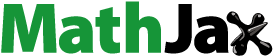
Abstract
This project was designed to extract anti-hyperlipidaemic activity of simvastatin for the longer duration of time and the solubility enhancement of this BCS class II drug was also sought by preparing nanostructured lipid carrier (NLC). The method for NLC preparation involved melt-emulsification followed by ultrasonication. The developed formulation was optimized by 3-level, 3-factor Box–Behnken design. The independent variables were % lipid mixture, % surfactant and sonication time (minutes). The responses selected were particle size, PDI and entrapment efficiency. The optimized formulation had particle size 114.62 ± 5.65, PDI 0.210 ± 0.034 and entrapment efficiency 85.20 ± 7.98%. The optimized formulation was further characterized by TEM and SEM. Spherical shape and nano size distribution of the optimized formulation were confirmed by SEM and TEM, respectively. The DSC of optimized formulation hints for the loss of crystalline nature of drug whereas the FT-IR spectra reveal that there is no interaction between drug and excipients when incorporated in NLC. The drug release from the NLC was biphasic (burst release was followed by sustained release). The kinetic of drug release followed Fickian diffusion with Korsmeyer–Peppas model. When the antihyperlipidaemic activity of SMV-NLC was compared with SMV-suspension, it was found that SMV-NLC significantly decreased the total cholesterol and triglyceride level by 2.77 and threefolds, respectively.
Graphical Abstract

Introduction
Hyperlipidaemia is a metabolic disease characterized by abnormally increased blood lipids, predominantly, cholesterol-enriched lipoprotein. Overall, raised cholesterol is estimated to cause 2.6 million deaths (4.5% of total) and 29.7 million disability-adjusted life years (DALYS) worldwide. It is a major cause of disease burden in both developed and developing countries. Around the world, hyperlipidaemia is also a major risk factor for Ischaemic heart disease and stroke [Citation1].
Drugs such as statins have been successfully used to reduce blood cholesterol level. They act by inhibiting 3-hydroxy-3-methylglutaryl-CoA reductase (HMG-CoA reductase), a rate-limiting step in cholesterol biosynthesis [Citation2]. Simvastatin (SMV) is a prodrug that belongs to BCS class II category (low solubility and high permeability). The combined effect of poor water solubility of SMV and extensive hepatic metabolism by CYP3A negatively affect the dissolution of SMV inside the gastrointestinal tract which ultimately results in low bioavailability (<5%) [Citation3,Citation4]. For the bioavailability enhancement, parenteral routes are preferred because SMV is a prodrug, hence oral administration is the only preferred route for this drug and hepatic metabolism cannot be avoided. On the other hand, the solubility of SMV can be enhanced by incorporating it into a nano-carrier [Citation5].
Among various nano-carrier systems, the polymeric nanoparticulate system is more preferred for peptide or protein delivery, whereas the lipidic nanoparticulate system is better for the oral delivery of poorly soluble drugs [Citation6]. Among lipidic nanoparticulate systems, nanoemulsion, self-nanoemulsifying drug-delivery system (SNEDDS), solid–lipid nanoparticles (SLNs) and nanostructured lipid carriers (NLCs) are frequently practiced drug carriers in recent time. However, nanoemulsion and SNEDDS do not have sufficient sustained release property. Contrary to it, SLNs and NLCs are better choices as far as the sustained release is concerned [Citation7]. The very less half-life of SMV (2 h) makes it a good candidate for sustained release. Although SLNs and NLCs both fulfill the criteria for sustained action, still NLC is selected over SLN for this research because it has been found that NLCs overcome the constraints of SLN, i.e. reduced drug loading, premature drug release and drug leakage. NLCs are lipid-based nanoparticles with a solid–lipid matrix incorporated with liquid lipid encapsulating the lipophilic drug and thus preventing it from degradation and enhancing the oral bioavailability of poorly water-soluble drugs of BCS-II class. NLCs are reported to have high loading capacity as the lipophilic drug can easily partition solid, liquid lipids and emulsifiers [Citation8].
In the present study, NLC of SMV was prepared using melt-emulsification followed by sonication. Because SMV is a substrate for P-gp efflux, therefore incorporation of a P-gp efflux inhibitor into the NLC is also the requirement of this study. Poloxamer-188 is used as a surfactant in this research because it has the P-gp efflux inhibiting property [Citation9]. This study focused on solubility enhancement of SMV and includes the development, optimization, characterization, in vitro release and antihyperlipidaemic activity of SMV-NLC in Wistar rats.
Materials and methods
Materials
Simvastatin was a gift from Ranbaxy Laboratories (India). Glyceryl monostearate (GMS), Labrafil M 1944 CS, Poloxamer188 and Mannitol were purchased from Alpha Chemicals (India), Gattefosse (France), BASF (India), CDH (India), respectively. The HPLC grade acetonitrile, phosphoric acid and methanol were purchased from Loba Chemie, India. Cholesterol was procured from Spectrochem Pvt. Ltd. (Mumbai, India). Cogent diagnostic reagent kit was procured from Span Cognet diagnostic kit (Gujarat, India). All other chemicals and reagents used were of analytical grade.
Methods
HPLC
Reverse phase HPLC analytical method was used for the quantitative analysis of drug using a Shimadzu LC-2010CHT series chromatographic system, Japan. It consisted of a Model LC-10 ATVP binary pump, C18 stainless steel column (250 mm ×4.6 mm, 5 μm) and it was also equipped with SPD-10 AVP UV–Visible spectrophotometric detection system [Citation6]. The data obtained were interpreted by VP software. The mobile phase used for RP-HPLC was a mixture of acetonitrile and 0.1% phosphoric acid (85:15, v/v) at pH 4 [Citation3]. The temperature of the column (5 μm RP C18, Lichrocart) was set at 40 °C while flow rate of 1.5 mL/min was maintained throughout the process. Samples were filtered prior to injection into the system. The injection volume was 20 μL and the wavelength for detection was fixed at 238 nm.
Selection of excipients
Selection of liquid lipids
The solubility of simvastatin in various liquid lipids (oleic acid, Labrafil M 1944 CS, eucalyptus oil, isopropyl myristate(IPM), Labrafil M 2125 CS, coconut oil, sunflower oil, olive oil), was determined by dissolving an excess amount of drug in small centrifuge tube (capacity 2 mL) containing 2 mL oil. This system was stirred continuously on a vortex mixer for 72 h at room temperature (25 °C). When equilibrium was achieved, the system was centrifuged at 5000 rpm for 15 min to get a clear supernatant. This supernatant was dissolved in methanol and amount of drug dissolved in that oil was determined by using UV spectrophotometer (UV 1700, Shimadzu, Japan) at 238 nm. The experiment was conducted in triplicate and results were represented as mean value (mg/ml) ± SD [Citation10].
Selection of solid lipids
The solubility determination of Simvastatin in various solid lipids (stearic acid, Precirol ATO 5, Compritol 888 ATO, glyceryl monostearate) was executed by adding simvastatin in increments of 1 mg until it failed to be dissolved further in the molten solid lipid (which were heated at 5 °C above its melting point). The amount of solid lipids required to solubilize simvastatin was calculated. The experiment was conducted in triplicate and results were represented as mean value (mg/g) ± SD [Citation11].
Miscibility of solid and liquid lipid
For the miscibility study, the mixture of solid and liquid lipid was heated on a magnetic stirrer (Remi instruments Ltd., Mumbai, India). The set up was maintained at 70 °C ± 2 °C and 200 rpm for 1 h. Once the temperature of the mixture was cooled down, it was smeared on a Whatman filter paper. Thereafter, the presence of oil droplets was visually assessed. If oil droplets are found on filter paper that means the solid and liquid lipid are not miscible with each other [Citation12].
Selection of surfactants
Surfactant for the preparation of NLC was selected based on its capacity to emulsify lipid mixture. 3 ml of methylene chloride was taken and 100 mg solid–liquid binary lipid was dissolved in it. Using a magnetic stirrer the solution was then added to 10 mL of 5% surfactant solution. Milli-Q water was then used to dilute the obtained suspension by 10-fold. UV-Vis spectrophotometer was used to observe the percentage transmittance of the sample. The sample exhibiting maximum transmittance was selected for the development of SMV-NLC [Citation9].
Preparation of simvastatin-loaded nanostructured lipid carrier
The simvastatin-loaded nanostructured lipid carrier system (NLC) was prepared by melt emulsification and ultrasonication technique. Briefly, an appropriate amount of glyceryl monostearate (GMS) as a solid lipid and Labrafil M1944 CS as liquid lipid was heated to 10 °C above the melting point of GMS [Citation13]. Simvastatin was dissolved by stirring in molten lipid to form a uniform and clear oil phase. An aqueous phase was prepared by dissolving Poloxamer188 in double distilled water (sufficient to produce 10 mL of preparation) and heated to the same temperature as the oil phase [Citation14]. The hot aqueous phase was added dropwise to the oil phase with continuous stirring at 600 rpm for 10 min. The hot lipid emulsion was sonicated by probe sonicator (Hielscher, UP-50H, Germany). Simvastatin hot nanoemulsion was cooled to room temperature and then was finally stored.
Adding 0.1 N HCl to the NLC changed its pH to 1.20 and produced an aggregate of NLC. The aggregate of NLC thus formed was centrifuged at 20 °C for 30 min at 30,000 rpm to obtain NLC pellet. 10 mL distilled water was mixed with 2% mannitol and the obtained pellet was suspended in it [Citation15].
Optimization of NLC by Box–Behnken statistical design
To evaluate the interactive effects of independent variables on dependent variables and in order to get optimized formulation with the best size, entrapment efficiency and PDI, a three-factor three-level Box–Behnken design (version 9.0.2.0, Stat Ease Inc., Minneapolis, MN) was used [Citation16]. The independent variables selected were % lipid mix, % surfactant and sonication time (in minutes) as shown in . From initial trials, it was observed that above said variables were found to be key factors affecting the particle size, entrapment efficiency, and PDI of NLC [Citation17]. This response surface methodology required 17 experimental runs as shown in .
Table 1. Coded independent and dependent variables with their lower and higher limit.
Table 2. Box–Behnken experimental design of SMV-loaded NLC and evaluated response parameters (n = 3).
The polynomial equation generated for the experimental design is given as follows:
(1)
(1)
Where Y is the response of the dependent variables associated with each factor level, b0 is intercept; b1 to b3 are linear coefficients, b12 to b23 are linear coefficients, b11 to b33 are regression coefficients of the respective variables, X1 to X3 are coded levels of independent variables, Xi, X1X2 and Xi 2 (i = 1, 2 or 3) represent the linear effect [Citation18]
Characterization of optimized simvastatin-loaded NLC
Particle size and polydispersity index
Particle size analysis is the most important thing when we claim the preparation of nanostructured lipid carrier. Dynamic light scattering (Malvern Zeta sizer, Nano ZS, UK) was employed for the particle size analysis [Citation19]. But before the analysis of particle size, the samples were diluted 50 times with double distilled water [Citation20].
Drug entrapment efficiency
The entrapment efficiency was calculated by determining the non-encapsulated simvastatin concentration in the supernatant. 10 ml volume of NLC dispersion was taken in a centrifuge tube and was then centrifuged at 12,000 rpm for 30 min at 15 °C by using Remi C-24 cooling centrifuge, Mumbai, India. The supernatant was taken out and the amount of drug in the supernatant was determined spectrophotometrically at λmax of 238 nm after suitable dilution with methanol [Citation21]. Entrapment efficiency was calculated by the following equation.
(2)
(2)
WTotal and WFree are the weight of drug added in the formulation and analyzed weight of drug in supernatant, respectively.
Differential scanning calorimetry (DSC)
The developed formulation containing simvastatin was characterized for crystallinity by DSC (Perkin Elmer, Pyris 6 DSC, USA) [Citation22]. For this purpose, two aluminium pans were taken, one pan was kept empty as a reference and the other pan containing approximately 2 mg of lyophilized formulation. The temperature of the instrument was maintained between 30 °C and 250 °C and the flow of nitrogen gas was maintained at a flow rate of 60 ml//min. The data obtained were interpreted using Pyris (Perkin-Elmer, USA) software [Citation23].
Transmission electron microscopy (TEM)
The particle size of optimized SMV-NLC was further confirmed using TEM (CM 200, Philips Briarcliff Manor, NY) [Citation24]. For the sample preparation, uranyl acetate was used as the negative staining agent. 10 mL of 50 times diluted optimized SMV-NLC was dropped over copper grid coated with carbon film. After drying it was observed under TEM [Citation25].
Scanning electron microscopy (SEM)
The developed formulation shape and surface characteristics were determined by SEM (model Zeiss 224 EVO 50 VP, Germany). The sample was coated with the gold–palladium alloy in a sputter coating unit after mounting sample on a round brass stub with the help of double-sided adhesive tape. In order to obtain good quality, SEM images uniform coating is done on the sample by sputtering approximately for 2 min. The slide was then observed under SEM [Citation26].
Fourier transform infrared spectroscopy (FT-IR)
The IR absorption spectra of the developed formulation were determined using Alpha FTIR spectrometer (Bruker Optik GmbH, Ettlingen, Germany) equipped with DTGS detector. The sample was grounded with KBR to form a pellet. The pellet thus formed was kept in a disc for analysis. It was scanned at the rate of 4 mm/s at a resolution of 2 cm−1 over a wave number range from 4000 to 500 cm−1 [Citation27].
In vitro drug release studies
In vitro release of drug from NLC was evaluated by the dialysis bag diffusion technique with slight modification [Citation28]. The release studies of simvastatin from NLC were performed in phosphate buffer (pH 6.8). The aqueous nanoparticle dispersion of 2 mL was placed in a dialysis bag (pre-activated) and sealed at both the ends. The dialysis bag (MW 12000–14000 Da, Hi Media, Mumbai) was immersed in the dissolution vessel containing 200 mL of dissolution medium (9:1 ratio of phosphate buffer 6.8 and ethanol) which was stirred at 100 rpm and maintained at 37 ± 2 °C. The receptor compartment was closed in order to prevent the evaporation of dissolution medium. An aliquot of 2 mL samples was withdrawn at a predetermined time interval of 0.5, 1.0, 2.0, 4.0, 6.0, 8.0, 12.0 and 24.0 h and the same volume was replaced by fresh dissolution medium. The samples were further diluted with methanol, filtered by 0.22 μm filter membrane and then analyzed using the RP-HPLC method. All the experiments were repeated three times and the graph was plotted between % cumulative drugs released and time (hours).
The % cumulative drug release (CDR) was calculated by the following formula:
(3)
(3)
Where, conc. is a concentration of drug calculated from regression equation of calibration plot. DF is dilution factor; VM is the volume of media and A is the amount of drug taken for in vitro study.
To determine the drug release pattern from the optimized NLC formulation, data obtained from the in vitro release study were fitted to various release models like zero-order model, first-order model, Higuchi model, Korsmeyer–Peppas model, Hixson–Crowell model and Weibull model [Citation29]. Model giving R2 nearer to 1 was selected as a best-fit model for the drug release.
Pharmacodynamic study
Animals procurement and maintenance
For conducting the pharmacodynamic study, Female albino Wistar rats (200–250 g) of either sex were taken. Approval for in vivo study was obtained from Jamia Hamdard, Institutional Animal Ethics Committee (173/CPCSEA, 28 January 2000; Approval no. 1126) and their guidelines were followed throughout the course of in vivo studies. Animals were kept in standard laboratory conditions maintained at a temperature of 25 ± 2 °C and relative humidity 55% ± 5% RH. The animals were housed in polypropylene cages with free access to standard diet and water. The animal dose for conducting in vivo studies was calculated on the basis of body surface area ratio of a rat to that of a human being [Citation30,Citation31]. For the present study, 10 mg/kg dose of simvastatin was fed to each animal of the group.
Antihyperlipidaemic activity
The animals were divided into three groups, Group-I (Control), Group-II (API suspension) and Group-III (Optimized NLC formulation). Hyperlipidaemia was induced in all the groups by administration of high-fat diet consisting of 200 mg of cholesterol suspended in 2 mL coconut oil for 14 days using oral feeding needle [Citation32]. After 2 h of administration of high-fat diet, group II and III were administered API suspension and optimized NLC formulation, respectively. After 0, 4, 7 and 14 days of the treatment, the rats were anesthetized using diethyl ether and blood samples were withdrawn from a tail vein in a microcentrifuge tube. From the blood, the serum is separated by incubating for 15 min at 37 °C, then centrifuged at 5000 rpm for 30 min. The withdrawn serum samples were analyzed in vitro for total cholesterol (TC), high-density lipoprotein (HDL) and triglycerides (TG) by using Span Cognet diagnostic kit [Citation33].
Results and discussion
Screening of components and preparation of simvastatin-loaded NLC
The solubility of SMV in different solid lipids and liquid lipids is graphically represented in . Among solid lipids, maximum solubility of SMV was found in Glyceryl monostearate (33.40 ± 0.98 mg/gm), whereas among liquid lipids, Labrafil M1944 CS (51.80 ± 1.08 mg/ml) dissolved the maximum drug. The selection of Glyceryl monostearate and Labrafil M1944CS as a binary lipid phase was further justified based on the miscibility study.
Clarity of NLC formulations produced by various surfactants is shown in in terms of % transmittance. Among all these surfactants, Poloxamer 188 produced the NLC formulation with maximum % transmittance. This can be attributed to the maximum particle size reduction potential of Poloxamer 188. The selection of Poloxamer 188 as a surfactant is advantageous too, as this surfactant is known for its P-glycoprotein efflux inhibition potential [Citation34]. As simvastatin is a P-glycoprotein substrate, Poloxamer 188 may have additional benefits of P-gp inhibition and hence better drug uptake. SMV-NLC was successfully produced by melt emulsification and ultrasonication technique [Citation35].
Table 3. Transmittance of NLC formulations produced by different surfactants (n = 3).
Optimization and selection of simvastatin-loaded NLC by Box–Behnken statistical design
Seventeen experimental runs, recommended by Box–Behnken statistical design were executed to optimize the SMV-NLC formulation. In all the 17 formulations, the minimum and maximum values for responses such as particle size, PDI and entrapment efficiency were varied from 89.45 ± 1.10 to 142.73 ± 2.30 nm, 0.172 ± 0.028 to 0.300 ± 0.036 and 72.60 ± 4.98 to 95.15 ± 6.88%, respectively (). These data generated equations were based on the quadratic polynomial model. The regression analysis results for all the three responses (Y1, Y2 and Y3) are summarized in . The ‘‘Predicted R2’’ values for all the three dependent variables were found to be in reasonable agreement with the ‘‘Adjusted R2’’ values.
Table 4. ANOVA Results for responses Y1, Y2 and Y3 obtained from experimental design response.
Three-dimensional plots obtained for all the three responses are shown in . These plots help in understanding the interaction effects of independent variables on the responses. depicts a quantitative comparison of observed values of the responses with that of the predicted values. These graphs help in ensuring whether the experimentally obtained data of dependent variables are reproducible or not.
Figure 2. 3 D-response surface plots showing effect of independent variables on (A) Particle size, (B) PDI and (C) entrapment efficiency.

Effect of independent variables on particle size
Particle size is one of the most important parameters for the characterization of NLCS, as the particle size in nano range leads to the increased interfacial surface area and hence incurs better drug absorption.
(4)
(4)
According to EquationEquation (4)(4)
(4) and , individually, % lipid mix positively affected the particle size and increase in lipid-mix concentration in the formulation enhanced the particle size drastically (). The individual effect of % surfactant and sonication time on particle size was negative (EquationEquation (4)
(4)
(4) . With the increase in surfactant concentration and sonication time, particle size decreased gradually (). Combined effect of lipid-mix and surfactant (X1X2) on particle size was negative with a magnitude of 0.55, suggesting that surfactant effect on particle size was slightly dominant over the effect of lipid-mix.
Effect of independent variables on PDI
Interpretation of EquationEquation (5)(5)
(5) led to the generation of a quadratic model with the lack of fit value of 0.38.
(5)
(5)
According to EquationEquation (5)(5)
(5) and , PDI of the NLC formulations was most sensitive to sonication time followed by % lipid-mix and % surfactant. Our result reveals that increase in the concentration of lipid has increasing effect on PDI, while surfactant and sonication time first reduce the PDI up to some extent then again raise the PDI of NLC formulations. However, the overall effect of sonication time was an increase in PDI and that of surfactant is a slight decrease in PDI.
Effect of independent variables on entrapment efficiency
Entrapment efficiency is a very important pharmacoeconomic parameter to be assessed, as higher entrapment efficiency helps in reducing the wastage of drug during the fabrication of NLC.
(6)
(6)
Model F value (2096.69) for EquationEquation (6)(6)
(6) indicates that model was significant (“Prob > F’’ less than 0.0500). In addition to this, the ‘‘lack of fit” was also found to be not significant (p = .368) suggesting the good model fitting. The ‘‘predicted R-squared’’ of .9967 was in reasonable agreement with the ‘‘adjusted R-squared’’ of .9992 (). From the EquationEquation (6)
(6)
(6) and , it is clear that individually, increase in % lipid-mix resulted in the increment of entrapment efficiency (). This can be attributed to the availability of more coating or matrix material to hold the drug. In general, individually, % surfactant and sonication time had a negative impact on the entrapment efficiency (EquationEquation (6)
(6)
(6) . But precisely, increase in surfactant concentration resulted in a decrease in entrapment efficiency up to a certain level, afterwards an increase in entrapment efficiency was observed ().
To get the optimized best formulation, constraints applied on the particle size, PDI and entrapment efficiency were “minimize”, “minimize” and “maximize”, respectively (). Based on the applied constraints, the numerical optimization section of Box–Behnken design predicted the recipe for the SMV-NLC preparation as 2.5% lipid-mix, 1% surfactant and 5 min sonication time. The corresponding particle size, PDI and entrapment efficiency were also predicted as 115.57, 0.209 and 85.38, respectively ().
Table 5. Optimized levels of independent variables and their corresponding predicted and observed responses.
The optimized SMV-NLC was prepared with the optimized level of component and process variables mentioned in . The experimental values of particle size, PDI and entrapment efficiency of the optimized SMV-NLC were found to be 114.62 ± 5.65, 0.210 ± 0.034 and 85.20 ± 7.98, respectively ().
Characterization of optimized SMV-NLC
Particle size and polydispersity index (PDI)
The average particle size and PDI of the optimized SMV-NLC were found to be 114.62 ± 5.65 nm and 0.210 ± 0.034 (n = 3) respectively, as shown in the representative particle size distribution graph (). The PDI of optimized SMV-NLC was below 0.3, indicating that the developed lipidic nanoparticulate system was uniform.
Drug entrapment efficiency
The entrapment efficiency of optimized SMV-NLC was found to be 85.20 ± 7.98%. High entrapment of drug is possible due to unique binary lipid matrix of NLC.
Transmission electron microscopy and scanning electron microscopy
TEM image of optimized SMV-NLC () further approved the particle size already determined by dynamic light scattering technique (). Moreover, the particles of optimized SMV-NLC were found to be circular in the 2-dimensional image captured by TEM (). The 3-dimensional SEM image () of the optimized SMV-NLC confirmed the morphology of lipidic nanoparticles as almost spherical.
Differential scanning calorimetry (DSC) analysis
DSC thermograms of SMV, physical mixture (GMS and poloxamer 188) and SMV-NLC are shown in . The pure drug, SMV displayed a sharp endothermic peak at 139.975 °C indicating the crystalline nature of SMV. A physical mixture of Poloxamer188 and GMS showed the melting point at 51.881 °C and 59.231 °C, respectively. The thermogram of lyophilized SMV-NLC exhibits three endothermic peaks, first peak at 49.858 (for Poloxamer188), second at 57.374 (GMS) and third at 166.109 (for mannitol). But the peak of SMV, which was supposed to appear at 139.975 °C in the lyophilized SMV-NLC, was vanished because drug get fully dissolved in the lipid and the nature of SMV was changed from crystalline to amorphous [Citation36,Citation37].
Fourier transform infrared spectroscopy analysis
The IR spectrum of SMV, physical mixture (SMV, GMS and poloxamer 188) and SMV-loaded optimized NLC formulation was recorded and the characteristic peaks were compared for drug-excipient interaction (). The pure drug showed a very strong characteristic absorption peak at 3540 cm−1 (O–H stretching vibration) and 1699 cm−1 (stretching vibration of C=O group), 1465 cm−1 (benzene ring) 1266 cm−1 (lactone –C–O–C vibration). A comparison of the IR spectral data of pure drug with those of physical mixture and optimized NLC formulation revealed that the characteristic peaks of the drug slightly diminished in intensity and amplitude or slightly get shifted. It clearly indicates that there is no significant physicochemical interaction between the original drug and excipients added. The negligible spectral variation in the final formulation is perhaps due to the overlap of the common functional groups of the excipients.
In vitro drug release study
displays the in vitro release profile of optimized SMV-NLC and SMV-suspension. After 24 h, the % CDR from optimized SMV-NLC and SMV-suspension were found to be 84.09% and 32.20%, respectively. The better release profile of SMV from the NLC formulation was due to its enhanced solubility when incorporated in NLC. From the graph (), it is also clear that drug release from the NLC was biphasic (initial burst release followed by sustained release). The burst release of SMV might be due to the disruption of liquid lipid located near the periphery of NLC particles. The sustained release of SMV at the later stage was due to its slow diffusion from the integrated solid and liquid lipid matrix [Citation38]. The release kinetics of SMV from NLC followed Korsmeyer–Peppas model with higher R2 values of .985 and the release exponent n for this model was found to be 0.49, suggesting the Fickian diffusion pattern of drug release.
In vivo studies (pharmacodynamic)
SMV is notable for lessening the raised level of total cholesterol (TC) and triglycerides (TG) in blood by repressing the catalyst HMG-CoA reductase. Additionally, it expands the level of HDL cholesterol (HDL-C) and in this way advancing the expulsion of cholesterol from the fringe cells and encourages its transportation back to the liver [Citation33]. The pharmacodynamic effect of SMV was found to be dependent on dose, hence was used as a reference for comparing in vivo activities of the developed SMV-NLC and the drug suspension.
The antihyperlipidaemic activity of SMV-NLC formulation compared to SMV suspension was evaluated through in vitro determination of TC, triglycerides (TG) and HDL-C in rodent serum after a treatment period of 0, 4, 7 and 14 days. The lipid profiles in serum of all the experimental groups at different time intervals are shown in .
Figure 9. (A) Percentage change in total serum cholesterol level at different time intervals. (B) Percentage change in serum TG level at different time intervals. (C) Percentage change in HDL level at different time intervals.

Following 14 days of treatment with high-fat eating routine, control group demonstrated huge ascent in total cholesterol (63.14%↑), TG (86.38%↑) and HDL-cholesterol (24.50%↑). Though SMV suspension group appeared around 30.76% expansion in total cholesterol, 31.50% increment in TG and 44.43% expansion in HDL-cholesterol. The SMV-NLC group indicated just around 11.09% increment in total cholesterol, 10.49% expansion in TG and 69.37% expansion in HDL-cholesterol.
SMV-NLC formulation when compared with SMV suspension demonstrated roughly 2.77 times diminish in total cholesterol, three times diminish in TG and 1.56 times increment in HDL-cholesterol which was observed to be significant (p < .05). From the obtained outcome of trial experiment, it was gathered that effectiveness of SMV-NLC in controlling hyperlipidaemia condition was observed to be superior to the SMV suspension. This outcome was credited for the most part to the improved solvency and disintegration on account of NLC definition.
Conclusions
SMV-NLC was developed and optimized using melt-emulsification and Box–Behnken design technique, respectively to achieve sustained release and better antihyperlipidaemic activity of simvastatin. Characterization of SMV-NLC has been done and our developed formulation was found to be with a reasonable particle size (114.62 ± 5.65 nm), low PDI (0.210 ± 0.034) and good entrapment efficiency (85.20 ± 7.98). From the in vitro release study, it is clear that incorporation of SMV in NLC resulted in the sustained release of SMV. The in vivo pharmacodynamic study showed that SMV-loaded NLC produces a better antihyperlipidaemic effect as compared to pure drug suspension of simvastatin, suggesting NLC can be a potential carrier for the delivery of SMV.
Acknowledgements
The author, Meraj Alam is thankful to Ranbaxy Laboratory Limited (Gurgaon, Haryana) for the gift sample of Simvastatin.
Disclosure statement
There is no conflict of interest associated with this research work and manuscript.
References
- World Health Organization. Global Health Observatory (GHO) Data [Internet]. Geneva: WHO; 2017. [cited 2017 June 20]. Available from: http://www.who.int/gho/ncd/risk_factors/cholesterol_text/en/
- Petyaev IM. Improvement of hepatic bioavailability as a new step for the future of statin. Arch Med Sci. 2015;11:406–410.
- Lai J, Chen J, Lu Y, et al. Glyceryl monooleate/poloxamer 407 cubic nanoparticles as oral drug delivery systems: I. in vitro evaluation and enhanced oral bioavailability of the poorly water-soluble drug simvastatin. AAPS PharmSciTech. 2009;10:960–966.
- Shitara Y, Sugiyama Y. Pharmacokinetic and pharmacodynamic alterations of 3-hydroxy-3-methylglutaryl coenzyme A (HMG-CoA) reductase inhibitors: drug-drug interactions and interindividual differences in transporter and metabolic enzyme functions. Pharmacol Ther. 2006;112:71–105.
- Harisa GI, Badran MM. Simvastatin nanolipid carriers decreased hypercholesterolemia induced cholesterol inclusion and phosphatidylserine exposure on human erythrocytes. J Mol Liquids. 2015;208:202–210.
- Kawish SM, Ahmed S, Gull A, et al. Development of nabumetone loaded lipid nano-scaffold for the effective oral delivery; optimization, characterization, drug release and pharmacodynamic study. J Mol Liquids. 2017;231:514–522.
- Müller RH, Mäde K, Gohla S. Solid lipid nanoparticles (SLN) for controlled drug delivery – a review of the state of the art. Eur J Pharm Biopharm. 2000;50:161–177.
- Muller RH, Peterson RD, Hommoss A, et al. Nanostructured lipid carriers (NLC) in cosmetic dermal products. Adv Drug Deliv Rev. 2007;59:522–530.
- Negi LM, Jaggi M, Talegaonkar S. Development of protocol for screening the formulation components and the assessment of common quality problems of nano-structured lipid carriers. Int J Pharm. 2014;461:403–410.
- Moghddam SM, Ahad A, Aqil M, et al. Optimization of nanostructured lipid carriers for topical delivery of nimesulide using Box-Behnken design approach. Artificial Cells Nanomed Biotechnol. 2017;45:617–624.
- Shete H, Patravale VS. Long chain lipid based tamoxifen NLC. Part I: preformulation, formulation development and physicochemical characterization. Int J Pharm. 2013;454:573–583.
- Gaba B, Fazil M, Khan S, et al. Nanostructured lipid carrier system for topical delivery of terbinafine hydrochloride. Bull Fac Pharm Cairo Univ. 2015;53:147–159.
- Zhang W, Li X, Ye T, et al. Design, characterization, and in vitro cellular inhibition and uptake ofoptimized genistein-loaded NLC for the prevention of posterior capsular opacification using response surface methodology. Int J Pharm. 2013;454:354–366.
- Alam T, Pandit J, Vohora D, et al. Optimization of nanostructured lipid carriers of lamotrigine for brain delivery: in vitro characterization and in vivo efficacy in epilepsy. Expert Opin Drug Deliv. 2015;12:181–194.
- Huang ZR, Hua SC, Yang YL, et al. Development and evaluation of lipid nanoparticles for camptothecin delivery: a comparison of solid lipid nanoparticles, nanostructured lipid carriers, and lipid emulsion. Acta Pharmacol Sin. 2008;29:1094–1102.
- SrivastavaKohli MK, Ali M. Formulation development of novel in situ nanoemulgel (NEG) of ketoprofen for the treatment of periodontitis. Drug Deliv. 2016;23:154–166.
- Ahmed S, Sarim Imam S, Zafar A, et al. In vitro and preclinical assessment of factorial design based nanoethosomes transgel formulation of an opioid analgesic. Artif Cells Nanomed Biotechnol. 2016;44:1793–1802.
- Ahmad J, Mir SR, Kohli K, et al. Quality by design approach for self-nanoemulsifying system of paclitaxel. Sci Adv Mater. 2014;6:1778–1791.
- Kumar VV, Chandrasekar D, Ramakrishna S, et al. Development and evaluation of nitrendipine loaded solid lipid nanoparticles: influence of wax and glyceride lipids on plasma pharmacokinetics. Int J Pharm. 2007;335:167–175.
- Qadri GR, Ahad A, Aqil M, et al. Invasomes of isradipine for enhanced transdermal delivery against hypertension: formulation, characterization and in vivo pharmacodynamic study. Artif Cells Nanomed Biotechnol. 2017;45:139–145.
- Ali Z, Mishra N, Baldi A. Development and characterization of arteether-loaded nanostructured lipid carriers for the treatment of malaria. Artif Cells Nanomed Biotechnol. 2016;44:545–549.
- Agrawal Y, Petkar KC, Sawant KK. Development, evaluation and clinical studies of Acitretin loaded nanostructured lipid carriers for topical treatment of psoriasis. Int J Pharm. 2010;401:93–102.
- Ahmad J, Mir SR, Kohli K, et al. Solid-nanoemulsion preconcentrate for oral delivery of paclitaxel: formulation design, biodistribution, and γ scintigraphy imaging. BioMed Res Int. 2014;2014:984756.
- Tsai MJ, Huang YB, Wu PC, et al. Oral apomorphine delivery from solid lipid nanoparticles with different monostearate emulsifiers: pharmacokinetic and behavioral evaluations. J Pharm Sci. 2011;100:547–557.
- SahanaSantra B, Basu K, Mukherjee SB. Development of biodegradablepolymerbased tamoxifen citrate loaded nanoparticles and effect of some manufacturing process parameters on them: a physicochemical and in-vitro evaluation. Int J Nanomedicine. 2010;5:621–630.
- Zhuanga C, Li Y, Wanga NM, Zhang XN, et al. Preparation and characterization of vinpocetine loaded nanostructured lipid carriers (NLC) for improved oral bioavailability. Int J Pharm. 2010;394:179–185.
- Rizwanullah M, Amin S, Ahmad J. Improved pharmacokinetics and antihyperlipidemic efficacy of rosuvastatin-loaded nanostructured lipid carriers. J Drug Target. 2017;25:58–74.
- Zhang Z, Bu H, Gao Z, et al. The characteristics and mechanism of simvastatin loaded lipid nanoparticles to increase oral bioavailability in rats. Int J Pharm. 2010;394:147–153.
- Alam S, Khan ZI, Mustafa G, et al. Development and evaluation of thymoquinone encapsulated chitosan nanoparticles for nose-to brain targeting: a pharmaco scintigraphic study. Int J Nanomed. 2012;7:5705–5718.
- Reagan-Shaw S, Nihal M, Ahmad N. Dose translation from animal to human studies revisited. Faseb J. 2008;22:659–661.
- Shin JW, Seol IC, Son CG. Interpretation of animal dose and human equivalent dose for drug development. J Korean Oriental Med. 2010;31:1–7.
- Dixit RP, Nagarsenker MS. Formulation and in vivo evaluation of self-nanoemulsifying granules for oral delivery of a combination of ezetimibe and simvastatin. Drug Dev Ind Pharm. 2008;34:1285–1296.
- Chavhan SS, Petkar KC, Sawant KK. Simvastatin nanoemulsion for improved oral delivery: design, characterisation, in vitro and in vivo studies. J Microencapsul. 2013;30:771–779.
- Magnusson G, Olsson T, Nyberg JA. Toxicity of Pluronic F-68. Toxicol Lett. 1986;30:203–207.
- Huang J, Si L, Jiang L, et al. Effect of pluronic F68 block copolymer on P-glycoprotein transport and CYP3A4 metabolism. Int J Pharm. 2008;356:351–353.
- Harivardhan Reddy L, Murthy RSR. Etoposide-loaded nanoparticles made from glyceride lipids: formulation, characterization, in vitro drug release, and stability evaluation. AAPS Pharm Sci Technol. 2005;6:E158–E166.
- Sarmento B, Ferreira D, Veiga F, et al. Characterization of insulin loaded alginate nanoparticles produced by ionotropic pre-gelation through DSC and FTIR studies. Carbohydr Polym. 2006;66:1–7.
- Aslam M, Aqil M, Ahad A, et al. Application of Box–Behnken design for preparation of glibenclamide loaded lipid based nanoparticles: Optimization, in vitro skin permeation, drug release and in vivo pharmacokinetic study. J Mol Liquids. 2016;219:897–908.