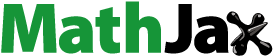
Abstract
Sirolimus (rapamycin) is a mammalian target of rapamycin (mTOR) inhibitor with immunosuppressive, antiproliferative, antiangiogenic, antifungal, anti-restenosis and anti-inflammatory properties. However, its clinical application is often hampered by poor aqueous solubility, first-pass metabolism, transport by p-glycoprotein efflux pump, limited oral bioavailability and nonspecific distribution in off-target sites. Recently, various formulation strategies have emerged to overcome these limitations. Among these, pharmaceutical nanotechnology with numerous advantages has great potential for sirolimus delivery. Up to now, the only nanoparticle based FDA approved formulation in the market is Rapamune® tablet which is composed of drug nanocrystals. This review focuses on recent studies that have been investigated various nanostructured carriers such as liposomes, micelles, polymeric nanoparticles, nanocrystals, magnetic nanoparticles, albumin nanoparticles, solid dispersion nanoparticles and niosomes for sirolimus delivery (in organ transplantation, cancer, vascular restenosis, etc.).
Graphical Abstract

Introduction
Sirolimus, also known as rapamycin, is a clinically important polyketide macrolide compound () originally produced by fermentation from Streptomyces hygroscopicus collected from Easter Island (Rapa Nui) [Citation1]. The macrolactone ring of sirolimus is synthesized by mixed polyketide synthase/non-ribosomal peptide synthetase systems using 4,5-dihydrocyclohex-1-ene-carboxylic acid as a starter unit. The linear polyketide chain condensation with pipecolate and further cyclization form the macrolide ring that then undergoes a series of post-polyketide synthase modifications [Citation2].
Figure 1. Schematic illustration of the mammalian target of rapamycin (mTOR) pathway and sirolimus (SIR) mechanism. mTOR integrates in/out from numerous upstream cues including several growth factors and interleukin-2 (IL-2). After entry into the cell, sirolimus associates with FK506 binding protein 12 (FKBP-12). The resulting complex interacts with the FKBP12-rapamycin binding domain located in the carboxyl terminus of mTOR and inhibits the mTOR kinase activity.

After entry into the cell, sirolimus associates with the abundant immunophilin, FK506 binding protein 12 (FKBP-12) (). The resulting complex interacts with the FKBP12-rapamycin binding domain, located in the carboxyl terminus of mammalian target of rapamycin (mTOR) and inhibits the mTOR kinase activity [Citation3]. By binding to and inhibiting mTOR, sirolimus blocks activation of p70S6 kinase resulting in cell cycle arrest at the G1 to S phase. mTOR inhibition also blocks interleukin-2 (IL-2) receptor and CD28-dependent signalling pathways. mTOR is the central component of a complex intracellular signalling pathway involved in cellular processes such as cell growth and proliferation, immunity, angiogenesis, fibrogenesis and metabolism () [Citation4,Citation5].
After oral administration of both liquid and solid formulations, sirolimus is rapidly absorbed with a time to peak blood concentration of about 1–2 h. It has poor (F = 14%) and variable oral absorption. First pass elimination by the intestinal and hepatic cytochrome P450 and p-glycoprotein activity likely contribute to low and variable bioavailability. Absorption is variably affected by food, especially high-fat meals that decrease the oral bioavailability [Citation6]. Following absorption, most drug molecules in human whole blood, partition into red blood cells and approximately 40% of the drug in plasma is bound to proteins, mainly to albumin. Because of its lipophilic nature, sirolimus is widely distributed, displaying a large apparent volume of distribution (5.6–16.7 L/kg). Target whole blood sirolimus concentration ranges from 5 to 15 ng/mL. It is extensively metabolized by the cytochrome P450 isoenzyme CYP3A4 and hence extensive drug–drug interactions may occur [Citation6,Citation7]. Sirolimus biotransformation occurs by hydroxylation or demethylation and several metabolites have been identified. The terminal half-life has been reported to be in the range of 57–72 h. The majority of parent drug and its metabolites are excreted via the faeces, with only about 2% undergoing renal excretion [Citation6,Citation8]. Sirolimus pharmacokinetics displays wide inter- and intra-patient variability. Therefore, for prevention of graft rejection and minimizing toxicity, careful blood level monitoring is emphasized [Citation9].
Isolated from fungi in the soil of Easter Island with anticandida activity, sirolimus subsequently shows impressive immunosuppressive activity. Demonstration of remarkable immunosuppressive potency in preclinical studies and clinical trials led to sirolimus approval for prophylaxis of renal graft rejection. It has been also investigated for liver, heart, lung and pancreas transplantation. Sirolimus is administered with other immunosuppressants (cyclosporine, corticosteroids, mycophenolate mofetil and tacrolimus) as early as possible after transplantation [Citation3,Citation10]. Sirolimus-eluting stents have been shown to be very effective in reducing coronary restenosis, re-intervention rates and other adverse cardiac events in patients with coronary artery disease [Citation11,Citation12]. Antiproliferative properties of sirolimus make it beneficial for the treatment of tumours and some benign pathological states associated with abnormal proliferation (). Sirolimus and its analogues are beneficial for treating various other diseases and conditions, including tuberous sclerosis, Kaposi’s sarcoma, diabetes, lymphangioleiomyomatosis, uveoretinitis, keloids, scars, psoriasis, Muir–Torre syndrome and aging [Citation13–15]. Approved and investigational uses of sirolimus are summarized in [Citation13–27]. There are reports on radiosensitizing ability of sirolimus in different cancers [Citation28–30]. Sirolimus is currently available as oral solution and tablet in market.
Figure 2. Indications for which sirolimus has been investigated. A growing number of evidence has demonstrated that sirolimus is beneficial for treating various diseases [Citation13–27]. GVHD: Graft-versus-host disease; HIV: Human immunodeficiency virus; LAM: Lymphangioleiomyomatosis.
![Figure 2. Indications for which sirolimus has been investigated. A growing number of evidence has demonstrated that sirolimus is beneficial for treating various diseases [Citation13–27]. GVHD: Graft-versus-host disease; HIV: Human immunodeficiency virus; LAM: Lymphangioleiomyomatosis.](/cms/asset/d916dfa1-1865-4e08-b74e-1749b647a33b/ianb_a_1408123_f0002_c.jpg)
In spite of various promising pharmacological activities of sirolimus, it faces a number of limitations that need to be addressed by novel drug delivery systems. Sirolimus has some dose-dependent adverse effects, including immunosuppression, inhibition of bone growth, increased cholesterol, triglycerides and creatinine serum levels and decreased glomerular filtration rate. Leukopenia, thrombocytopenia, anaemia, rash, stomatitis, arthralgia, diarrhoea, hypertension and hypokalaemia may also occur [Citation31]. Moreover, the lipophilic nature of sirolimus allows its wide distribution in lipid membranes resulting in a large volume of distribution and relatively long half-life [Citation6,Citation7].
Another major challenge for in vivo application of sirolimus is its strong hydrophobic nature which leads to difficulties in preparing intravenous formulations and delivery to a target site in effective concentrations. Sirolimus has a molecular weight of 914 g/mol, partition coefficient (log P) of 5.8, no ionizable functional groups within the pH range of 1–10 and a very poor water solubility (2.6 µg/mL) [Citation32,Citation33]. Poor water soluble drugs are generally dissolved using large amounts of excipients. However, the need for frequent drug administration raises more concerns due to possible toxic effects of the excipients. Sirolimus shows low oral bioavailability due to sensitivity to gastric acid, limited solubility and permeability, first-pass metabolism and transport by p-glycoprotein efflux pump [Citation6,Citation7].
High sequestration of free sirolimus into the erythrocytes may also hinder accessibility into target sites (tumour tissues). It shows inter- and intra-patient variability in drug pharmacokinetics [Citation6,Citation7]. The above mentioned limitations stress the need to improve delivery formulation of sirolimus.
The emergence of pharmaceutical nanotechnology has great potential for sirolimus delivery due to small size, enhanced drug solubilization and dissolution rates, high efficiency in drug encapsulation and cellular uptake, controlled drug release, cargo protection from harsh environment, escape from reticuloendothelial system (RES), long circulation residence, targeted delivery to specific areas and modulation of drug pharmacokinetics and biodistribution [Citation34–36]. This review focuses on recent studies that have investigated various nanostructured carriers such as liposomes, micelles, polymeric nanoparticles, nanocrystals, magnetic nanoparticles, albumin nanoparticles, solid dispersion nanoparticles and niosomes for sirolimus delivery (in organ transplantation, cancer, vascular restenosis, etc.) ().
Sirolimus delivery using liposomes
Liposomes are spherical vesicles that have an internal aqueous phase surrounded by one or more bilayer membranes mimicking human cell membranes. They are considered the oldest nanoformulations and several liposomes have been approved in the last 20 years. Liposomes are composed of cholesterol and amphiphilic phospholipids. They are classified into uni-, oligo- or multi-lamellar according to number of layers and size. Depending upon the preparation methods and composition, size of liposomes ranges from around 50 nm to several microns [Citation37–39]. Liposomes have several desirable properties for delivery of various therapeutic agents, including biodegradability, superior biocompatibility, sustained-release properties, capability of hydrophobic and hydrophilic drugs entrapment, low clearance rates, surface modification, safety, cargo protection from external degradation and flexibility in adjusting physicochemical characteristics [Citation38,Citation40]. They also preferentially accumulate at pathologic sites of inflammation, infections and tumours [Citation41]. To shield from the recognition by RES and increase circulation time of liposomes, polyethylene glycol (PEG) conjugated phospholipids are inserted into the membrane bilayer and sterically stabilized (stealth) liposomes have been developed [Citation42]. Moreover, by this modification conjugating different targeting moieties to the liposomal surface can be facilitated [Citation43]. Many researches have been focused on sirolimus liposomal formulations and some recent examples have been summarized in .
Table 1. Nanocarrier compositions and characteristics of sirolimus liposomal formulations.
In our series of studies on formulation of an effective drug delivery system for restenosis treatment, first we focused on preparation and optimization of sirolimus nanoliposomes [Citation44]. Sirolimus liposomes were prepared by the remote film loading method. This method involved the addition of empty liposomes to drug thin film and the subsequent sonication for a few minutes. The effects of key formulation variables (cholesterol proportion, lipid to drug molar ratio, lipid composition and sonication time) on drug encapsulation efficiency (EE) and release rate were investigated using a fractional factorial design. By this approach, almost complete drug entrapment was achieved. In vitro release studies revealed that nanoliposomes could control the drug release for 3 weeks [Citation44].
To develop the optimized carrier for local delivery, the effects of various physicochemical parameters of liposomes (surface charge, particle size and incorporation of PEG) on the anti-restenosis efficacy were evaluated using morphometric, immunohistochemical and in vivo computed tomography imaging analyses. The restenosis inhibitory efficacy decreased in the following order: cationic ∼100 nm vesicles ≥ cationic ∼60 nm vesicles > neutral ∼100 nm vesicles ≥ stealth ∼100 nm vesicles > anionic ∼100 nm vesicles [Citation45]. Observing higher efficacy by 1,2-dioleoyl-3-trimethylammonium-propane (DOTAP) enriched cationic vesicles compared to other formulations, we proposed that more biocompatible chitosan coating could improve local retention and efficacy [Citation46]. Sirolimus loaded chitosan decorated liposomes were prepared and characterized. In vivo evaluation by pathology, immunohistochemical and in vivo CT angiography imaging confirmed the greater advantages of chitosan dressed vesicles as nanocarriers for sirolimus intramural delivery over the conventional liposomes () [Citation46].
Figure 4. Chitosan decorated sirolimus liposomes attenuated vascular restenosis following local delivery as confirmed by pathology, immunohistochemical and in vivo CT angiography. Reprinted from ref [Citation46]. Copyright (2017), with permission from Elsevier.
![Figure 4. Chitosan decorated sirolimus liposomes attenuated vascular restenosis following local delivery as confirmed by pathology, immunohistochemical and in vivo CT angiography. Reprinted from ref [Citation46]. Copyright (2017), with permission from Elsevier.](/cms/asset/095bae75-156a-4507-a0db-dc706061ab74/ianb_a_1408123_f0004_c.jpg)
In another study, sirolimus and indocyanine green (ICG) were co-encapsulated into folate targeted thermosensitive liposomes to enhance tumour therapeutic and diagnostic functions () [Citation47]. ICG is a near infrared (NIR) fluorescent dye that generates high fluorescence and photoacoustic signals. The theranostic targeted thermosensitive liposome was ∼150 nm, spherical shape and stable for at least 4 weeks. Following irradiation, percentage of sirolimus released nearly doubled compared to without irradiation indicating that NIR-induced hyperthermia could accelerate drug release. Both NIR-induced hyperthermia and folate anchoring dramatically enhanced the cellular uptake of the two agents and cytotoxicity in HUVEC and HeLa cells. In vivo studies showed a much higher antitumour activity without causing systemic toxicity. Moreover, photothermal therapy and sirolimus showed synergistic effect on the ablation of tumour vessels () [Citation47].
Figure 5. Schematic structure of the theranostic targeted thermosensitive liposome (A) and its antitumour mechanism (B). Redrawn with permission from Pang et al. [Citation47]. Copyright (2016) American Chemical Society.
![Figure 5. Schematic structure of the theranostic targeted thermosensitive liposome (A) and its antitumour mechanism (B). Redrawn with permission from Pang et al. [Citation47]. Copyright (2016) American Chemical Society.](/cms/asset/78bc6671-5a06-4312-91f2-86e91a81167a/ianb_a_1408123_f0005_c.jpg)
The proposed mechanisms for this efficient multifunctional carrier were as follow: (1) through enhanced permeability and retention (EPR) as well as folate mediated active targeting effects, the formulation was selectively accumulated at the tumour site and efficiently internalized into endothelial and tumour cells; (2) this theranostic probe precisely tracked the tumour tissue, focused the laser beam on the tumour region and converted NIR laser light energy to hyperthermia; (3) hyperthermia generated by activation of ICG under NIR irradiation mediated photothermal therapy and triggered sirolimus release from the thermosensitive liposomes; and (4) through blocking the regulation effect of mTOR by the released drug, antiproliferative and antiangiogenic effects were observed [Citation47].
Sirolimus delivery using polymeric nanoparticles
Polymeric nanoparticles (NPs), invented in the 1970s, are solid colloidal systems composed of natural, synthetic or semi-synthetic polymers with size ranging from 10 to 1000 nm [Citation57]. Chitosan, alginate and gelatine are a few examples of widely investigated natural polymers. Synthetic polymers extensively studied for various therapeutic delivery are poly (DL-lactide-co-glycolide) (PLGA), polycaprolactone (PCL), poly(lactic acid) (PLA), polyacrylic acid (PAA), poly(methyl methacrylates) and polyalkylcyanoacrylate [Citation58,Citation59]. Physicochemical properties of the employed polymer determine the fabrication process requirements and conditions to form NPs. Polymeric NPs have been extensively investigated as pharmaceutical delivery systems owing to their small size, controlled release properties, reduced toxicity, prolonged circulation time, cargo protection from harsh environments, enhanced therapeutics bioavailability, surface modification and functionalization and targeted capabilities [Citation60]. Nanocapsules are reservoir-type NPs, in which the cargo is present in the core surrounded by a polymer membrane, while nanospheres are matrix based NPs in which the cargo is uniformly dispersed throughout the matrix [Citation61]. Sterically stabilized (stealth) NPs, carrying PEG coatings, maintain an increased circulation time and predominantly increase the feasibility to accumulate in pathological sites due to EPR effect [Citation42]. summarizes the recent researches on sirolimus polymeric NPs.
Table 2. Nanocarrier compositions and characteristics of sirolimus polymeric nanoparticles.
Shi et al. [Citation62] prepared and characterized sirolimus loaded PLGA NPs embedded in pluronic gel system for periadventitial delivery around the carotid artery immediately after open vascular reconstruction surgery. Sirolimus was encapsulated in NPs via a single emulsion method with respective EE and loading level of 69.1 and 11.6%. In vitro release studies revealed that drug loaded NPs dispersed in gel provided a more sustained release profile than free drug containing pluronic gel. Efficient uptake of NPs by vascular smooth muscle cells was observed in vitro, allowing for more sustained sirolimus delivery and durable inhibition of phosphorylation of S6 kinase. In vivo studies in a rat carotid injury model revealed that drug loaded NPs led to a more sustained inhibition of S6 kinase, vascular smooth muscle cells proliferation, intimal hyperplasia and restenosis compared to the free drug [Citation62].
As we mentioned before, sirolimus low solubility and extensive efflux by p-glycoprotein limit its oral bioavailability. In a recent study, Katiyar et al. [Citation63] reported PLGA NPs formulation of sirolimus, along with a chemosensitizer (piperine) for improved oral bioavailability and anti-cancer efficacy. In cytotoxicity studies on MDA-MB-231 cell line, half-maximal inhibitory concentration (IC50) of sirolimus solution and sirolimus NPs were 20.3 and 11.4 µM, respectively. By using everted gut sac technique, it was revealed that permeation of sirolimus was increased by NP formulation as well as by chemosensitizer treatment. After oral administration, 1.7-fold increase was observed in bioavailability of sirolimus suspension in the presence of the chemosensitizer suspension. Furthermore, there was a 3.5- fold increase in drug bioavailability of NPs as compared to drug suspension. The co-delivery of sirolimus and piperine by PLGA NPs resulted in ∼4.8-fold increase in bioavailability compared to drug suspension. This study has proven the potential of combination piperine/sirolimus NPs in the oral delivery of hydrophobic sirolimus [Citation63].
Sirolimus delivery using micelles
Micelles are spherical or globular colloidal particles made of monolayers with diameters generally less than 100 nm. Traditional micelles, form from an average number (aggregation number) of surfactant monomers, generally have high critical micelle concentration (CMC) and low stability upon dilution. Recently, polymeric micelles, self-assembled NPs prepared from amphiphilic block copolymers in aqueous media, have attracted a great deal of attention due to lower CMC, better thermodynamic stability in physiological solutions, slower rate of dissociation allowing a longer drug retention time, narrow and small size distribution, higher solubilization capacity, controlled drug release and greater accumulation at the target sites mediated by escaping from RES uptake and EPR effect [Citation77]. The block copolymer micelles can be subcategorized into at least two main types: 1) Amphiphilic block copolymer micelles and 2) polyion complex micelles or block ionomer complexes. A-B diblock copolymer, A-B-C triblock copolymer, grafted and hyperbranched polymers are different patterns of micelles formed by arranging these block copolymers [Citation78,Citation79]. Based on a series of studies, drug-loading capacity of micelles can be significantly improved by optimizing chemical structures of inner core for stronger carrier–cargo interaction [Citation77]. Due to numerous advantages of micelles, many researches have been performed on micelles formulation and in vitro as well as in vivo characterization of sirolimus ().
Table 3. Nanocarrier compositions and characteristics of sirolimus micelles.
Shin et al. [Citation80] designed “Triolimus” a 3-in-1 poly(ethylene glycol)-block-poly(D,L-lactic acid) (PEG-b-PLA) based micellar nanocontainer for co-delivery of sirolimus, paclitaxel and 17-allylamino-17-demethoxygeldanamycin (17-AAG). The water solubility was increased by 80-, 104- and 700- fold, respectively. All drugs were released simultaneously over 24 h period. 3-in-1 micelles had the highest cytotoxicity and combination index analysis revealed that 3-in-1 micelles exerted strong synergy in 4T1 and MCF-7 breast cancer cells. Acute toxicity of the micelle was surprisingly low at respective doses of 30, 60 and 60 mg/kg for sirolimus, paclitaxel and 17-AAG [Citation80]. The investigators extended their initial observations and studied in vivo efficacy [Citation81] as well as pharmacokinetics [Citation82] of the nanosystem. Mechanistically, the drug combination inhibited both the PI3K/AKT/mTOR and Ras/Raf/MAPK pathways. In A549 and MDA-MB-231 tumour xenograft models, a three-infusion schedule of Triolimus displayed more potent and durable tumour control than paclitaxel-containing micelles [Citation81]. After intravenous administration of Triolimus to mice at high doses of 30 mg/kg sirolimus, 60 mg/kg paclitaxel and 60 mg/kg 17-AAG, the area under the plasma concentration–time curves (AUC) of sirolimus and paclitaxel increased compared to single-drug‐loaded micelles. In contrast, following administration of the 3-in-1 micelles at modest dose (sirolimus, paclitaxel and 17-AAG at 5, 10 and 10 mg/kg, respectively), pharmacokinetic differences between 3-in-1 and single drug formulations were not observed [Citation82]. All data suggested that the nano-package cocktail provided a novel and efficient approach for combination cancer therapy.
In another study, a trilayer polymeric micelle system composed of the triblock poly(ethylene glycol)-b-poly N-[N-(2-aminoethyl)-2-aminoethyl] aspartamide-b-poly(ε-caprolactone) (PEG-b-PAsp(DET)-b-PCL) polymer was synthesized and studied for co-delivery of siRNA targeting Y-box binding protein-1 and sirolimus [Citation83]. The outer PEG layer was used to improve pharmacokinetics, the cationic pH responsive PAsp(DET) segment was designed to interact with siRNA, and the inner hydrophobic PCL compartment was used for efficient sirolimus entrapment. The co-loaded micelles were ∼108 nm and stable in phosphate buffered saline (PBS) for 7 d at room temperature. The micelles efficiently controlled sirolimus release rate. Combination siRNA/sirolimus micelles greatly enhanced in vitro cytotoxicity against PC3 prostate cancer cells and induced significant tumour regression and growth inhibition in a PC3 xenograft mouse model of human prostate cancer [Citation83].
Sirolimus delivery using nanocrystals
One promising approach to overcome the solubility limitation of hydrophobic cargos is transforming the drug powder into nanocrystals or nanosuspensions. Nanocrystals are pure solid drug particles with a mean diameter below 1 µm and nanosuspensions are submicron colloidal aqueous dispersions of drug particles. Nanocrystals can be administered by various routes; however, product developments have mainly focused on the oral route of administration [Citation93]. Particle size plays a key role in the in vivo performance of drug nanosuspensions. According to Noyes–Whitney equation (EquationEquation (1)(1)
(1) ), where dX/dt is the dissolution rate, S represents the surface area, D is the diffusion coefficient of the solute, h represents the thickness of the dissolution boundary layer, Cs is the saturation solubility and Ct is the bulk solution concentration, a decrease in particle size into the nanoscale causes an increase in total effective surface area, the dissolution rate and in vivo absorption [Citation94,Citation95].
(1)
(1)
Bottom-up and top-down methods are the two primary techniques for drug nanocrystal production. The bottom-up processes involve the formation of NPs by controlled precipitation at nanometre scale, while the top-down processes associate with applying shear stress on large drug particles by milling or homogenization to reduce particle size [Citation93,Citation95,Citation96].
Rapamune® tablet was the first marketed nanocrystal product launched in 2002 by Wyeth Pharmaceuticals. Compared to drug solution, the tablet has the advantage of being easy to administer and 21% higher bioavailability. The oral single dose of Rapamune® is 1 or 2 mg and the total tablet weight is ∼365 mg for 1 mg formulation and ∼370 mg for the 2 mg formulation. The inactive ingredients that can be found in the labelling information of the Rapamune® are lactose, sucrose, PEG 8000, PEG 20,000, microcrystalline cellulose, calcium sulphate, pharmaceutical glaze, talc, magnesium stearate, titanium dioxide, poloxamer 188, povidone, glyceryl monooleate, dl-alpha tocopherol and carnauba wax. This product has been produced by the wet milling top-down method [Citation96].
In this technique, drug particles are dispersed in a surfactant/stabilizer media and then particle size of the obtained suspension is reduced by milling energy. In NanoCrystal™ technology used in Rapamune®, drug, dispersion medium, milling media and stabilizer are filled into the milling chamber. High shear force generated by the collision of the solid drug crystals with the milling media results in particle size reduction. The balls or pearls used as milling media consist of stainless steel, glass, ceramics or highly cross-linked polystyrene resin-coated beads [Citation95,Citation96].
Miscellaneous nanocarriers
Other nanoformulations have also been explored for sirolimus delivery such as magnetic NPs, albumin NPs, solid dispersion NPs, niosomes, nanoemulsions, β-cyclodextrin-based NPs and lipid-based NPs that are summarized in .
Table 4. Nanocarrier compositions and characteristics of sirolimus incorporated in miscellaneous nanoformulations.
In a recent study, Dou et al. [Citation97] developed an acetalated β-cyclodextrin (Ac-bCD) based NP for sustained sirolimus delivery for atherosclerosis (). For chronic inflammatory diseases, biodegradable materials (such as polyanhydrides and polyesters) with acidic byproducts may lead to local inflammation. In this study, kinetically controlled acetylation was used to synthetize biocompatible and biodegradable materials of Ac-bCDs. Ac-bCDs degrade into a non-acidic and water-soluble parent compound under physiological conditions. High EE (>90%) and slowed release rate were achieved. Pharmacokinetic studies revealed a prolonged profile of drug concentration in the blood after subcutaneous administration of 3 mg/kg sirolimus NPs. Nearly constant drug levels in the blood and the aorta (around 50 ng/L and 1.2 ng/g, respectively) were maintained for 13 d (). Apolipoprotein E-deficient mice were treated by subcutaneous administration of drug NPs at the lower (1.0 mg/kg of sirolimus) and higher (3.0 mg/kg of sirolimus) dose every 15 d. The average lesion area was 4.7 and 3.9%, respectively (). Sirolimus treatment significantly diminished macrophages and matrix metallopeptidase 9 (MMP-9) in the plaques and serum inflammatory cytokines including tumour necrosis factor α (TNF-α), interferon-γ (INF-γ), IL-1α and IL-1β. Moreover, NPs treatment dramatically stabilized atherosclerotic plaques. These effects were more remarkable for NPs treatment groups as compared to the free drug group. These findings demonstrated that sustained sirolimus delivery may provide more significant antiatherosclerotic activity over traditional administration through selective inhibiting of mTORC1 signalling in apolipoprotein E-deficient mice [Citation97].
Figure 6. Design of sustained sirolimus delivery nanomedicines based on acetalated β-CD (A), sirolimus concentrations in the blood and the aorta of C57BL/6 mice (n = 4, mean ± SEM), after subcutaneous administration of sirolimus nanoparticles at 3 mg/kg of drug (B). Therapy with sirolimus nanoparticle significantly reduced atherosclerosis in ApoE−/−mice (C). (C) Representative photographs of total aortas from each group and quantitative results subjected to various treatments. Reprinted with minor modification from Dou et al. [Citation97]. Copyright (2016), with permission from Elsevier.
![Figure 6. Design of sustained sirolimus delivery nanomedicines based on acetalated β-CD (A), sirolimus concentrations in the blood and the aorta of C57BL/6 mice (n = 4, mean ± SEM), after subcutaneous administration of sirolimus nanoparticles at 3 mg/kg of drug (B). Therapy with sirolimus nanoparticle significantly reduced atherosclerosis in ApoE−/−mice (C). (C) Representative photographs of total aortas from each group and quantitative results subjected to various treatments. Reprinted with minor modification from Dou et al. [Citation97]. Copyright (2016), with permission from Elsevier.](/cms/asset/49011059-bda2-45d8-9004-24722ea67f1a/ianb_a_1408123_f0006_c.jpg)
In another study, pH-sensitive niosomal formulation was prepared and characterized to improve sirolimus efficacy and selectivity [Citation98]. Vesicles were composed of Span 60 and either cholesteryl hemisuccinate (CHEMS) or PEG-Poly (monomethyl itaconate)-CholC6 copolymers for pH sensitivity and prepared by a modified ethanol injection method. Vesicles’ physicochemical characteristics, pH-sensitivity, stability and in vitro antiproliferative activity were evaluated. Mean particle size of formulations was ∼285 nm and %EE of prepared niosomes was in the range of 68.2–75.3%. Drug release rate from two pH-sensitive formulations at pH 7.4 was much slower than release rate at pH 6.5. However, after 1 h incubation of CHEMS-based vesicles in plasma, pH-sensitivity property was lost and high release rate occurred at pH 7.4. Whereas, pH-sensitive PEG-Poly (monomethyl itaconate)-CholC6 bearing vesicles were stable in plasma and could release drug in the mild acidic environments, often present in endosomal compartment and tumour. Higher antiproliferative activity against erythromyeloblastoid leukaemia cell line was observed with both pH-sensitive niosomes compared with conventional niosomes. In contrast, conventional niosomes showed higher antiproliferative effect on HUVEC cells as a healthy cell line. The results revealed that this novel pH-sensitive niosome can improve sirolimus in vitro efficiency [Citation98].
Conclusions
The preclinical and clinical studies performed over the last two decades have shown that sirolimus (rapamycin) shows efficacy in different diseases and conditions, including prophylaxis of graft rejection, restenosis, tumours, Kaposi’s sarcoma, diabetes, lymphangioleiomyomatosis, uveoretinitis, keloids, scars, psoriasis and aging. Although sirolimus has shown efficacy in various pathological conditions, its potency has substantially diminished by sirolimus dose-dependent adverse effects, wide distribution into lipid membranes, extensive apparent volume of distribution, strong hydrophobic nature, difficulties in preparing intravenous formulations, low oral bioavailability, high sequestration of free drug into the erythrocytes, low accessibility into target sites and inter- and intra-patient variability in drug pharmacokinetics.
To overcome these obstacles, the various nanocarriers, including liposomes, micelles, polymeric NPs, nanocrystals, magnetic NPs, albumin NPs, solid dispersion NPs, nanoemulsions, lipidic NPs and niosomes have been under development and evaluation. As summarized in this article, many of these nanoformulations have been found to be appropriate for the sirolimus incorporation as well as controlled and targeted sirolimus delivery to improve its effects in several distinct diseases and reduce its side effects. The characteristics of these nanosystems can be tuned according to the specific requirements of the target disease. Based on our understanding from the literature, the growing number of sirolimus researches in chemotherapy for cancer treatment can improve existing therapies by targeting tumours as well as by reducing the administered dose.
Impressive results of different sirolimus nanostructures and improved drug efficacy in the cell lines and animal models highlight their potential for evaluation in clinical trials to establish their effectiveness in clinics. In spite of numerous advantages of the studied nanocarriers, the main obstacles are lack of tissue and cell specificity and off-target drug distribution. A few studies were focused on combination of sirolimus with contrast-, imaging-, antibody- or ligand-mediated targeted delivery. Therefore, future studies are greatly needed to achieve better-targeted and advanced therapeutic modalities. Furthermore, more attention should be paid to the toxicity of sirolimus nanoformulations, particularly after repeated and high dose administration. So far, the emphasis of most sirolimus nanoformulations is particularly on treatment of cancer and vascular restenosis. Studies on potential of these nanocarriers for treatment of other conditions, including diabetes, Alzheimer, infections, keloids, scars, psoriasis and aging are in great demand.
Abbreviations | ||
17-AAG | = | 17-allylamino-17-demethoxygeldanamycin |
Ac-bCD | = | acetalated β-cyclodextrin |
AUC | = | area under the plasma concentration–time curves |
CHEMS | = | cholesteryl hemisuccinate |
CMC | = | critical micelle concentration |
DOTAP | = | 1,2-dioleoyl-3-trimethylammonium-propane |
EE | = | encapsulation efficiency |
EPR | = | enhanced permeability and retention |
FKBP-12 | = | FK506 binding protein 12 |
GVHD | = | graft-versus-host disease |
HIV | = | human immunodeficiency virus |
HUVEC | = | human umbilical vein endothelial cells |
IC50 | = | half-maximal inhibitory concentration |
ICG | = | indocyanine green |
INF-γ | = | interferon-γ |
IL | = | interleukin |
LAM | = | lymphangioleiomyomatosis |
mTOR | = | mammalian target of rapamycin |
MMP-9 | = | matrix metallopeptidase 9 |
NIR | = | near infrared |
NPs | = | nanoparticles |
PAA | = | polyacrylic acid |
PBS | = | phosphate buffered saline |
PCL | = | polycaprolactone |
PEG | = | polyethylene glycol |
PEG-b-PAsp(DET)-b-PCL | = | poly(ethylene glycol)-b-poly N-[N-(2-aminoethyl)-2-aminoethyl] aspartamide-b-poly(ε-caprolactone) |
PEG-b-PLA | = | poly(ethylene glycol)-block-poly(D,L-lactic acid) |
PLA | = | poly(lactic acid) |
PLGA | = | poly (DL-lactide-co-glycolide) |
RES | = | reticuloendothelial system |
SIR | = | sirolimus |
TNF-α | = | tumour necrosis factor α |
Disclosure statement
No potential conflict of interest was reported by the authors.
References
- Sehgal SN, Baker H, Vezina C. Rapamycin (AY-22,989), a new antifungal antibiotic. II. Fermentation, isolation and characterization. J Antibiot (Tokyo). 1975;28:727–732.
- Park SR, Yoo YJ, Ban YH, et al. Biosynthesis of rapamycin and its regulation: past achievements and recent progress. J Antibiot (Tokyo). 2010;63:434–441.
- Sehgal SN. Sirolimus: its discovery, biological properties, and mechanism of action. Transplant Proc. 2003;35:7s–14s.
- Dowling RJ, Topisirovic I, Fonseca BD, et al. Dissecting the role of mTOR: lessons from mTOR inhibitors. Biochim Biophys Acta. 2010;1804:433–439.
- Baretic D, Williams RL. The structural basis for mTOR function. Semin Cell Dev Biol. 2014;36:91–101.
- MacDonald A, Scarola J, Burke JT, et al. Clinical pharmacokinetics and therapeutic drug monitoring of sirolimus. Clin Ther. 2000;22 Suppl B:B101–B121.
- Mahalati K, Kahan BD. Clinical pharmacokinetics of sirolimus. Clin Pharmacokinet. 2001;40:573–585.
- Picard N, Djebli N, Sauvage FL, et al. Metabolism of sirolimus in the presence or absence of cyclosporine by genotyped human liver microsomes and recombinant cytochromes P450 3A4 and 3A5. Drug Metab Dispos. 2007;35:350–355.
- Shihab F, Christians U, Smith L, et al. Focus on mTOR inhibitors and tacrolimus in renal transplantation: pharmacokinetics, exposure-response relationships, and clinical outcomes. Transpl Immunol. 2014;31:22–32.
- Augustine JJ, Bodziak KA, Hricik DE. Use of sirolimus in solid organ transplantation. Drugs. 2007;67:369–391.
- Cheng-Lai A, Frishman WH. Sirolimus-eluting coronary stents: novel devices for the management of coronary artery disease. Am J Ther. 2004;11:218–228.
- Piscione F, Piccolo R, Cassese S, et al. Clinical impact of sirolimus-eluting stent in ST-segment elevation myocardial infarction: a meta-analysis of randomized clinical trials. Catheter Cardiovasc Interv. 2009;74:323–332.
- Hartford CM, Ratain MJ. Rapamycin: something old, something new, sometimes borrowed and now renewed. Clin Pharmacol Ther. 2007;82:381–388.
- Paghdal KV, Schwartz RA. Sirolimus (rapamycin): from the soil of Easter Island to a bright future. J Am Acad Dermatol. 2007;57:1046–1050.
- Li J, Kim SG, Blenis J. Rapamycin: one drug, many effects. Cell Metab. 2014;19:373–379.
- Cutler C, Antin JH. Sirolimus for GVHD prophylaxis in allogeneic stem cell transplantation. Bone Marrow Transplant. 2004;34:471–476.
- Bride KL, Vincent T, Smith-Whitley K, et al. Sirolimus is effective in relapsed/refractory autoimmune cytopenias: results of a prospective multi-institutional trial. Blood. 2016;127:17–28.
- Ormerod AD, Shah SA, Copeland P, et al. Treatment of psoriasis with topical sirolimus: preclinical development and a randomized, double-blind trial. Br J Dermatol. 2005;152:758–764.
- Heredia A, Amoroso A, Davis C, et al. Rapamycin causes down-regulation of CCR5 and accumulation of anti-HIV beta-chemokines: an approach to suppress R5 strains of HIV-1. Proc Natl Acad Sci USA. 2003;100:10411–10416.
- Bove J, Martinez-Vicente M, Vila M. Fighting neurodegeneration with rapamycin: mechanistic insights. Nat Rev Neurosci. 2011;12:437–452.
- Keogh A, Richardson M, Ruygrok P, et al. Sirolimus in de novo heart transplant recipients reduces acute rejection and prevents coronary artery disease at 2 years: a randomized clinical trial. Circulation. 2004;110:2694–2700.
- Nguyen QD, Merrill PT, Clark WL, et al. Intravitreal sirolimus for noninfectious uveitis: a phase III sirolimus study assessing double-masKed uveitis TReAtment (SAKURA). Ophthalmology. 2016;123:2413–2423.
- Pleyer U, Thurau SR. Sirolimus for the treatment of noninfectious uveitis. Expert Opin Pharmacother. 2016;17:127–135.
- Stallone G, Infante B, Grandaliano G, et al. Kaposi's sarcoma and mTOR: a crossroad between viral infection neoangiogenesis and immunosuppression. Transpl Int. 2008;21:825–832.
- Campbell SB, Walker R, Tai SS, et al. Randomized controlled trial of sirolimus for renal transplant recipients at high risk for nonmelanoma skin cancer. Am J Transplant. 2012;12:1146–1156.
- Um SH, Frigerio F, Watanabe M, et al. Absence of S6K1 protects against age- and diet-induced obesity while enhancing insulin sensitivity. Nature. 2004;431:200–205.
- Ong PS, Wang LZ, Dai X, et al. Judicious toggling of mTOR activity to combat insulin resistance and cancer: current evidence and perspectives. Front Pharmacol. 2016;7:395.
- Kim EJ, Jeong JH, Bae S, et al. mTOR inhibitors radiosensitize PTEN-deficient non-small-cell lung cancer cells harboring an EGFR activating mutation by inducing autophagy. J Cell Biochem. 2013;114:1248–1256.
- Nagata Y, Takahashi A, Ohnishi K, et al. Effect of rapamycin, an mTOR inhibitor, on radiation sensitivity of lung cancer cells having different p53 gene status. Int J Oncol. 2010;37:1001–1010.
- Shinohara ET, Maity A, Jha N, et al. Sirolimus as a potential radiosensitizer in squamous cell cancer of the head and neck. Head Neck. 2009;31:406–411.
- Moes DJ, Guchelaar HJ, de Fijter JW. Sirolimus and everolimus in kidney transplantation. Drug Discov Today. 2015;20:1243–1249.
- Simamora P, Alvarez JM, Yalkowsky SH. Solubilization of rapamycin. Int J Pharm. 2001;213:25–29.
- Yanez JA, Forrest ML, Ohgami Y, et al. Pharmacometrics and delivery of novel nanoformulated PEG-b-poly(epsilon-caprolactone) micelles of rapamycin. Cancer Chemother Pharmacol. 2008;61:133–144.
- Pathak K, Raghuvanshi S. Oral bioavailability: issues and solutions via nanoformulations. Clin Pharmacokinet. 2015;54:325–357.
- Chen H, Khemtong C, Yang X, et al. Nanonization strategies for poorly water-soluble drugs. Drug Discov Today. 2011;16:354–360.
- Aberoumandi SM, Mohammadhosseini M, Abasi E, et al. An update on applications of nanostructured drug delivery systems in cancer therapy: a review. Artif Cells Nanomed Biotechnol. 2017;45:1–11.
- Panahi Y, Farshbaf M, Mohammadhosseini M, et al. Recent advances on liposomal nanoparticles: synthesis, characterization and biomedical applications. Artif Cells Nanomed Biotechnol. 2017;45:788–799.
- Daraee H, Etemadi A, Kouhi M, et al. Application of liposomes in medicine and drug delivery. Artif Cells Nanomed Biotechnol. 2016;44:381–391.
- Alavi S, Haeri A, Dadashzadeh S. Utilization of chitosan-caged liposomes to push the boundaries of therapeutic delivery. Carbohydr Polym. 2017;157:991–1012.
- Haeri A, Sadeghian S, Rabbani S, et al. PEGylated estradiol benzoate liposomes as a potential local vascular delivery system for treatment of restenosis. J Microencapsul. 2012;29:83–94.
- Maeda H. Vascular permeability in cancer and infection as related to macromolecular drug delivery, with emphasis on the EPR effect for tumor-selective drug targeting. Proc Jpn Acad Ser B Phys Biol Sci. 2012;88:53–71.
- Suk JS, Xu Q, Kim N, et al. PEGylation as a strategy for improving nanoparticle-based drug and gene delivery. Adv Drug Deliv Rev. 2016;99:28–51.
- Marques-Gallego P, de Kroon AI. Ligation strategies for targeting liposomal nanocarriers. Biomed Res Int. 2014;2014:129458.
- Haeri A, Sadeghian S, Rabbani S, et al. Use of remote film loading methodology to entrap sirolimus into liposomes: preparation, characterization and in vivo efficacy for treatment of restenosis. Int J Pharm. 2011;414:16–27.
- Haeri A, Sadeghian S, Rabbani S, et al. Physicochemical characteristics of liposomes are decisive for their antirestenosis efficacy following local delivery. Nanomedicine (Lond). 2017;12:131–145.
- Haeri A, Sadeghian S, Rabbani S, et al. Effective attenuation of vascular restenosis following local delivery of chitosan decorated sirolimus liposomes. Carbohydr Polym. 2017;157:1461–1469.
- Pang X, Wang J, Tan X, et al. Dual-modal imaging-guided theranostic nanocarriers based on indocyanine green and mTOR inhibitor rapamycin. ACS Appl Mater Interfaces. 2016;8:13819–13829.
- Ghanbarzadeh S, Khorrami A, Mohamed Khosroshahi L, et al. Fusogenic pH sensitive liposomal formulation for rapamycin: improvement of antiproliferative effect. Pharm Biol. 2014;52:848–854.
- Ghanbarzadeh S, Arami S, Pourmoazzen Z, et al. Improvement of the antiproliferative effect of rapamycin on tumor cell lines by poly (monomethylitaconate)-based pH-sensitive, plasma stable liposomes. Colloids Surf B Biointerfaces. 2014;115:323–330.
- Alemdar AY, Sadi D, McAlister VC, et al. Liposomal formulations of tacrolimus and rapamycin increase graft survival and fiber outgrowth of dopaminergic grafts. Cell Transplant. 2004;13:263–271.
- Rouf MA, Vural I, Renoir JM, et al. Development and characterization of liposomal formulations for rapamycin delivery and investigation of their antiproliferative effect on MCF7 cells. J Liposome Res. 2009;19:322–331.
- Eloy JO, Petrilli R, Topan JF, et al. Co-loaded paclitaxel/rapamycin liposomes: development, characterization and in vitro and in vivo evaluation for breast cancer therapy. Colloids Surf B Biointerfaces. 2016;141:74–82.
- Haeri A, Sadeghian S, Rabbani S, et al. Sirolimus-loaded stealth colloidal systems attenuate neointimal hyperplasia after balloon injury: a comparison of phospholipid micelles and liposomes. Int J Pharm. 2013;455:320–330.
- Zhang W, Sun Y, Li Y, et al. Preparation and influencing factors of sirolimus liposome by supercritical fluid. Artif Cells Blood Substit Immobil Biotechnol. 2012;40:62–65.
- Buech G, Bertelmann E, Pleyer U, et al. Formulation of sirolimus eye drops and corneal permeation studies. J Ocul Pharmacol Ther. 2007;23:292–303.
- Linares-Alba MA, Gomez-Guajardo MB, Fonzar JF, et al. Preformulation studies of a liposomal formulation containing sirolimus for the treatment of dry eye disease. J Ocul Pharmacol Ther. 2016;32:11–22.
- Narvekar M, Xue HY, Eoh JY, et al. Nanocarrier for poorly water-soluble anticancer drugs–barriers of translation and solutions. AAPS PharmSciTech. 2014;15:822–833.
- Lai P, Daear W, Lobenberg R, et al. Overview of the preparation of organic polymeric nanoparticles for drug delivery based on gelatine, chitosan, poly(d,l-lactide-co-glycolic acid) and polyalkylcyanoacrylate. Colloids Surf B Biointerfaces. 2014;118:154–163.
- Afsharzadeh M, Hashemi M, Mokhtarzadeh A, et al. Recent advances in co-delivery systems based on polymeric nanoparticle for cancer treatment. Artif Cells Nanomed Biotechnol. 2017. DOI:https://doi.org/10.1080/21691401.2017.1376675
- Frank LA, Contri RV, Beck RC, et al. Improving drug biological effects by encapsulation into polymeric nanocapsules. Wires Nanomed Nanobiotechnol. 2015;7:623–639.
- Vauthier C, Bouchemal K. Methods for the preparation and manufacture of polymeric nanoparticles. Pharm Res. 2009;26:1025–1058.
- Shi X, Chen G, Guo LW, et al. Periadventitial application of rapamycin-loaded nanoparticles produces sustained inhibition of vascular restenosis. PLoS One. 2014;9:e89227.
- Katiyar SS, Muntimadugu E, Rafeeqi TA, et al. Co-delivery of rapamycin- and piperine-loaded polymeric nanoparticles for breast cancer treatment. Drug Deliv. 2016;23:2608–2616.
- Acharya S, Dilnawaz F, Sahoo SK. Targeted epidermal growth factor receptor nanoparticle bioconjugates for breast cancer therapy. Biomaterials. 2009;30:5737–5750.
- Zou J, Zhang X, Yang H, et al. Rapamycin-loaded nanoparticles for inhibition of neointimal hyperplasia in experimental vein grafts. Ann Vasc Surg. 2011;25:538–546.
- Das S, Haddadi A, Veniamin S, et al. Delivery of rapamycin-loaded nanoparticle down regulates ICAM-1 expression and maintains an immunosuppressive profile in human CD34+ progenitor-derived dendritic cells. J Biomed Mater Res A. 2008;85:983–992.
- Haddadi A, Elamanchili P, Lavasanifar A, et al. Delivery of rapamycin by PLGA nanoparticles enhances its suppressive activity on dendritic cells. J Biomed Mater Res A. 2008;84:885–898.
- Moeller S, Kegler R, Sternberg K, et al. Influence of sirolimus-loaded nanoparticles on physiological functions of native human polymorphonuclear neutrophils. Nanomedicine. 2012;8:1293–1300.
- Zago AC, Raudales JC, Attizzani G, et al. Local delivery of sirolimus nanoparticles for the treatment of in-stent restenosis. Cathet Cardiovasc Intervent. 2013;81:E124–E129.
- Yuan XB, Yuan YB, Jiang W, et al. Preparation of rapamycin-loaded chitosan/PLA nanoparticles for immunosuppression in corneal transplantation. Int J Pharm. 2008;349:241–248.
- Shah M, Edman MC, Janga SR, et al. A rapamycin-binding protein polymer nanoparticle shows potent therapeutic activity in suppressing autoimmune dacryoadenitis in a mouse model of Sjogren’s syndrome. J Control Release. 2013;171:269–279.
- Zhao Y, Zhang T, Duan S, et al. CD44-tropic polymeric nanocarrier for breast cancer targeted rapamycin chemotherapy. Nanomedicine. 2014;10:1221–1230.
- Bisht S, Feldmann G, Koorstra JB, et al. In vivo characterization of a polymeric nanoparticle platform with potential oral drug delivery capabilities. Mol Cancer Ther. 2008;7:3878–3888.
- Zhang Z, Xu L, Chen H, et al. Rapamycin-loaded poly(epsilon-caprolactone)-poly(ethylene glycol)-poly(epsilon-caprolactone) nanoparticles: preparation, characterization and potential application in corneal transplantation. J Pharm Pharmacol. 2014;66:557–563.
- Lu XY, Li MC, Zhu XL, et al. Microbial synthesized biodegradable PHBHHxPEG hybrid copolymer as an efficient intracellular delivery nanocarrier for kinase inhibitor. BMC Biotechnol. 2014;14:4.
- Reddy MK, Vasir JK, Sahoo SK, et al. Inhibition of apoptosis through localized delivery of rapamycin-loaded nanoparticles prevented neointimal hyperplasia and reendothelialized injured artery. Circ Cardiovasc Interv. 2008;1:209–216.
- Zhang Y, Huang Y, Li S. Polymeric micelles: nanocarriers for cancer-targeted drug delivery. AAPS PharmSciTech. 2014;15:862–871.
- Oba M. Study on development of polymeric micellar gene carrier and evaluation of its functionality. Biol Pharm Bull. 2013;36:1045–1051.
- Jones MC. Thinking outside the ‘block’: alternative polymer compositions for micellar drug delivery. Curr Top Med Chem. 2015;15:2254–2266.
- Shin HC, Alani AW, Cho H, et al. A 3-in-1 polymeric micelle nanocontainer for poorly water-soluble drugs. Mol Pharm. 2011;8:1257–1265.
- Hasenstein JR, Shin HC, Kasmerchak K, et al. Antitumor activity of Triolimus: a novel multidrug-loaded micelle containing Paclitaxel, Rapamycin, and 17-AAG. Mol Cancer Ther. 2012;11:2233–2242.
- Shin HC, Cho H, Lai TC, et al. Pharmacokinetic study of 3-in-1 poly(ethylene glycol)-block-poly(D, L-lactic acid) micelles carrying paclitaxel, 17-allylamino-17-demethoxygeldanamycin, and rapamycin. J Control Release. 2012;163:93–99.
- Zeng S, Xiong MP. Trilayer micelles for combination delivery of rapamycin and siRNA targeting Y-box binding protein-1 (siYB-1). Biomaterials. 2013;34:6882–6892.
- Kim MS, Kim JS, Cho WK, et al. Enhanced solubility and oral absorption of sirolimus using D-alpha-tocopheryl polyethylene glycol succinate micelles. Artif Cells Nanomed Biotechnol. 2013;41:85–91.
- Tian W, Liu J, Guo Y, et al. Self-assembled micelles of amphiphilic PEGylated rapamycin for loading paclitaxel and resisting multidrug resistant cancer cellsdaggerElectronic supplementary information (ESI) available: chemicals and reagents, detailed experimental procedures for materials synthesis, characterization, cellular evaluations and supporting figures and tables. J Mater Chem B Mater Biol Med. 2015;3:1204–1207.
- Dane KY, Nembrini C, Tomei AA, et al. Nano-sized drug-loaded micelles deliver payload to lymph node immune cells and prolong allograft survival. J Control Release. 2011;156:154–160.
- O'Neil CP, van der Vlies AJ, Velluto D, et al. Extracellular matrix binding mixed micelles for drug delivery applications. J Control Release. 2009;137:146–151.
- Nadig SN, Dixit SK, Levey N, et al. Immunosuppressive nano-therapeutic micelles downregulate endothelial cell inflammation and immunogenicity. RSC Adv. 2015;5:43552–43562.
- Elsaid N, Somavarapu S, Jackson TL. Cholesterol-poly(ethylene) glycol nanocarriers for the transscleral delivery of sirolimus. Exp Eye Res. 2014;121:121–129.
- Elsaid N, Jackson TL, Gunic M, et al. Positively charged amphiphilic chitosan derivative for the transscleral delivery of rapamycin. Invest Ophthalmol Vis Sci. 2012;53:8105–8111.
- Lu W, Li F, Mahato RI. Poly(ethylene glycol)-block-poly(2-methyl-2-benzoxycarbonyl-propylene carbonate) micelles for rapamycin delivery: in vitro characterization and biodistribution. J Pharm Sci. 2011;100:2418–2429.
- Khondee S, Rabinsky EF, Owens SR, et al. Targeted therapy of colorectal neoplasia with rapamycin in peptide-labeled pegylated octadecyl lithocholate micelles. J Control Release. 2015;199:114–121.
- Leone F, Cavalli R. Drug nanosuspensions: a ZIP tool between traditional and innovative pharmaceutical formulations. Expert Opin Drug Deliv. 2015;12:1607–1625.
- Gao L, Liu G, Ma J, et al. Drug nanocrystals: in vivo performances. J Control Release. 2012;160:418–430.
- Zhou Y, Du J, Wang L, et al. State of the art of nanocrystals technology for delivery of poorly soluble drugs. J Nanopart Res. 2016;18:257.
- Junghanns JU, Muller RH. Nanocrystal technology, drug delivery and clinical applications. Int J Nanomedicine. 2008;3:295–309.
- Dou Y, Guo J, Chen Y, et al. Sustained delivery by a cyclodextrin material-based nanocarrier potentiates antiatherosclerotic activity of rapamycin via selectively inhibiting mTORC1 in mice. J Control Release. 2016;235:48–62.
- Ghanbarzadeh S, Khorrami A, Pourmoazzen Z, et al. Plasma stable, pH-sensitive non-ionic surfactant vesicles simultaneously enhance antiproliferative effect and selectivity of Sirolimus. Pharm Dev Technol. 2015;20:279–287.
- Dilnawaz F, Singh A, Mohanty C, et al. Dual drug loaded superparamagnetic iron oxide nanoparticles for targeted cancer therapy. Biomaterials. 2010;31:3694–3706.
- Liu Q, Zhang J, Sun W, et al. Delivering hydrophilic and hydrophobic chemotherapeutics simultaneously by magnetic mesoporous silica nanoparticles to inhibit cancer cells. Int J Nanomedicine. 2012;7:999–1013.
- Bibee KP, Cheng YJ, Ching JK, et al. Rapamycin nanoparticles target defective autophagy in muscular dystrophy to enhance both strength and cardiac function. FASEB J. 2014;28:2047–2061.
- Cho W, Kim MS, Kim JS, et al. Optimized formulation of solid self-microemulsifying sirolimus delivery systems. Int J Nanomedicine. 2013;8:1673–1682.
- Gasper WJ, Jimenez CA, Walker J, et al. Adventitial nab-rapamycin injection reduces porcine femoral artery luminal stenosis induced by balloon angioplasty via inhibition of medial proliferation and adventitial inflammation. Circ Cardiovasc Interv. 2013;6:701–709.
- Kim MS, Kim JS, Park HJ, et al. Enhanced bioavailability of sirolimus via preparation of solid dispersion nanoparticles using a supercritical antisolvent process. Int J Nanomedicine. 2011;6:2997–3009.
- Solymosi T, Angi R, Basa DO, et al. Sirolimus formulation with improved pharmacokinetic properties produced by a continuous flow method. Eur J Pharm Biopharm. 2015;94:135–140.
- Sobhani H, Tarighi P, Ostad SN, et al. Formulation development and toxicity assessment of triacetin mediated nanoemulsions as novel delivery systems for rapamycin. Iran J Pharm Res. 2015;14(Suppl):3–21.
- Wang J, Guo F, Yu M, et al. Rapamycin/DiR loaded lipid-polyaniline nanoparticles for dual-modal imaging guided enhanced photothermal and antiangiogenic combination therapy. J Control Release. 2016;237:23–34.
- Visweswaran GR, Gholizadeh S, Ruiters MH, et al. Targeting rapamycin to podocytes using a vascular cell adhesion molecule-1 (VCAM-1)-harnessed SAINT-based lipid carrier system. PLoS One. 2015;10:e0138870.