on colorectal and prostatic cancer cell lines. In this complex, cellulose nanocrystals led to good mechanical strength, uniform nanorod shape and liquid crystalline behaviour. Cellulose nanocrystals were obtained by acid-hydrolysis of cotton fibres, followed by modification with cyclodextrins [Citation62]. The cell uptakes (PC-3, DU145 and HT-29 cells) of pure curcumin, Cur–CD and Cur–CD/CNCx were examined using confocal microscopy (). They reported low cellular accumulation with pure curcumin and Cur–CD complex after 4 h incubation, whereas Cur–CD/CNCx resulted in high accumulation of curcumin and showed strong anti-proliferative effect in all three cell lines. These results indicate the important role of CNCx in order to enhance the cell uptake.
Figure 6. Confocal microscopy images of PC-3, DU 145 and HT-29 cells without any treatment as control (CTL), Cur, Cur–CD and Cur–CD/CNCx (50 lM). Green fluorescence appears due to curcumin. (Reprinted from Ref. [Citation62] Copyright 2016, with permission from Elsevier.)
![Figure 6. Confocal microscopy images of PC-3, DU 145 and HT-29 cells without any treatment as control (CTL), Cur, Cur–CD and Cur–CD/CNCx (50 lM). Green fluorescence appears due to curcumin. (Reprinted from Ref. [Citation62] Copyright 2016, with permission from Elsevier.)](/cms/asset/b038405a-a3e6-4597-9f3c-ec219c6ee0e8/ianb_a_1395344_f0006_c.jpg)
Cationic dextran
Dextran (DEX) is a hydrophilic homopolysacharide of glucose, and also known as a natural analogue to PEG and possesses exceptional properties comprising excellent colloidal stability, biodegradability, bioavailability and hydrophilicity [Citation63,Citation64]. Based on various experiments, DEX-coated nanoparticles showed more colloidal stability behaviour compared to those of coated with PEG chains [Citation65] and also they show no non-specific protein binding activity [Citation66]. Interestingly, due to the ability of substitution of plentiful hydroxyl groups at the surface of DEX with various functional groups, DEX can be easily modified and conjugated to various molecules such as fluorescent dye, tetramethylrhodamine (TRITC-dextran) [Citation67] and different drugs including ibuprofen [Citation68] and platinum(IV) [Citation69]. The particle size and its distribution are two major factors that control vital properties of final dextran nanogels such as water absorption capacity and its kinetics. Abedini et al. [Citation70] prepared cross-linked dextran microgels (CDMs) via a water/oil (w/o) emulsification technique. They prepared aqueous phase (w) by dissolving 6.24 g of dextran (25% W/V) in 20 ml of 1.2 M NaOH solution, then homogenized the solution for 5 min at 3000 rpm. Dextran solution was then added to the oil phase (consisted of 245 ml of n-octane containing 2% W/V of the surfactant (Span® 80)) to provide 10% (v/v) w/o dispersion. As a crosslinking agent, 2.1 ml epichlorohydrin (ECH) was added to the emulsion and stirrer was set up at different values (400–900 rpm) at 40 °C which resulted in different-sized CDMs [Citation70]. Sun et al. [Citation71] reported the preparation of mucoadhesive chitosan/dextran (CS/DS) nanoparticles via ionic gelation method. CS/DS nanoparticles (100–200 nm in diameter) were prepared by following steps; (i) CS was dissolved in distilled water at concentration of 1–2 mg/mL, (ii) 3 ml of DS solution (0.2–0.6 mg/mL) was added into CS solution and stirred at 1000 rpm for 10 min. Based on reports, decreasing molecular weight of DS resulted in enhancing amount of adsorbed mucin and buccal cells on DS. The zeta potential of CS/DS nanoparticles ranged from 52 to 70 mV varying by concentration of CS and DS. Moreover, CS/DS nanoparticles showed five times higher mucoadhesive activity to buccal cells compared to control CS/TPP nanoparticles in ex vivo adhesion tests.
Dextran nanoparticles as drug carriers
As mentioned earlier, biodegradability, bioavailability and hydrophilicity are a few of many superior properties of DEX, which present DEX as a promising choice in drug delivery nanosystems. Wu et al. [Citation72] prepared pH/redox responsive succinic acid-modified dextran nanoparticles (DNPs) employing cystamine as cross-linker agent. The obtained particles were loaded with DOX and possessed negative charge at pH 7.4 and positive charge at pH 5. DNPs showed good serum stability and internalized in Hela cells quickly due to the charge reversible feature of the designed NPs. DNPs also exhibited biocompatible, biodegradable behaviour and pH/redox response to the acidic environment in tumour cells, which offer them as anticancer drug carriers for improvement of tumour intercellular uptake of DOX [Citation72]. Li et al. [Citation73] designed cisplatin-loaded dextran (cis/dex) nanoparticles modified with luteinizing hormone-releasing hormone (LHRH) and examined the inhibition effect of obtained nanoparticles on the 4T1 orthotopic mammary tumour metastasis model. LHRH was acting as a targeting ligand, which was attached to the overexpressed LHRH-receptors onto the surface of 4T1 breast cancer cells. Compared to the non-targeted cis/dex nanoparticles, LHRH-targeted cis/dex nanoparticles showed a high concentration of cisplatin in the injected primary tumour, enhanced cellular uptake and higher cytotoxicity against cancer cells. Furthermore, both targeted and non-targeted cis/dex nanoparticles reduced the systemic toxicity of cisplatin and enhanced the maximum tolerated dose of CDDP from 4 to 30 mg/kg [Citation73]. Recently, Saboktakin et al. [Citation74] prepared methotrexate (MTX)-encapsulated dextran sulphate (DS) and glycol chitosan (GCS) nanoparticles (DS-GCS) via a cross-linking method for the treatment of brain tumours. Based on results, DS led to improved physicochemical and biological properties of final particles. Also, it was demonstrated that increasing the cross-linking degree between DS–GCS chains, resulted in decreasing amount of drug released. The highest amount of drug release for MTX-loaded GCS–DS nanoparticles was observed in a high ionic strength media. In another study, DOX-loaded stearate-g-dextran (Dex-SA/DOX) micelles (<50 nm) was produced by an esterification reaction between the hydroxyl group of dextran and the carboxyl group of stearic acid (SA) through self-assembly technique [Citation75]. Dex-SA/DOX micelles showed sustained drug release behaviour which was affected by the molecular weight of Dex, the graft ratio of SA and the drug-loading content. Also, obtained micelles had superior internalization ability against A549 cells, which enhanced the DOX delivery into the tumour cells. Furthermore, Dex-SA/DOX micelles showed same cytotoxic behaviour against the drug-sensitive tumour cells as those of pure commercial DOX. Based on In vivo antitumor activity results, compared to pure DOX, Dex-SA/DOX micelles showed higher tumour growth inhibition activity and decreased the drug-related toxicities in the animal body [Citation75].
Synthetic cationic polymers
In recent decades, synthetic cationic polymers have shown great competence in medical applications including drug delivery [Citation76], gene therapy [Citation77] and tissue engineering [Citation78]. While, batch to batch variation of natural polymers is their major setback, this can be overcome by using the synthetic polymers benefiting their tailor-able properties, predictable lot-to-lot uniformity and ability to conjugate different bioactive molecules and functional groups to their surface. These properties regulate both the degradation properties of the polymer and its therapeutic potential in biological media. At the following passage, we discuss the most frequently employed synthetic cationic polymers in drug delivery field. We also provided a brief summary of their chemical structures and their various biomedical applications in .
Table 2. Summary of the chemical structures of the most widely applied synthetic cationic polymers and their related applications in biomedicine.
Poly(2-N,N-dimethylaminoethylmethacrylate) (PDMAEMA)
Poly(2-N,N-dimethylaminoethylmethacrylate) PDMAEMA is a water-soluble pH-sensitive cationic polymer, which has been widely applied in in medical applications. PDMAEMA possesses inherent cationic charge due to its tertiary amine groups at the surface of polymer that become partially protonated at the physiological solution that offers PDMAEMA as a promising candidate for gene delivery [Citation91] and drug delivery [Citation92]. Free radical polymerization is the common and facile procedure for preparation of PDMAEMA. Chakma et al. [Citation93] applied an alternative free radical bulk polymerization to synthesize PDMAEMA without using any solvent. Purified 2-N,N-dimethylaminoethylmethacrylate (DMAEMA) monomer (46.6 g) and 0.035 g AIBN (2,2′-azobisisobutyronitrile) as a initiator were placed into a 500 ml reactor and purged with nitrogen for 5 min and then the reactor was sealed and kept in a thermal bath at 50 °C for one week [Citation93]. The obtained polymer was in solid phase and the glass transition temperature (Tg) of PDMAEMA was measured to be 19.5 °C. In another study, PDMAEMA were synthesized by conventional solution polymerization of DMAEMA in toluene at 95 °C, initiated with 2,2-azobis(2-methylbutyronitrile) (AMBN) [Citation94]. Additionally, dodecanethiol (C12-SH) acted as a chain transfer agent to control the molar mass of produced polymer. Zhang et al. [Citation95] designed water-soluble and degradable PDMAEMA employing 5, 6-benzo-2-methylene-1,3-dioxepane (BMDO) to overcome the non-degradability issue of PDMAEMA. First, BDMO monomer (0.99 g) was dissolved in 0.1 ml DMAEMA in a pre-dried Schlenk tube under an argon atmosphere. Then, the poly (ethylene oxide) (PEO) and PEG6000 were used as free-radical axo-initiator and cytotoxicity reducing agent, respectively. The reaction mixture was located in a preheated oil bath at 70 °C for 24 h. Finally, the mixture was cooled in the ice bath and diluted with chloroform and precipitated in 200 ml of pentane. The obtained degradable co-polymer contained hydrolyzable ester linkages in the backbone and were soluble in water even with very high amounts of ester linkages. Moreover, the resulted polymer presented considerably less toxicity against L929 cell line [Citation95].
PDMAEMA in drug delivery
In the last decade, numerous investigations have been developed based on PDMAEMA as drug carrier agents. Due to its pH-sensitive properties, PDMAEMA plays a critical role in many multiple-responsive nanoparticles in order to achieve a smart drug delivery system [Citation96]. Recently, Tzankova et al. [Citation97] synthesized curcumin-loaded cationic micelles (∼130 nm) based on triblock copolymer PDMAEMA-poly (ɛ-caprolactone)-PDMAEMA and evaluated there in vitro cytotoxicity against two cell culture models. As they reported, unloaded cationic micelles did not show significant changes in cell viability and membrane integrity of human hepatoma cell line at the different concentrations, ranging from 10.0 to 80.0 mg/mL. Furthermore, the in vivo toxicity of both unloaded and loaded cationic micelles did not induce toxicity changes such as hematopoietic and liver tissue damages in male Wistar rats. Wang et al. [Citation98] fabricated CO2 stimulus-sensitive nanoparticles based on PDMAEMA-b-poly styrene (PDMAEMA-b-PS) by surfactant-free mini-emulsion reversible addition–fragmentation chain transfer (RAFT) polymerization method. The obtained particles were about 120 nm in diameter and their dispersion and aggregation behaviour were dependent on alternative bubbling of CO2 and N2. The drug release rate (accelerated or delayed) from these nanoparticles could be also adjusted by bubbling (or removing) of CO2. In this study PDMAEMA-b-PS nanoparticles have been used as Indomethacin (IND) delivery carriers. After bubbling of CO2 into intact nanoparticles (228 nm) solution, the size of nanoparticles decreased and the emulsion became transparent and once the CO2 was eliminated (presence of N2), the size of nanoparticles increased up to 224 nm. These phenomena could be imputed to the reversible interaction of PDMAEMA and CO2, in which the presence of CO2 results in protonation of tertiary amine groups of PDMAEMA along with formation of carbonic acid in water that enhances the solubility of particles in water and decreases their size ().
Figure 7. Schematic illustration of (A) Reactions of side chains of PDMAEMA on nanoparticles’ surface upon CO2 and N2 bubbling; (B) dispersion of nanoparticles in the presence/absence of CO2 (Reprinted from Ref. [Citation98] Copyright 2013, with permission from Elsevier.)
![Figure 7. Schematic illustration of (A) Reactions of side chains of PDMAEMA on nanoparticles’ surface upon CO2 and N2 bubbling; (B) dispersion of nanoparticles in the presence/absence of CO2 (Reprinted from Ref. [Citation98] Copyright 2013, with permission from Elsevier.)](/cms/asset/76cec08d-1c26-450e-b40b-ca27ab710b61/ianb_a_1395344_f0007_c.jpg)
He also reported that, presence of the CO2 significantly increased and improved the release rate of IND in PBS. These release behaviours can be associated with two reasons; (i) presence of CO2, causes enhancement in dispersion and interaction of nanoparticles with a buffer which leads in speeding up the release of drugs, (ii) thickness of shell might be decreased once PDMAEMA is protonated, which lets the drug out so easily through the shell. Generally, Such CO2 controlled drug delivery carriers, can be excellent choice to decrease the side effects caused by drug overdoses and accomplish the desired therapeutic effects of drugs [Citation98]. Xiong et al. [Citation99] reported the synthesis of DOX-loaded star polymer (DOX-NPs or DNP), including cyclodextrin polymer and pH-sensitive PDMAEMA as the core and PEG as the arm, in order to drug delivery investigation in vitro and in vivo. DOX-NPs had high encapsulation efficiency (77.1%), as well as sustain release of DOX in vitro and presented concentration-, time- and cell type-dependent cell uptake behaviour. Moreover, the obtained DOX-NPs exhibited meaningfully high cytotoxicity against HeLa and HepG2 cancer cells compared to free Dox. As in vivo experiment, cervical tumour-bearing mice were treated with DOX-NPs. Based on tumour size results, mice which were treated with DOX-NPs showed significant tumour growth inhibition and had smallest tumour size compared to control and free-DOX treated mice ().
Figure 8. Taken images of the tumours (A) removed from mice at the end point of the research. Tumour-growth changes (B) and body weight changes (C) of tumour-bearing BALB/c mice after tail vein injection of normal saline (square), free DOX (circle) and DOX-NPs (or DNP) (triangle) (at a DOX dose of 4 mg kg−1). The arrow indicates the beginning time points of the drug injection and the drug injection was repeated every three days. (Reprinted from Ref. [Citation99] Copyright 2014, with permission from Elsevier.)
![Figure 8. Taken images of the tumours (A) removed from mice at the end point of the research. Tumour-growth changes (B) and body weight changes (C) of tumour-bearing BALB/c mice after tail vein injection of normal saline (square), free DOX (circle) and DOX-NPs (or DNP) (triangle) (at a DOX dose of 4 mg kg−1). The arrow indicates the beginning time points of the drug injection and the drug injection was repeated every three days. (Reprinted from Ref. [Citation99] Copyright 2014, with permission from Elsevier.)](/cms/asset/45d41f7d-b03e-44c4-8e9b-e353f4640262/ianb_a_1395344_f0008_c.jpg)
Poly-l-lysine (PLL)
Poly-l-lysine (PLL) was one of the first cationic polymers examined in order to develop polyplexes with nucleic acids for receptor-mediated gene delivery [Citation100]. PLL is defined as a cationic homo-polymer of the amino acid l-lysine that is containing a high amount of primary amines which are partially protonated in physiological environment. This behaviour gives PLL ability to electrostatically interact with negatively charged biomolecules such as cell membrane or DNA that could be useful for drug delivery and gene delivery, respectively. Despite cytotoxic properties of PLL in high molecular weight and inclination to agglomeration and precipitation, the PLL, still is a beneficial and commonly used polymer, thus it is worth to overcome its drawbacks by different modification methods. One of the first improved approaches for synthesis of PLL was performed by Sela et al. [Citation101]. They converted L-lysine monomer into the cyclic N-carboxy-(N-benzyloxycarbonyl)-l-lysine anhydride, then the obtained N-carboxyanhydride monomers were polymerized by ring-opening polymerization method employing a primary amine initiator (). Based on the same principle, Kim et al. [Citation102] synthesized PLL firstly by converting (N-benzyloxycarbonyl)-l-lysine to N-carboxy-(N-benzyloxycarbonyl)-l-lysine. (N-benzyloxycarbonyl)-l-lysine (5 g) and 50 ml anhydrous dimethyl formamide were stirred under N2 atmosphere. Finally, 54.5 µl of ethylenediamine was added as initiator to the solution and stirred for 72 h to perform final polymerization process. In this study, PEG also was introduced to PLL in order to increase the stabilization of the cationic polymers by inhibiting the protein adsorption. Therefore, bifunctional PEG succinimidyl succinate (1.53 g) was added drop-wise to the final mixture and the copolymerization process continued for another 72 h [Citation102].
The preparation of PLL- alginate nanospheres was reported by Robinson et al. [Citation103]. To do so, 2 ml of aqueous calcium chloride was added drop-wise to 10 ml of aqueous sodium alginate and ultra-sonicated for 30 s and then stirred for 30 min. Then 2 ml of an aqueous PLL solution was added to the obtained mixture and stirred for an additional 30 min. The nanospheres were formed after keeping final suspension in environment temperature overnight and obtained by ultracentrifugation.
PLL as drug delivery agents
In recent years, PLL has attracted extensive attention of researchers towards novel drug delivery field because of its superior properties that mentioned earlier. Here we review a few of recent applications of PLL in drug delivery systems. Liu et al. designed a novel multi-block polymer as novel drug carriers based on poly(lactic acid)–poly(ethylene glycol)–poly(l-lysine) (PLA–PEG–PLL) to enhance the paclitaxel (PTX) delivery efficiency [Citation104]. In order to obtain a targeted drug delivery system, the PTX-loaded PLA–PEG–PLL nanoparticles (PNP) were functionalized with vascular endothelial growth factor (VEGF) antibody as target ligand (VPNP). It has been reported that, VPNP showed higher cytotoxicity effect against HepG2 cells compared to PNP and Taxol®. The antitumor efficacy in a murine model bearing H22, showed the same results, in which VPNP showed the best cell uptake indicating better interaction of functionalized particles with target cells and enhanced drug-uptake by tumour cells. In another attempt, synthesis of PLL-modified liposomes (100 nm) for retinal drug delivery was reported by Sasaki et al. [Citation105]. In this work, coumarin-6 was encapsulated within liposomes and applied as a model drug. It was found that PLL modification of liposomes significantly enhanced the coumarin-6 delivery to the mouse retina segments after eye drop administration. Based on results, 15,000–30,000 and 0.005% were the ideal molecular weight and concentration of PLL, respectively in which no cytotoxic and aggregation behaviour were reported in corneal or conjunctival cells [Citation105]. These results present PLL-modified liposomes as an excellent candidate for effective retinal drug delivery in eye drop formulations. Shi et al. [Citation106] fabricated insulin nanospheres (INS) and used cationic ɛ-poly-l-lysine (EPL) for surface modification and obtained core–shell structure (INS@EPL) in order to improve the pulmonary delivery of insulin. INS was produced by self-assembly of insulin into nanospheres by a novel thermal induced phase separation technique and INS@EPL was fabricated, employing a modified ionic shell cross-linking method using TPP. Based on reports, surface modification of nanospheres with EPL, resulted in a shift from negative (−16.3 ± 4.1) to positive (+19.1 ± 6.8) zeta potential. This cationic nature helps extensive electrostatically binding of EPL to the negatively charged heparin sulphate proteoglycans found on cell surfaces ().
Figure 10. Schematic illustration of shell cross-linked polycationic peptide guided lung deposition and alveoli permeation of self-assembled insulin nanospheres. (Reprinted from Ref. [Citation106] Copyright 2014, with permission from Elsevier.)
![Figure 10. Schematic illustration of shell cross-linked polycationic peptide guided lung deposition and alveoli permeation of self-assembled insulin nanospheres. (Reprinted from Ref. [Citation106] Copyright 2014, with permission from Elsevier.)](/cms/asset/59c42891-350b-4d75-9ca0-786a3f5ca099/ianb_a_1395344_f0010_c.jpg)
After pulmonary administration, both INS and INS@EPL were deposited in the middle part of right lung after 30 min (). After 90 min, the nanospheres progressively diffused into the lower part of the lungs and deposited at the entire lung lobes including alveoli. In contrary to INS@EPL, naked INS was cleared completely from lungs in 180 min. INS and INS@EPL showed 11.7% and 23.4% relative bioavailability, respectively which indicated the prolonged lung distribution and pharmacological effects of INS@EPL.
Figure 11. Representative images for lung deposition of INS (A) and INS@EPL (B) after intratracheal administration in rats. The images were merged from RGB and optical spectra. (Reprinted from Ref. [Citation106] Copyright 2014, with permission from Elsevier.)
![Figure 11. Representative images for lung deposition of INS (A) and INS@EPL (B) after intratracheal administration in rats. The images were merged from RGB and optical spectra. (Reprinted from Ref. [Citation106] Copyright 2014, with permission from Elsevier.)](/cms/asset/f6a42121-b983-4c3a-b300-7bfffb635119/ianb_a_1395344_f0011_c.jpg)
Poly(ethyleneeimine) (PEI)
Poly(ethyleneeimine) (PEI), a none-degradable polymer comprising primary, secondary and tertiary amino groups which is one of the most commonly employed cationic polymers in various industries. PEI can be obtained in two various structures: (i) linear PEI (LPEI) is a solid form of PEI and (ii) branched PEI (BPEI) is a highly viscous liquid at the room temperature. LPEI contains primary and secondary amino groups, while BPEI, in addition to primary and secondary groups, also possesses tertiary amino groups (with ratio of 1:2:1) which makes BPEI more stable and suitable transfection vector [Citation107]. It is worth noting that, at physiological conditions 25% of these amino groups are protonated which gives PEI high buffer capability that could be beneficial in endosomal escape mechanisms. Brissault et al. [Citation108] synthesized LPEI by hydrolysis and/or reduction of poly(2-ethyl-2-oxazoline) (PEtOXZ) (). A mixture of PEtOXZ (1 g), hydrochloric acid (11 ml, 37%) and 8 ml water was heated at 110 °C for 3 h. After solvent evaporation, the obtained mixture was dissolved in water and pH was adjusted to 9–10 by adding a proper amount of NaOH. Then the aqueous phase was vaporized, the residue washed with methylene chloride and consequently, the organic layer (Na2SO4) dried and vaporized. Based on results, yield of the polymer was 320 mg (71%) [Citation108]. In another study, Harpe et al. [Citation109] used acid-catalyzed polymerization of aziridine for the synthesis of BPEI (). First, aziridine (5 ml) were dissolved in 50 ml distilled water and 0.5 ml of 32% (w/v) hydrochloric acid (HCl) were added to the solution, then the flask was sealed and submerged in oil bath in 65 °C and kept for 24 h. NaOH was added to the mixture in order to neutralization process and then water was vaporized and the obtained polymer was dried under vacuum for 24 h [Citation109].
Figure 12. Synthesis of (A) LPEI by acid hydrolysis of poly(2-ethyl-2-oxazoline) (B) BPEI by acid-catalyzed polymerization of aziridine.
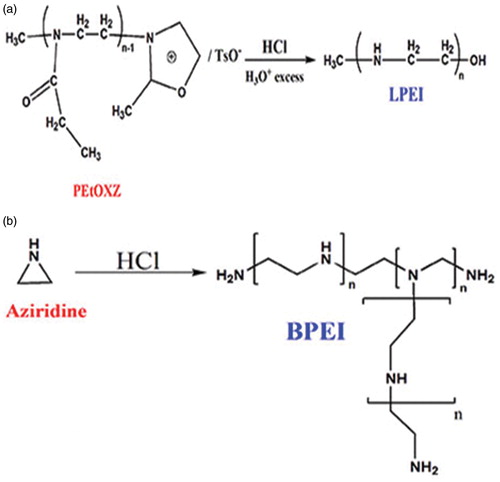
Despite unique properties of PEI, none-modified PEI possesses few considerable limitations for biomedical applications such as cytotoxicity (depending on molecular weight), low hemocompatibility and lack of biodegradability [Citation110]. In order to overcome such drawbacks, different strategies have been developed to fabricate modified PEI in order to enhance its biocompatibility and biodegradability. PEG is one of the excellent candidates for PEI modification which has been applied widely in recent investigations. Lately, Liu et al. [Citation111] synthesized PEI–PEG copolymer with the aim of co-delivery of DNA and DOX to tumour cells. He produced PEI–PEG copolymer via the reaction of N-N-hydroxysuccinimide (NHS) ester group of PEG with the amino groups of PEI (). Firstly, PEI was dissolved in 10 ml DMSO, then PEG derivatives with a maleimide and a ɯ-N-hydroxysuccinimidyl ester group (NHS–PEG–MAL) was added into the reaction solution under stirring. After that, in order to adjust the pH over 8, 20 µl TEA was added to the solution and stirred for 48 h at the room temperature. Then the mixture purified by dialysis against distilled water and lyophilized [Citation111]. Chitosan is another commonly used polymer for modification of PEI, which results in biodegradable and biocompatible PEI with low toxicity [Citation112]. Li et al. [Citation113] obtained chitosan-graft-polyethylenimine (CHI-g-PEI) copolymer with a biocleavable disulphide linkage between chitosan chains and PEI grafts. The produced copolymer showed cationic surface charge, stable behaviour and had no toxic effects on healthy cells.
Role of PEI in pharmaceutical applications
Here, we discuss the role of PEI in drug delivery applications and review several experiments that have been developed recently. Yu et al. [Citation114] reported the synthesis of a novel paclitaxel-delivery system based on PEI and poly(d,l-lactide-co-glycolide) (PLGA) copolymer which was electrostatically conjugated to herceptin that acted as a targeting ligand (eHER-PPNs) and employed as vectors targeting HER2-positive BT474 cells and HER2-negative MCF7 cells (). The obtained particles were about 280 nm in size and showed a neutral surface charge (1.00 ± 0.73 mV), which remained stable under physiological conditions. Based on MTT assay in BT474 cells, the biocompatibility of blank PPNs and eHER-PPNs displayed no significant cytotoxicity at the concentration about 50 μg/mL that explains their good biocompatibility. Based on results, the Paclitaxel-delivery efficiently was significantly higher (p < 0.001) than unmodified PPNs as well as free Paclitaxel. Moreover, eHER-PPNs presented enhanced cellular uptake efficiency (p < 0.001) in comparison with chemically-conjugated herceptin-bearing PEI/PLGA nanoparticles (cHER-PPNs) [Citation114].
Figure 14. Schematic illustration of the eHER-PPNs. (Reprinted from Ref. [Citation114] Copyright 2016, with permission from Elsevier.)
![Figure 14. Schematic illustration of the eHER-PPNs. (Reprinted from Ref. [Citation114] Copyright 2016, with permission from Elsevier.)](/cms/asset/0721927e-b4f4-4d5d-a71f-6071e490e00d/ianb_a_1395344_f0014_c.jpg)
Shi et al. [Citation115] reported the fabrication of PEI-derivatized C60 (C60-PEI) through cationic polymerization of aziridine on the surface of C60-NH2. Afterwards, the C60-PEI was modified with folic acid (FA) via an amide linker and consequently, docetaxel (DTX) was conjugated to the C60-PEI-FA (C60-PEI-FA/DTX) () in order to obtain a proper drug delivery system. This study revealed that, compared to the free DTX and C60-PEI/DTX, the C60-PEI-FA/DTX as a targeted delivery agents could efficiently cross the cell membranes that led to more apoptosis and showed higher antitumor efficacy in a cultured PC3 cells.
Figure 15. Characterization of C60-PEI-FA/DTX (A): AFM images of C60-PEI-FA/DTX; (B): TEM images of C60-PEI-FA/DTX. (Reprinted from Ref. [Citation115] Copyright 2012, with permission from Elsevier.)
![Figure 15. Characterization of C60-PEI-FA/DTX (A): AFM images of C60-PEI-FA/DTX; (B): TEM images of C60-PEI-FA/DTX. (Reprinted from Ref. [Citation115] Copyright 2012, with permission from Elsevier.)](/cms/asset/1eae04ea-ea09-45f8-84ab-0a20fce84ec9/ianb_a_1395344_f0015_c.jpg)
Based on in vivo experiments on tumour-bearing S180 mouse model, compared to the free DTX, C60-PEI-FA/DTX exhibited enhanced antitumor efficacy, protracted blood circulation and 7.5-fold higher DTX uptake of tumour without any significant toxic effects to healthy organs, which represents the C60-PEI-FA/DTX complex as promising choice for increasing treatment efficacy with minimal side effects. Yu et al. [Citation116] evaluated anticancer activity of four series of PEI-coated PLGA/lipid nanoparticles (PPLs). In this study, 800 Da-, 2 kDa- and 25 kDa-branched PEIs, and 25 kDa-linear PEI were designated as a polymeric coat for the nanoparticles and egg phosphatidylcholine (Egg PC) and paclitaxel (PTX) were applied as lipid and model drug, respectively. The obtained nanoparticles possessed hydrodynamic diameter ranging from 135.8 to 535.9 nm and zeta potentials between 13.5 and 45.4 mV. It was found that 5% of PEI and 20% of lipid content resulted in optimum formulation. In the following, the cytotoxicity evaluation of free PEIs and blank PPLs against A549 cell lines was performed after 24 h of incubation. As reported, the blank PPLs did not show any significant cytotoxic effects compared to free PEIs at the identical PEI concentration (10 μg/mL). In vitro experiments revealed that, 25 kDa-branched PEI coated PLGA/lipid nanoparticles (25k-bPPLs) showed the most cytotoxic effect in different cancer cell lines compared to those of uncoated drug-loaded PLGA/lipid nanoparticles (PLs). In the meantime, 25k-bPPLs had high cellular delivery efficiency without any tangible toxicity and also showed excellent colloidal stability at pH 7.4. These results offer 25k-bPPLs as a promising drug delivery carrier for cancer therapeutics [Citation116]. Lin et al. [Citation117] designed a cationic liposome–PEG–PEI complex (LPPC) in order to encapsulation and transdermal release of tamoxifen and evaluation of its efficacy in the skin penetration, drug accumulation and cancer therapy. Tamoxifen-loaded LPPC (LPPC/TAM) was 270 nm in diameter and the zeta-potential was measured to be 40 mV. LPPC/TAM significantly enhanced the cell death in all breast cancer cells, especially in oestrogen receptor-positive breast cancer cells. Furthermore, administration of LPPC/TAM by transdermal route prevented about 86% of tumour growth in mice bearing BT474 tumours without any local skin injury. This complex could be a useful as a transdermal drug consumption tool for the breast cancer therapy [Citation117].
Poly(amidoamine) (PAMAM)
Poly(amidoamine) (PAMAM) is one of the unique members of cationic polymers family that possesses excellent properties including water solubility, biodegradability, biocompatibility and low toxicity compared to other cationic polymers. Moreover, PAMAM could easily undergo further chemical functionalization due to numerous tertiary amino groups existing in its backbone, which comes in handy in order to side chain substituents for special purposes. PAMAM is commonly produced via aza-type Michael addition reaction of primary amines to bis-acrylamides [Citation118] which is a slow and time-consuming procedure. Sun et al. [Citation119] synthesized degradable PAMAM-based hydrogel through Michael-type addition between methylene bisacrylamide and amine mixture of 2-(2-aminoethoxy) ethanol and tyramine (TA) using horseradish peroxidase as cross-linker. The obtained hydrogel showed completely degradable behaviour within six to eight days with low cytotoxicity. Zintchenko et al. [Citation120] developed a novel method for synthesis of PAMAM by employing “Green” biocompatible earth alkaline metal salts such as CaCl2. As reported, the addition of CaCl2 to the reaction solution led to significant increase in reaction rate as compared to conventional methods without any variation in the polymer physico-chemical properties as well its biological activity [Citation120]. PAMAM can be obtained in both linear and branched (dendrimers) structures by manipulating the monomers (i.e. using ketals, acetals, carboxyl, amide and ester groups) employed for the polymerization, which can be useful in altering the polymer properties for desired applications (). Jain et al. [Citation121] fabricated family of cationic, pH-sensitive PAMAMs in which the acetal or ketal linkages were introduced into the backbone of the polymers to provide a degradable polymer in low pH. A significant hydrolysis rate has been seen as the pH decreased from 7.4 to 5.0 as the same condition existing in lysosomes. Moreover, depending on the structure of the monomer that applied to the synthesis of the each acid-degradable polymers, the hydrolysis half-life of the PAMAMs varied from 0.03 to 81.5 days at pH 5.0 and 6.0 to 161 days at pH 7.4 [Citation121].
Figure 16. Reaction process for the synthesis of linear PAMAM. R1, R2, R3 and R4 can be any alkyl residues in order to incorporate ketals, acetals, carboxyl, amide and ester groups.
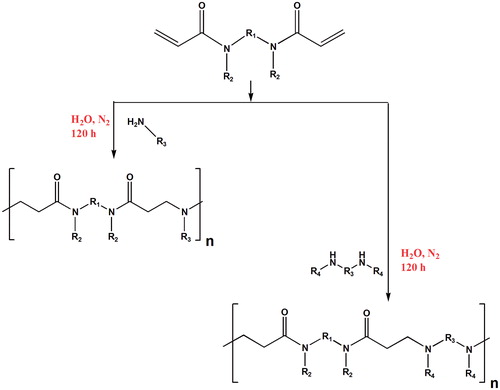
Lin et al. [Citation118] produced series of bio-reducible PAMAMs via Michael-type addition method containing multiple disulphide linkages in the main chain and oligoamines in the side chain (SS–PAOAs). The obtained linear PAMAMs revealed strong DNA condensation capability at low N/P (nitrogen to phosphate) ratios. Furthermore, the chemical structure of oligoamine side chains in the SS–PAOAs, showed a dissimilar effect on the transfection efficiency, toxicity profile and buffer capacity of PAMAM. Besides, increasing the alkyl spacer among the amino units in side chains from ethylene to propylene led to significant toxicity [Citation118].
Recent applications of PAMAM in drug delivery
In recent decade, numerous investigations based on PAMAM polymers, have been developed in drug delivery field. Hu et al. [Citation122] reported the synthesis of redox- and pH-responsive Dox-loaded PAMAM, in which PEG molecules were conjugated to the PAMAM via cleavable disulphide bonds. Based on the in vitro release study, DOX releasing from the obtained carriers were noticeably increased in reductive and acidic environment. Besides, cleavable PAMAMs were more effective in eliminating B16 and A549 tumour cells as compared to the non-cleavable counterparts. Singh et al. [Citation123] also used PAMAM dendrimers as drug carriers for combination therapy of an anti-hypertensive drug, ramipril (RAPL) and diuretic hydrochlorothiazide (HCTZ). They claimed that, the solubility of RAPL and HCTZ was reliant on pH of dendrimer solution and dendrimer concentration. Dendrimer-based formulations exhibited faster and complete dissolution compared to the pure RAPL or HCTZ. Moreover, dendrimer-based hybrid formulations showed stable behaviour at dark and refrigerated conditions up to five weeks [Citation123]. In another study, Qi et al. [Citation124] developed a DOX-loaded carboxymethyl chitosan-modified PAMAM dendrimers (PAMAM–DOX–CMCS) to achieve progressive drug targeting of tumours via pH-sensitive charge inversion. At the physiological conditions (pH 7.4) carboxymethyl chitosan acted as a shield for PAMAM dendrimers and resulted in negative charge at the surface of fabricated structure. At tumour site and under acidic conditions (pH 6.5), PAMAM recovered a positive charge via the removal of the carboxymethyl chitosan coating, which results in high intracellular uptake within tumour cells through electrostatic adsorptive endocytosis. Furthermore, compared to the free DOX, obtained dendrimers exhibited 16.45- and 9.27-fold higher cellular uptake efficiencies at pH 6.5 in MCF-7 and A549 cells, respectively. Also, intravenous administration of PAMAM–DOX–CMCS exhibited 1.50-fold greater antitumor activity and showed no significant systematic toxicity based on histological analysis compared to the free DOX [Citation124]. Recently, Dexamethasone (DEX)-conjugated PAMAM dendrimers were used for retinal sustain delivery of DEX [Citation125]. The results revealed that DEX–PAMAM conjugates were capable of enhancing the ocular permeability and ocular tissue levels of DEX following the subconjunctival injection, and the results were promising compared to the previous experiments which reported the DEX clearance from vitreous in 3 h. In another investigation, PAMAM-stabilized silver nanocomposites (DsAgNCs) were applied to encapsulate anticancer drug 5-fluorouracil (5-FU) to obtain synergism in cancer cells [Citation126]. Sustained release of 5-FU and synergistic anti-proliferative effect of 5-FU-loaded DsAgNCs (5-FU@DsAgNCs) were reported in A549 and MCF-7 cells with IC50 of 5 μg/mL and 1.5 μg/mL, respectively. Besides, nuclear and morphological changes, typical of apoptosis induction, were revealed (). Generally, they reported high apoptotic effects of 5-FU@DsAgNCs in MCF-7 compared to the A549 cancer cells [Citation126]. The fabricated complex was quite promising for simultaneous delivery of 5-FU and DsAgNPs to accomplish synergistic anticancer effects.
Figure 17. (A) Representative AO/EB (green/red dye) dual staining images of untreated, IC50 and 2 × IC50 5-FU@DsAgNC treated A549 and MCF-7 cells. EA and LA represent early apoptotic and late apoptotic cells, respectively. Scale bar: 100 μm. (B) FE-SEM images of untreated and IC50 5-FU@DsAgNC treated A549 and MCF-7 cells, respectively. (Reprinted from Ref. [127] with permission from The Royal Society of Chemistry.)
![Figure 17. (A) Representative AO/EB (green/red dye) dual staining images of untreated, IC50 and 2 × IC50 5-FU@DsAgNC treated A549 and MCF-7 cells. EA and LA represent early apoptotic and late apoptotic cells, respectively. Scale bar: 100 μm. (B) FE-SEM images of untreated and IC50 5-FU@DsAgNC treated A549 and MCF-7 cells, respectively. (Reprinted from Ref. [127] with permission from The Royal Society of Chemistry.)](/cms/asset/bf0861da-0cd2-4251-891c-b77d3683ca93/ianb_a_1395344_f0017_c.jpg)
Conclusions
Recent wide range experiments and clinical trials on nanoparticulate drug delivery systems proved the value of these systems for the biopharmaceutical industry benefiting their superior advantages over conventional drug delivery systems. The main purpose of this paper was to review some of the latest developments in drug delivery systems based on cationic polymers. In summary, we discussed various procedures that have been applied to synthesize natural and synthetic cationic polymers with favourable properties such as biocompatibility, biodegradability, low toxcicity, water solubility and proper interaction with targeting cell. We reviewed various strategies that can be applied in order to improve these properties, like introducing biodegradable bonds such as disulphide bonds into the backbone of cationic polymers to enhance their biodegradability and decrease their intrinsic toxcicity. We also brought up various surface modification methods associated with cationic polymers along with drug delivery agents in order to design a proper drug delivery system. We hope, ongoing research in multidisciplinary fields of cationic polymers will confidently results in further advanced designs, which is only attainable by comprehending the potentials and boundaries of conjugation, modification and functionalization chemistry.
Disclosure statement
No potential conflict of interest was reported by the authors.
References
- Shabestari Khiabani S, Farshbaf M, Akbarzadeh A, et al. Magnetic nanoparticles: preparation methods, applications in cancer diagnosis and cancer therapy. Artif Cells Nanomed Biotechnol. 2017;45:6–17.
- Farshbaf Davaran M, Rahimi S, Annabi F, et al. Carbon quantum dots: recent progresses on synthesis, surface modification and applications. Artif Cells Nanomed Biotechnol. Forthcoming. [cited 2017 Sep 21]. doi: 10.1080/21691401.2017.1377725.
- Panahi Y, Farshbaf M, Mohammadhosseini M, et al. Recent advances on liposomal nanoparticles: synthesis, characterization and biomedical applications. Artif Cells Nanomed Biotechnol. 2017;45:788–799.
- Panahi Mohammadhosseini Y, Nejati-Koshki MK, Abadi AJN, et al. Preparation, surface properties, and therapeutic applications of gold nanoparticles in biomedicine. Drug Res (Stuttg). 2017;67:77–87.
- Afsharzadeh Hashemi M, Mokhtarzadeh M, Abnous AK, et al. Recent advances in co-delivery systems based on polymeric nanoparticle for cancer treatment. Artif Cells Nanomed Biotechnol. Forthcoming. [cited 2017 Sep 28]. doi: 10.1080/21691401.2017.1376675.
- Peer D, Karp JM, Hong S, et al. Nanocarriers as an emerging platform for cancer therapy. Nature Nanotech. 2007;2:751–760.
- Strebhardt K, Ullrich A. Paul Ehrlich's magic bullet concept: 100 years of progress. Nat Rev Cancer. 2008;8:473–480.
- Brigger I, Dubernet C, Couvreur P. Nanoparticles in cancer therapy and diagnosis. Adv Drug Deliv Rev. 2002;54:631–651.
- Zhang L, Gu F, Chan J, et al. Nanoparticles in medicine: therapeutic applications and developments. Clin Pharmacol Ther. 2008;83:761–769.
- Hiwale P, Lampis S, Conti G, et al. In vitro release of lysozyme from gelatin microspheres: effect of cross-linking agents and thermoreversible gel as suspending medium. Biomacromolecules. 2011;12:3186–3193.
- Lee W-F, Lee S-C. Effect of gelatin on the drug release behaviors for the organic hybrid gels based on N-isopropylacrylamide and gelatin. J Mater Sci: Mater Med. 2007;18:1089–1096.
- Obata Y, Nishino T, Kushibiki T, et al. HSP47 siRNA conjugated with cationized gelatin microspheres suppresses peritoneal fibrosis in mice. Acta Biomater. 2012;8:2688–2696.
- Chen J-P, Su C-H. Surface modification of electrospun PLLA nanofibers by plasma treatment and cationized gelatin immobilization for cartilage tissue engineering. Acta Biomater. 2011;7:234–243.
- Lee J, Yun K-S, Choi CS, et al. T cell-specific siRNA delivery using antibody-conjugated chitosan nanoparticles. Bioconjugate Chem. 2012;23:1174–1180.
- Shi W, Nie D, Jin G, et al. BDNF blended chitosan scaffolds for human umbilical cord MSC transplants in traumatic brain injury therapy. Biomaterials. 2012;33:3119–3126.
- Wang H, Zhao P, Liang X, et al. Folate-PEG coated cationic modified chitosan–cholesterol liposomes for tumor-targeted drug delivery. Biomaterials. 2010;31:4129–4138.
- Song Y, Zhang L, Gan W, et al. Self-assembled micelles based on hydrophobically modified quaternized cellulose for drug delivery. Colloids Surf B: Biointerfaces. 2011;83:313–320.
- Xu F, Ping Y, Ma J, et al. Comb-shaped copolymers composed of hydroxypropyl cellulose backbones and cationic poly ((2-dimethyl amino) ethyl methacrylate) side chains for gene delivery. Bioconjugate Chem. 2009;20:1449–1458.
- Young SA, HUNG SC, Anderson WH, et al. Effects of cationic hydroxyethyl cellulose on glucose metabolism and obesity in a diet‐induced obesity mouse model. J Diabetes. 2012;4:85–94.
- Cohen JL, Schubert S, Wich PR, et al. Acid-degradable cationic dextran particles for the delivery of siRNA therapeutics. Bioconjugate Chem. 2011;22:1056–1065.
- Kalaska B, Sokolowska E, Kaminski K, et al. Cationic derivative of dextran reverses anticoagulant activity of unfractionated heparin in animal models of arterial and venous thrombosis. Eur J Pharmacol. 2012;686:81–89.
- KaminskiPłonka K, Ciejka M, Szczubiałka J, et al. Cationic derivatives of dextran and hydroxypropylcellulose as novel potential heparin antagonists. J Med Chem. 2011;54:6586–6596.
- Konat Zorzi G, Contreras-Ruiz L, Párraga JE, et al. Expression of MUC5AC in ocular surface epithelial cells using cationized gelatin nanoparticles. Mol Pharmaceutics. 2011;8:1783–1788.
- Won Y-W, Yoon S-M, Sonn CH, et al. Nano self-assembly of recombinant human gelatin conjugated with α-tocopheryl succinate for Hsp90 inhibitor, 17-AAG, delivery. ACS Nano. 2011;5:3839–3848.
- Li C, Yuan W, Jiang H, et al. PCL film surfaces conjugated with P (DMAEMA)/gelatin complexes for improving cell immobilization and gene transfection. Bioconjugate Chem. 2011;22:1842–1851.
- MorimotoChono K, Kosai S, Seki TT, et al. Design of cationic microspheres based on aminated gelatin for controlled release of peptide and protein drugs. Drug Deliv. 2008;15:113–117.
- Park C, Vo CL-N, Kang T, et al. New method and characterization of self-assembled gelatin–oleic nanoparticles using a desolvation method via carbodiimide/N-hydroxysuccinimide (EDC/NHS) reaction. Eur J Pharm Biopharm. 2015;89:365–373.
- Choubey J, Bajpai AK. Investigation on magnetically controlled delivery of doxorubicin from superparamagnetic nanocarriers of gelatin crosslinked with genipin. J Mater Sci: Mater Med. 2010;21:1573–1586.
- Patra S, Basak P, Tibarewala D. Synthesis of gelatin nano/submicron particles by binary nonsolvent aided coacervation (BNAC) method. Mater Sci Eng C. 2016;59:310–318.
- Gupta AK, Gupta M, Yarwood SJ, et al. Effect of cellular uptake of gelatin nanoparticles on adhesion, morphology and cytoskeleton organisation of human fibroblasts. J Control Release. 2004;95:197–207.
- Dixit N, Vaibhav K, Pandey RS, et al. Improved cisplatin delivery in cervical cancer cells by utilizing folate-grafted non-aggregated gelatin nanoparticles. Biomed Pharmacother. 2015;69:1–10.
- Yeh TK, Lu Z, Wientjes MG, et al. Formulating paclitaxel in nanoparticles alters its disposition. Pharm Res. 2005;22:867–874.
- Li W-M, Su C-W, Chen Y-W, et al. In situ DOX-calcium phosphate mineralized CPT-amphiphilic gelatin nanoparticle for intracellular controlled sequential release of multiple drugs. Acta Biomater. 2015;15:191–199.
- Cascone MG, Lazzeri L, Carmignani C, et al. Gelatin nanoparticles produced by a simple W/O emulsion as delivery system for methotrexate. J Mater Sci: Mater Med. 2002;13:523–526.
- Lu Z, Yeh T-K, Tsai M, et al. Paclitaxel-loaded gelatin nanoparticles for intravesical bladder cancer therapy. Clin Cancer Res. 2004;10:7677–7684.
- Young Lee G, Park K, Nam JH, et al. Anti-tumor and anti-metastatic effects of gelatin-doxorubicin and PEGylated gelatin-doxorubicin nanoparticles in SCC7 bearing mice. J Drug Target. 2006;14:707–716.
- Madan J, Dhiman N, Sardana S, et al. Long-circulating poly(ethylene glycol)-grafted gelatin nanoparticles customized for intracellular delivery of noscapine: preparation, in-vitro characterization, structure elucidation, pharmacokinetics, and cytotoxicity analyses. Anti-Cancer Drugs. 2011;22:543–555.
- Dash M, Chiellini F, Ottenbrite R, et al. Chitosan—A versatile semi-synthetic polymer in biomedical applications. Prog Polym Sci. 2011;36:981–1014.
- Mukhopadhyay P, Chakraborty S, Bhattacharya S, et al. pH-sensitive chitosan/alginate core-shell nanoparticles for efficient and safe oral insulin delivery. Int J Biol Macromol 2015;72:640–648.
- Weecharangsan W, Opanasopit P, Ngawhirunpat T, et al. Evaluation of chitosan salts as non-viral gene vectors in CHO-K1 cells. Int J Pharm. 2008;348:161–168.
- Thibault M, Astolfi M, Tran-Khanh N, et al. Excess polycation mediates efficient chitosan-based gene transfer by promoting lysosomal release of the polyplexes. Biomaterials. 2011;32:4639–4646.
- Oh I-h, Min HS, Li L, et al. Cancer cell-specific photoactivity of pheophorbide a–glycol chitosan nanoparticles for photodynamic therapy in tumor-bearing mice. Biomaterials. 2013;34:6454–6463.
- Belalia R, Grelier S, Benaissa M, et al. New bioactive biomaterials based on quaternized chitosan. J Agric Food Chem. 2008;56:1582–1588.
- Dong Y, Ng WK, Shen S, et al. Scalable ionic gelation synthesis of chitosan nanoparticles for drug delivery in static mixers. Carbohydr Polym. 2013;94:940–945.
- Birch NP, Schiffman JD. Characterization of self-assembled polyelectrolyte complex nanoparticles formed from chitosan and pectin. Langmuir. 2014;30:3441–3447.
- Zhou Z, Jiang F, Lee T-C, et al. Two-step preparation of nano-scaled magnetic chitosan particles using Triton X-100 reversed-phase water-in-oil microemulsion system. J Alloys Compounds. 2013;581:843–848.
- Agnihotri SA, Mallikarjuna NN, Aminabhavi TM. Recent advances on chitosan-based micro-and nanoparticles in drug delivery. J Control Release. 2004;100:5–28.
- Fan W, Yan W, Xu Z, et al. Formation mechanism of monodisperse, low molecular weight chitosan nanoparticles by ionic gelation technique. Colloids Surf B: Biointerfaces. 2012;90:21–27.
- Akbuǧa J, Durmaz G. Preparation and evaluation of cross-linked chitosan microspheres containing furosemide. Int J Pharm. 1994;111:217–222.
- Bodnar M, Hartmann JF, Borbely J. Preparation and characterization of chitosan-based nanoparticles. Biomacromolecules. 2005;6:2521–2527.
- Raja MA, Shah Z, Arif M, et al. Self-assembled nanoparticles based on amphiphilic chitosan derivative and arginine for oral curcumin delivery. Int J Nanomed. 2016;11:4397–4412.
- Nag M, Gajbhiye V, Kesharwani P, et al. Transferrin functionalized chitosan-PEG nanoparticles for targeted delivery of paclitaxel to cancer cells. Colloids Surf B Biointerfaces. 2016;148:363–370.
- Al-Kassas R, Wen J, Cheng AE, et al. Transdermal delivery of propranolol hydrochloride through chitosan nanoparticles dispersed in mucoadhesive gel. Carbohydr Polym. 2016;153:176–186.
- Pramanik A, Laha D, Dash SK, et al. An in-vivo study for targeted delivery of copper-organic complex to breast cancer using chitosan polymer nanoparticles. Mater Sci Eng C Mater Biol Appl. 2016;68:327–337.
- Gonzalez H, Hwang SJ, Davis M. New class of polymers for the delivery of macromolecular therapeutics. Bioconjugate Chem. 1999;10:1068–1074.
- Duran N, Paula Lemes A, B Seabra A. Review of cellulose nanocrystals patents: preparation, composites and general applications. Recent Pat Nanotechnol. 2012;6:16–28.
- Peng B, Dhar N, Liu H, et al. Chemistry and applications of nanocrystalline cellulose and its derivatives: a nanotechnology perspective. Can J Chem Eng. 2011;89:1191–1206.
- Elazzouzi-Hafraoui S, Nishiyama Y, Putaux J-L, et al. The shape and size distribution of crystalline nanoparticles prepared by acid hydrolysis of native cellulose. Biomacromolecules. 2008;9:57–65.
- Ichikawa S, Iwamoto S, Watanabe J. Formation of biocompatible nanoparticles by self-assembly of enzymatic hydrolysates of chitosan and carboxymethyl cellulose. Biosci Biotechnol Biochem. 2005;69:1637–1642.
- Zare-Akbari Z, Farhadnejad H, Furughi-Nia B, et al. PH-sensitive bionanocomposite hydrogel beads based on caborboxymethyl cellulose/ZnO nanoparticle as drug carrier. Int J Biol Macromol. 2016;93:1317–1327.
- Ooi SY, Ahmad I, Amin MCIM. Cellulose nanocrystals extracted from rice husks as a reinforcing material in gelatin hydrogels for use in controlled drug delivery systems. Ind Crops Prod. 2016;93:227–234.
- Ndong Ntoutoume GMA, Granet R, Mbakidi JP, et al. Development of curcumin–cyclodextrin/cellulose nanocrystals complexes: new anticancer drug delivery systems. Bioorg Med Chem Lett. 2016;26:941–945.
- Alibolandi M, Mohammadi M, Taghdisi SM, et al. Fabrication of aptamer decorated dextran coated nano-graphene oxide for targeted drug delivery. Carbohydr Polym. 2017;155:218–229.
- Zhang Z, Chen X, Chen L, et al. Intracellular pH-sensitive PEG-block-acetalated-dextrans as efficient drug delivery platforms. ACS Appl Mater Interfaces. 2013;5:10760–10766.
- Goodwin AP, Tabakman S, Welsher MK, et al. Phospholipid − dextran with a single coupling point: a useful amphiphile for functionalization of nanomaterials. J Am Chem Soc. 2008;131:289–296.
- Lei H, Xie M, Zhao Y, et al. Chitosan/sodium alginate modificated graphene oxide-based nanocomposite as a carrier for drug delivery. Ceram Int. 2016;42:17798–17805.
- Tran NBNN, Knorr F, Mak WC, et al. Gradient-dependent release of the model drug TRITC-dextran from FITC-labeled BSA hydrogel nanocarriers in the hair follicles of porcine ear skin. Eur J Pharm Biopharm. 2017;116:12–16.
- Abioye AO, Chi GT, Simone E, et al. Real-time monitoring of the mechanism of ibuprofen-cationic dextran crystanule formation using crystallization process informatics system (CryPRINS). Int J Pharm. 2016;509:264–278.
- He S, Zhou D, Kuang H, et al. Dextran-platinum(IV) conjugate as drug carrier for triggered drug release. J Control Release. 2015;213:e96.
- Salimi-Kenari H, Imani M, Nodehi A, Abedini H. An engineering approach to design of dextran microgels size fabricated by water/oil emulsification. J Microencapsul. 2016;33:511–523.
- Suh JW, Lee JS, Ko S, et al. Preparation and characterization of mucoadhesive buccal nanoparticles using chitosan and dextran sulfate. J Agric Food Chem. 2016;64:5384–5388.
- Wu L, Zhang L, Shi G, et al. Zwitterionic pH/redox nanoparticles based on dextran as drug carriers for enhancing tumor intercellular uptake of doxorubicin. Mater Sci Eng C. 2016;61:278–285.
- Li M, Tang Z, Zhang Y, et al. Targeted delivery of cisplatin by LHRH-peptide conjugated dextran nanoparticles suppresses breast cancer growth and metastasis. Acta Biomater. 2015;18:132–143.
- Saboktakin MR, Tabatabaie RM, Maharramov A, et al. Synthesis and characterization of pH-dependent glycol chitosan and dextran sulfate nanoparticles for effective brain cancer treatment. Int J Biol Macromol. 2011;49:747–751.
- Du Y-Z, Weng Q, Yuan H, et al. Synthesis and antitumor activity of stearate-g-dextran micelles for intracellular doxorubicin delivery. ACS Nano. 2010;4:6894–6902.
- Wang S, Xu T, Yang Y, et al. Colloidal stability of silk fibroin nanoparticles coated with cationic polymer for effective drug delivery. ACS Appl Mater Interfaces. 2015;7:21254–21262.
- Thomas TJ, Tajmir-Riahi HA, Thomas T. Polyamine–DNA interactions and development of gene delivery vehicles. Amino Acids. 2016;48:2423–2431.
- Walmsley GG, McArdle A, Tevlin R, et al. Nanotechnology in bone tissue engineering. Nanomed Nanotechnol Biol Med. 2015;11:1253–1263.
- Liu Y, Cao X, Luo M, et al. Self-assembled micellar nanoparticles of a novel star copolymer for thermo and pH dual-responsive drug release. J Colloid Interface Sci. 2009;329:244–252.
- Han S, Cheng Q, Wu Y, et al. Effects of hydrophobic core components in amphiphilic PDMAEMA nanoparticles on siRNA delivery. Biomaterials. 2015;48:45–55.
- Skarmoutsou A, Lolas G, Charitidis CA, et al. Nanomechanical properties of hybrid coatings for bone tissue engineering. J Mech Behav Biomed Mater. 2013;25:48–62.
- Liu T, Xue W, Ke B, et al. Star-shaped cyclodextrin-poly (l-lysine) derivative co-delivering docetaxel and MMP-9 siRNA plasmid in cancer therapy. Biomaterials. 2014;35:3865–3872.
- Lam J, Clark EC, Fong EL, et al. Data describing the swelling behavior and cytocompatibility of biodegradable polyelectrolyte hydrogels incorporating poly (L-lysine) for applications in cartilage tissue engineering. Data Brief. 2016;7:614–619.
- Patra D, Sleem F. A new method for pH triggered curcumin release by applying poly (l-lysine) mediated nanoparticle-congregation. Anal Chim Acta. 2013;795:60–68.
- Chen L, Ji F, Bao Y, et al. Biocompatible cationic pullulan-g-desoxycholic acid-g-PEI micelles used to co-deliver drug and gene for cancer therapy. Mater Sci Eng C. 2017;70:418–429.
- Chen M, Tang Y, Wang T, et al. Enhanced gene delivery of low molecular weight PEI by flower-like ZnO microparticles. Mater Sci Eng C. 2016;69:1367–1372.
- Dong S, Han L, Cai M, et al. Synthesis of electroactive tetraaniline grafted polyethylenimine for tissue engineering, in. IOP Conf Ser: Mater Sci Eng. 2015;87:012072.
- Jeong H, Lee ES, Jung G, et al. Bioreducible-cationic poly(amido amine)s for enhanced gene delivery and osteogenic differentiation of tonsil-derived mesenchymal stem cells. J Biomed Nanotechnol. 2016;12:1023–1034.
- Rossi E, Gerges I, Tocchio A, et al. Biologically and mechanically driven design of an RGD-mimetic macroporous foam for adipose tissue engineering applications. Biomaterials. 2016;104:65–77.
- Cheng L, Hu Q, Cheng L, et al. Construction and evaluation of PAMAM-DOX conjugates with superior tumor recognition and intracellular acid-triggered drug release properties. Colloids Surf B Biointerfaces. 2015;136:37–45.
- Ko NR, Cheong J, Noronha A, et al. Reductively-sheddable cationic nanocarriers for dual chemotherapy and gene therapy with enhanced release. Colloids Surf B: Biointerfaces. 2015;126:178–187.
- Dragan ES, Loghin DFA, Cocarta A-I, et al. Multi-stimuli-responsive semi-IPN cryogels with native and anionic potato starch entrapped in poly (N, N-dimethylaminoethyl methacrylate) matrix and their potential in drug delivery. React Funct Polym. 2016;105:66–77.
- Du R, Feng X, Chakma A. Poly(N,N-dimethylaminoethyl methacrylate)/polysulfone composite membranes for gas separations. J Membrane Sci. 2006;279:76–85.
- Wang M, Oh JK, Dykstra TE, et al. Surface modification of CdSe and CdSe/ZnS semiconductor nanocrystals with poly (N, N-dimethylaminoethyl methacrylate). Macromolecules. 2006;39:3664–3672.
- Zhang Y, Zheng M, Kissel T, et al. Design and biophysical characterization of bioresponsive degradable poly(dimethylaminoethyl methacrylate) based polymers for in vitro DNA transfection. Biomacromolecules. 2012;13:313–322.
- Salehi R, Hamishehkar H, Eskandani M, et al. Development of dual responsive nanocomposite for simultaneous delivery of anticancer drugs. J Drug Target. 2014;22:327–342.
- Tzankova V, Gorinova C, Kondeva-Burdina M, et al. In vitro and in vivo toxicity evaluation of cationic PDMAEMA-PCL-PDMAEMA micelles as a carrier of curcumin. Food Chem Toxicol. 2016;97:1–10.
- Wang X, Jiang G, Wei Z, et al. Preparation and drug release property of CO2 stimulus-sensitive poly(N, N-dimethylaminoethyl methacrylate)-b-polystyrene nanoparticles. Eur Polym J. 2013;49:3165–3170.
- Xiong Q, Zhang M, Zhang Z, et al. Anti-tumor drug delivery system based on cyclodextrin-containing pH-responsive star polymer: In vitro and in vivo evaluation. Int J Pharm. 2014;474:232–240.
- Zauner W, Ogris M, Wagner E. Polylysine-based transfection systems utilizing receptor-mediated delivery. Adv Drug Deliv Rev. 1998;30:97–113.
- Sela M, Arnon R, Jacobson I. Synthesis of poly‐L‐lysine and poly‐L‐lysyl albumin via ϵ, N‐trifluoroacetyl‐α, N‐carboxy‐L‐lysine anhydride. Biopolymers. 1963;1:517–525.
- Ahn C-H, Chae SY, Bae YH, et al. Synthesis of biodegradable multi-block copolymers of poly(l-lysine) and poly(ethylene glycol) as a non-viral gene carrier. J Control Release. 2004;97:567–574.
- De S, Robinson D. Polymer relationships during preparation of chitosan–alginate and poly-l-lysine–alginate nanospheres. J Control Release. 2003;89:101–112.
- Liu Y, Liu C, Li M, et al. Polymer–polymer conjugation to fabricate multi-block polymer as novel drug carriers: poly (lactic acid)–poly (ethylene glycol)–poly (L-lysine) to enhance paclitaxel target delivery. J Biomed Nanotechnol. 2014;10:948–958.
- Sasaki H, Karasawa K, Hironaka K, et al. Retinal drug delivery using eyedrop preparations of poly-l-lysine-modified liposomes. Eur J Pharm Biopharm. 2013;83:364–369.
- Shi K, Liu Y, Ke L, et al. Epsilon-poly-l-lysine guided improving pulmonary delivery of supramolecular self-assembled insulin nanospheres. Int J Biol Macromol. 2015;72:1441–1450.
- Louis M, Dutoit S, Denoux Y, et al. Intraperitoneal linear polyethylenimine (L-PEI)-mediated gene delivery to ovarian carcinoma nodes in mice. Cancer Gene Ther. 2006;13:367–374.
- Jones GD, Langsjoen A, Neumann SMMC, et al. The polymerization of ethylenimine. J Org Chem. 1944;09:125–147.
- von Harpe A, Petersen H, Li Y, et al. Characterization of commercially available and synthesized polyethylenimines for gene delivery. J Control Release. 2000;69:309–322.
- Forrest ML, Koerber JT, Pack DW. A degradable polyethylenimine derivative with low toxicity for highly efficient gene delivery. Bioconjugate Chem. 2003;14:934–940.
- Liu C, Liu F, Feng L, et al. The targeted co-delivery of DNA and doxorubicin to tumor cells via multifunctional PEI-PEG based nanoparticles. Biomaterials. 2013;34:2547–2564.
- Kim TH, Kim SI, Akaike T, et al. Synergistic effect of poly (ethylenimine) on the transfection efficiency of galactosylated chitosan/DNA complexes. J Control Release. 2005;105:354–366.
- Li Z-T, Guo J, Zhang J-S, et al. Chitosan-graft-polyethylenimine with improved properties as a potential gene vector. Carbohydr Polym. 2010;80:254–259.
- Yu K, Zhao J, Zhang Z, et al. Enhanced delivery of Paclitaxel using electrostatically-conjugated Herceptin-bearing PEI/PLGA nanoparticles against HER-positive breast cancer cells. Int J Pharm. 2016;497:78–87.
- Shi J, Zhang H, Wang L, et al. PEI-derivatized fullerene drug delivery using folate as a homing device targeting to tumor. Biomaterials. 2013;34:251–261.
- Yu K, Zhao J, Yu C, et al. Role of four different kinds of polyethylenimines (PEIs) in preparation of polymeric lipid nanoparticles and their anticancer activity study. J Cancer. 2016;7:872–882.
- Lin Y-L, Chen C-H, Wu H-Y, et al. Inhibition of breast cancer with transdermal tamoxifen-encapsulated lipoplex. J Nanobiotechnol. 2016;14:11.
- Lin C, Blaauboer C-J, Timoneda MM, et al. Bioreducible poly(amido amine)s with oligoamine side chains: Synthesis, characterization, and structural effects on gene delivery. J Control Release. 2008;126:166–174.
- Sun Y, Deng Z, Tian Y, et al. Horseradish peroxidase‐mediated in situ forming hydrogels from degradable tyramine‐based poly (amido amine) s. J Appl Polym Sci. 2013;127:40–48.
- Zintchenko A, van der Aa LJ, Engbersen JF. Improved synthesis strategy of poly (amidoamine) s for biomedical applications: catalysis by green biocompatible earth alkaline metal salts. Macromol Rapid Commun. 2011;32:321–325.
- Jain R, Standley SM, Frechet JM. Synthesis and degradation of pH-sensitive linear poly (amidoamine) s. Macromolecules. 2007;40:452–457.
- Hu W, Cheng L, Cheng L, et al. Redox and pH-responsive poly (amidoamine) dendrimer–poly (ethylene glycol) conjugates with disulfide linkages for efficient intracellular drug release. Coll Surf B: Biointerfaces. 2014;123:254–263.
- Singh M, Pooja K, Kulhari DH, et al. Poly (amidoamine) dendrimer-mediated hybrid formulation for combination therapy of ramipril and hydrochlorothiazide. Eur J Pharm Sci. 2016;96:84–92.
- Qi X, Qin J, Fan Y, et al. Carboxymethyl chitosan-modified polyamidoamine dendrimer enables progressive drug targeting of tumors via pH-sensitive charge inversion. J Biomed Nanotechnol. 2016;12:667–678.
- Yavuz B, Bozdağ Pehlivan S, Sümer Bolu B, et al. Dexamethasone - PAMAM dendrimer conjugates for retinal delivery: preparation, characterization and in vivo evaluation. J Pharm Pharmacol. 2016;68:1010–1020.
- Matai I, Sachdev A, Gopinath P. Multicomponent 5-fluorouracil loaded PAMAM stabilized-silver nanocomposites synergistically induce apoptosis in human cancer cells. Biomater Sci. 2015;3:457–468.