Abstract
In this study, we report the synthesis, characterization and utilization of 99mTc-labelled polyethylenimine-entrapped gold nanoparticles (99mTc-Au-PENPs) for dual mode single-photon emission computed tomography/computed tomography (SPECT/CT) imaging applications. Polyethylenimine (PEI) was selected as a platform to conjugate with diethylene triamine pentacetate acid (DTPA) and polyethylene glycol monomethyl ether to synthesize Au PENPs, followed by acetylation or hydroxylation modification of the remaining PEI surface amine groups and radiolabelling of 99mTc. The generated multifunctional 99mTc-Au-PENPs with different surface groups (acetyl or hydroxyl) were characterized via different methods. The Au PENPs before 99mTc labelling are colloidally stable, haemocompatibility and noncytotoxic at an Au concentration up to 100 μM. The 99mTc-labelled Au PENPs exhibit high radiochemical purity, good stability and SPECT/CT imaging performance of different organs and lymph node. The designed strategy to use the radionuclide labelling technique and PEI-facilitated versatile nanoplatform may be extended to develop various novel nanoprobes for precision imaging applications.
Introduction
The advent of molecular imaging technique has led to unprecedented progress in non-invasive visualization, characterization and quantification of biologic processes at molecular and cellular levels [Citation1–3]. Nuclear medicine imaging, particularly positron emission tomography (PET) and single-photon emission computed tomography (SPECT), has been playing a crucial role in the development of molecular imaging [Citation4,Citation5]. With the advantage of high sensitivity, PET and SPECT imaging can offer physiologic, metabolic and functional information, which is superior to the standard magnetic resonance (MR) and computed tomography (CT) imaging. Although relatively low resolution and lack of anatomical information have always been the limitations of nuclear medicine imaging, fusion imaging technique that combines functional and anatomic imaging modalities can integrate advantages and cover shortages of each imaging modality [Citation6–8]. The developed PET/MR, PET/CT and SPECT/CT hybrid imaging techniques have gained wide acceptance as powerful tools in basic research and clinical applications. However, few new imaging agents have been provided for clinical purposes during the last decade, in particular, multifunctional radiolabelled agents for these hybrid imaging techniques. Therefore, the present research efforts are mainly focused on the development of multifunctional imaging probes in this field.
As the development of nanotechnology, nanoparticles (NPs) have been commended as promising platforms for diagnosis and treatment of diseases [Citation9–15]. For CT imaging, various NP systems, especially gold (Au) NPs, have been explored as effective contrast agents and have displayed great advantages compared with the clinical iodine-based contrast agents, such as better imaging performance and biocompatibility, longer blood circulation time and controllable behaviours [Citation16–23]. On the other hand, 99mTc is an ideal radioisotope for SPECT imaging due to its favourable low-energy γ-ray (140 keV), appropriate half-life (6.02 h), low cost and commercial availability. Numerous 99mTc-labelled NPs have been developed as molecular probes for SPECT imaging applications in cancer, sentinel lymph node (SLN) and other biological systems [Citation24–27]. Notably, unique features of nanotechnology allow integrating multiple imaging modalities in one particle system to form multifunctional NPs for multimodal imaging [Citation28–30]. Polyethylenimine (PEI) with the superiority of high-density amines has been frequently served as a template for imaging, drug and gene delivery [Citation2,Citation21,Citation22,Citation31–38]. In our previous study, polyethylene glycol (PEG)-functionalized PEI-entrapped Au NPs (Au PENPs) have been successfully utilized for blood pool and tumour CT imaging in vivo [Citation21,Citation22]. Interestingly, the PEI surface might be further modified with bifunctional chelators such as diethylene triamine pentacetate acid (DTPA) to radiolabel 99mTc for SPECT imaging. Furthermore, since slight physicochemical differences in NPs surface may dramatically influence their biological behaviours in vivo, the Au PENPs with different surface modifications may offer diverse imaging information. Therefore, the previous successes of using PEI as a platform to build up contrast agents encourage us to incorporate Au and 99mTc into PEI-based NPs for SPECT/CT imaging applications.
Herein, we designed and synthesized 99mTc-labelled Au PENPs as dual-mode contrast agents for SPECT/CT imaging (Scheme 1). PEI was firstly modified with DTPA and mPEG to prepare Au PENPs. The remaining amine groups of PEI were acetylated or hydroxylated by acetic anhydride (Ac2O) or glycidol, respectively. Followed by radiolabelling with 99mTc, 99mTc-Au-PENPs with different surface groups (99mTc-Au-Ac-PENPs and 99mTc-Au-Gly-PENPs) were developed. The gained Au PENPs before and after 99mTc radiolabelling were thoroughly characterized via different techniques. The cytotoxicity of the unlabelled Au PENPs was evaluated via cell viability assay in vitro. The radiostability of 99mTc-labelled Au PENPs was analyzed by evaluation of their radiochemical purities at different time points. Finally, the imaging performance of the 99mTc-Au-PENPs was assessed via SPECT/CT imaging of different organs and SLN in vivo. To our knowledge, this is the first report related to the development of the PEI-based platform with different surface groups for SPECT/CT imaging applications.
Materials and methods
Materials
PEI (Branched, Mw = 25,000), glycidol, DTPA dianhydride, 1-ethyl-3-(3-(dimethylamino)propyl) carbodiimide hydrochloride (EDC · HCl), stannous chlorides, sodium borohydride (NaBH4) and 3-(4,5-dimethylthiazol-2-yl)-2,5-diphenyltetrazolium bromide (MTT) were supplied by Sigma-Aldrich (St. Louis, MO). PEG monomethyl ether with one end of the carboxyl group (mPEG-COOH, Mw = 5000) and phosphate-buffered saline (PBS) were purchased from Shanghai Yanyi Biotechnology Corporation (Shanghai, China). 99mTc-pertechnetate (Na99mTcO4) was purchased from Shanghai GMS Pharmaceutical Co., Ltd. (Shanghai, China). Acetic anhydride (Ac2O), HAuCl4·4H2O, triethylamine all other chemicals and solvents were supplied by Sinopharm Chemical Reagent Co., Ltd. (Shanghai, China). SKOV-3 cells (human ovarian carcinoma cell line) were supplied by Institute of Biochemistry and Cell Biology (the Chinese Academy of Sciences, Shanghai, China). Disposable PD-10 desalting columns and Omnipaque (iohexol 300) were obtained from GE Healthcare (Shanghai, China).
Surface modification of PEI with DTPA and mPEG-COOH
DTPA dianhydride (2.2 mg) dissolved in 2 ml of water was dropwise added to a PEI aqueous solution (2 mg/ml, 12 ml) under vigorous magnetic stirring. The reaction mixture was incubated at room temperature for 8 h to yield the raw product of PEI-DTPA. Then the EDC-activated mPEG-COOH was reacted with the PEI-DTPA in aqueous solution under continuous stirring. The reaction was stopped after 48 h to gain the product of PEI-DTPA-mPEG.
Synthesis of Au PENPs
The obtained PEI-DTPA-mPEG was used as a template to synthesize Au PENPs. The synthetic process and surface modification of Au PENPs were performed according to our previous work [Citation18–22].
Synthesis of 99mTc-Au-PENPs
The Au PENPs were labelled with 99mTc through DTPA ligands according to protocols described in the literature [Citation25,Citation26].
Micro-SPECT/CT imaging in vivo
All animal experiments were approved by the ethical committee of Shanghai General Hospital and performed according to protocols and guideline of Shanghai General Hospital and also in accordance of the policy of the National Ministry of Health. In the micro-SPECT/CT imaging study, 99mTc-Au-Ac-PENPs or 99mTc-Au-Gly-PENPs (0.20 ml, [99mTc] = 370 MBq/ml, [Au] = 0.08 M) saline solution was intravenously administrated into each ICR mouse. SPECT/CT scanning was carried out at 0.5, 1, and 2 h postinjection by NanoSPECT/CT InVivo Animal Imager (Bioscan Inc., Tucson, AZ). Mice were anaesthetized with 2% isoflurane before SPECT/CT imaging.
In vivo sentinel lymph node SPECT/CT imaging
For SLN SPECT/CT imaging, New Zealand white rabbits (Sino-British SIPPR/BK Lab Animal Ltd., Shanghai, China) were firstly anaesthetized through intraperitoneal injection of pentobarbital sodium (40 mg/kg for each rabbit). 99mTc-Au-Gly-PENPs or 99mTc-Au-Ac-PENPs (0.50 ml, [Au] = 0.08 M, [99mTc] = 370 MBq/ml) were injected intradermally into a rabbit’s left and right paws, respectively. At 0.5, 1, and 4 h postinjection, SPECT scanning was performed by an Infinia SPECT scanner equipped with a Xeleris Workstation and Low Energy General Purpose collimator (GE Medical Systems, Milwaukee, WI). At the same time points, CT imaging was carried out by a GE Discovery STE PET/CT system (GE Medical Systems) with a tube voltage of 100 kV, an electrical current of 220 mA, and a slice thickness of 1.25 mm.
Results
Synthesis and characterization of 99mTc-Au-PENPs
As represented in Scheme 1, DTPA and mPEG-COOH were first modified on the surface of PEI, and then the functional PEI was used as a template to form Au PENPs via sodium borohydride reduction chemistry. Then, acetylation or hydroxylation of the remaining PEI surface amine groups was carried out to render the formed Au PENPs with different surface groups. Finally, 99mTc was chelated with the DTPA on the PEI surface, and 99mTc-Au-PENPs as dual-mode SPECT/CT contrast agents with different surface modifications were constructed.
1H NMR shows that the number of DTPA and mPEG modified with each PEI is estimated to be 6.9 and 18.4, respectively by an indirect method according to our previous work [Citation18,Citation19,Citation39]. As shown in Figure S1a (Supporting information), the peaks between 2.1 and 3.0 ppm are associated with the -CH2- signals of PEI, while chemical shifts at 3.2 and 3.4 ppm relate to the -CH2- peaks of DTPA [Citation21,Citation22]. Moreover, the surface potential of PEI (51.4 ± 7.3 mV) reduces to 37.2 ± 5.4 mV due to the DTPA modification, confirming the success of the DTPA surface modification. As shown in Figure S1b, the additional peaks at 3.5 and 3.2 ppm are related to ethylene backbone and methoxyl protons of mPEG. Compared with PEI-DTPA, a remarkably reduced surface potential of PEI-DTPA-mPEG (21.3 ± 6.2 mV) was observed due to the PEGylation. After the surface acetylation and hydroxylation, the surface potentials of Au-Ac-PENPs (1.6 ± 0.9 mV) and Au-Gly-PENPs (1.8 ± 0.5 mV) are almost neutral. The 1H NMR spectra (Figure S1c and d) also indicates the successful surface acetylation and hydroxylation, in accordance with our previous report [Citation18–20].
The size and morphology of the Au PENPs were observed by TEM (). Both Au-Ac-PENPs and Au-Gly-PENPs have a spherical shape with a mean diameter of 2.8 and 2.7 nm, respectively. Their crystalline phase was further affirmed by selected area electron diffraction (SAED). The (111), (200), (220) and (311) rings in the SAED patterns show their typical face-centred-cubic crystal structures (). UV-vis spectra () show the prominent surface plasma resonance (SPR) peaks of Au-Ac-PENPs and Au-Gly-PENPs, confirming the Au NP formation. However, the SPR peaks for both Au PENPs are notably different (513 nm for Au-Ac-PENPs and 500 nm for Au-Gly-PENPs, respectively), which may be due to the different state of aggregation in aqueous solution induced by the different surface modifications. The hydrodynamic sizes of the Au PENPs dispersed in water were also measured by DLS to be 83.4 ± 7.4 nm for Au-Ac-PENPs and 22.1 ± 2.6 nm for Au-Gly-PENPs, respectively. The actual Au loading in the PEI was measured to be 191 and 196 Au atom per PEI by ICP-OES for the Au-Ac-PENPs and Au-Gly-PENPs, respectively, which is consistent with the initial Au salt/PEI molar ratio (200:1) due to the complete reduction of Au salt for both Au PENPs.
Figure 1. (a,c) TEM images, (b,d) size distribution, (e,f) selected area electron diffraction pattern and (G,H) UV-vis spectra of Au-Ac-PENPs (a, b, c, and g) and Au-Gly-PENPs (c, d, f, and h), respectively.
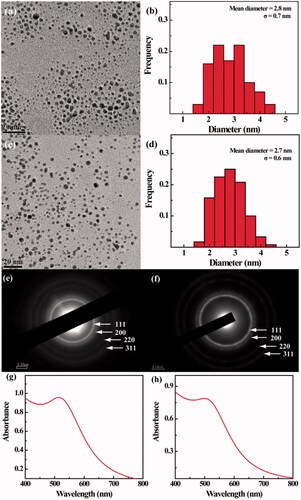
For 99mTc radiolabelling, the radiochemical yield was calculated to be above 90% for both Au-Ac-PENPs and Au-Gly-PENPs. After purification by PD-10 desalting columns, the radiochemical purities of the radiolabelled NPs were over 95%.
Blood compatibility, cell viability and in vivo toxicology investigation
Haemolysis assay was performed to estimate the blood compatibility of the Au PENPs. The results show that the haemolysis percentages of both Au-Ac-PENPs and Au-Gly-PENPs at a concentration up to 400 μg/ml are all less than 4% (). Both samples do not show any appreciable cytotoxicity to SKOV-3 cells at the Au concentration as high as 100 μM for 24 h when compared with control cells treated with PBS ().
Figure 2. (a) Haemolytical activity of Au-Ac-PENPs and Au-Gly-PENPs under different concentrations. (b) MTT assay of the viability of SKOV-3 cells treatment with Au-Ac-PENPs and Au-Gly-PENPs at the Au concentrations of 0–100 μM for 24 h. (c) Haematology and blood biochemistry data of the mice treated with saline (control group), Au-Ac-PENPs, and Au-Gly-PENPs.
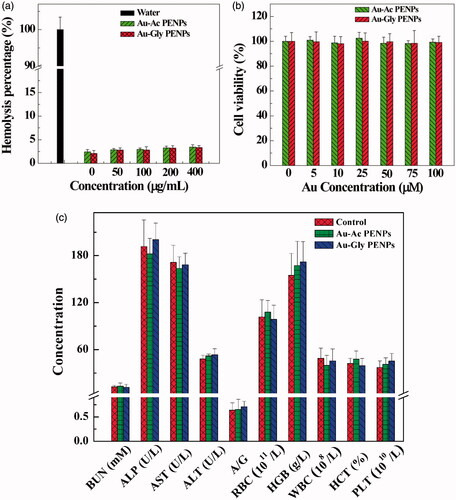
The biosafety of the formed nanomaterials in vivo was investigated. Body weight measurements, behaviour observations and histological examinations were performed to confirm their safety in mice in vivo. As shown in , mice in the two test groups and the control group display similar trends in their health, diet, behaviour, neurological status and body weight change during the studied period. In serum biochemistry analyze and haematological assessments, all biochemistry parameters of both test and control groups keep constant in the normal range in the same time (). H&E stained organ slices in both groups show the similar morphology of the organs and no perceptible lesion or hydropic damage is found (Figure S5, Supporting Information). All these data confirmed the biosafety of the Au PENPs.
In vivo SPECT imaging
The whole body micro-SPECT/CT images were gained after intravenous injection of 99mTc-Au-Ac-PENPs and 99mTc-Au-Gly-PENPs, respectively. As shown in , 99mTc-Au-Ac-PENPs are largely accumulated in the liver and spleen within 2 h postinjection. A portion of 99mTc-Au-Ac-PENPs can be detected in the lung and kidneys at 0.5 h postinjection (), and the accumulation becomes higher at 1 h postinjection (). Soon afterwards, the 99mTc-Au-Ac-PENPs are gradually cleared from the lung and kidneys, and only the liver and spleen can be easily observed at 2 h postinjection (). Differently, 99mTc-Au-Gly-PENPs show the appearance in the blood as indicated by the brighter zones of the jugular vein, heart, kidneys, and postcaval vein at 0.5 h postinjection, and only weak SPECT signals can be found in the liver (). After that, the accumulation in the kidneys and bladder continues to increase (), displaying that the formed 99mTc-Au-Gly-PENPs are excreted through urinary system with little accumulation in the liver and spleen ().
Figure 3. SPECT/CT images of a mouse lung, liver, and bladder at (a) 0.5 h, (b)1 h, and (c) 2 h post intravenous injection of 99mTc-Au-Ac-PENPs; SPECT/CT images of a mouse heart, liver, spleen, kidneys, postcaval vein, and bladder at (d) 0.5 h, (e)1 h, and (f) 2 h post intravenous injection of 99mTc-Au-Gly-PENPs.
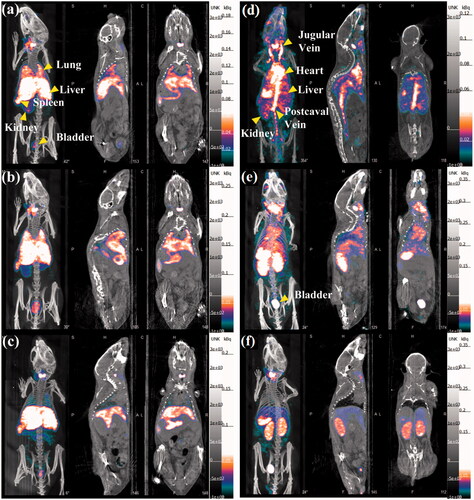
To quantitatively analyze the contrast effect of 99mTc-Au-PENPs, the relative signal intensities of regions of interest (ROIs) were measured (). Similar to the results of SPECT/CT imaging, 99mTc-Au-Ac-PENPs are primarily found in liver, spleen, and lung within 1 h postinjection (). Subsequently, the signal intensity of liver and spleen only slightly decreases, while that of lung sharply reduces from 0.0057 μCi/mm3 (1 h) to 0.0008 μCi/mm3 (2 h). Diversely, the signal intensity of bladder increases to 0.0023 μCi/mm3 (2 h). In contrast, 99mTc-Au-Gly-PENPs mainly appear in postcaval vein and heart within 0.5 h after injection (). With the clearance from blood, the signal intensities of postcaval vein and heart reduce significantly from 0.0065 and 0.0068 μCi/mm3 (0.5 h) to about 0.0004 and 0.0006 μCi/mm3 (2 h), respectively. Meanwhile, the signal intensities of kidneys and bladder increase considerably from 0.0015 and 0.0008 (0.5 h) to 0.0072 and 0.0059 μCi/mm3 (1 h), respectively.
In vivo CT imaging
The CT results were similar to the obtained SPECT images. After the administration of Au-Ac-PENPs, the brighter zones can be easily detected in the lung, liver and kidneys (), while the brighter zones in heart, liver, kidneys and postcaval vein are also significant after the injection of Au-Gly-PENPs (). Likewise, to quantitatively evaluate the CT contrast enhancement, the average HU of ROIs was obtained and the relative HU (RHU) was also recorded as the HU ratio of pre- and post-injection images. As shown in , Au-Ac-PENPs display the main accumulation in the liver and spleen, but very little in the blood. Within 2 h postinjection of Au-Ac-PENPs, the RHUs of liver and spleen remain at about 2.2 and 1.8, respectively, while the RHU of lung slightly increases from 3.1 (0.5 h) to 3.6 (1 h), and then considerably reduces to 1.9 (2 h). In contrast, Au-Gly-PENPs show predominant accumulation in heart and postcaval vein at 0.5 h postinjection (). After that, the RHUs of postcaval vein and heart reduce significantly from 5.4 and 2.9 (0.5 h) to about 1.2 and 1.3 (2 h). While the RHUs of liver, spleen and lung are all below 1.5 within 2 h postinjection of Au-Gly-PENPs. At the same time, the CT contrast enhancement of Au-Gly-PENPs in kidneys and bladder can be detectable at 0.5 h postinjection and remains constant in hours.
Figure 5. Micro-CT images of a mouse lung, liver, and kidneys (a) before and at (b) 0.5 h, (c) 1 h, and (d) 2 h post intravenous injection of Au-Ac-PENPs. Micro-CT images of a mouse heart, liver, kidneys and postcaval vein (e) before and at (f) 0.5 h, (g) 1 h, and (h) 2 h post intravenous injection of Au-Gly-PENPs.
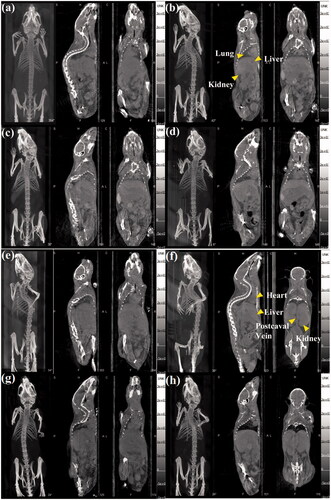
Figure 6. Relative CT value of different organs before and at different time points post intravenous injection of (a) Au-Ac-PENPs and (b) Au-Gly-PENPs. Biodistribution of 99mTc-Au-PENPs in the blood, muscle, and major organs of the mice at different time points post intravenous injection of (c) 99mTc-Au-Ac-PENPs and (d) 99mTc-Au-Gly-PENPs.
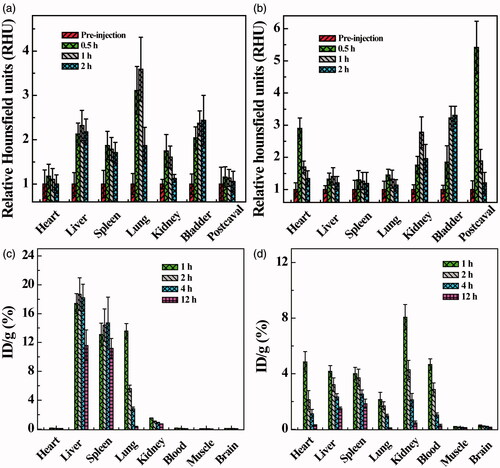
Biodistribution study
We next tested the biodistribution behaviour of the Au PENPs. As shown in , 99mTc-Au-Ac-PENPs mainly accumulate in the lung, liver and spleen at 1 h postinjection, while the highest uptake of 99mTc-Au-Gly-PENPs is in kidneys (3.0% ID/g), followed by heart, liver, spleen, blood and lung. The muscle and brain have the lowest uptake in the whole study period. 99mTc-Au-Ac-PENPs have significantly reduced uptake in lung (from 13.6% to 0.3% ID/g), but have slightly decreased uptake in liver (from 17.4% to 11.6% ID/g), kidneys (from 1.5% to 0.7% ID/g) and spleen (from 13.1% to 11.2% ID/g) within 12 h postinjection. On the contrary, 99mTc-Au-Gly-PENPs exhibit a different distribution pattern in vivo (). A sharp decrease of 99mTc-Au-Gly-PENPs in blood, kidneys and heart is observed from 4.7%, 8.1%, and 4.9% ID/g (1 h) to 0.3%, 0.5%, and 0.3% ID/g (12 h), respectively, while their uptake in liver and spleen gradually reduces from 4.2% and 4.0% ID/g to 1.5% and 1.9% ID/g, respectively.
In vivo sentinel lymph node imaging
The use of the Au PENPs for SLN imaging was then exploited. As shown in , the popliteal SLNs can be detected in both CT and SPECT imaging at 0.5 h post subcutaneous injection of 99mTc-Au-Ac-PENPs and 99mTc-Au-Gly-PENPs into the left and right footpads of a normal rabbit, respectively. Subsequently, the accumulation in SLNs gradually becomes higher and brighter with time. At 4 h post injection, both the left and right SLNs show the accurate delineation and clear boundary with surrounding tissues. Quantitatively, the HU values of both SLNs were measured at different time points. As shown in Figure S6 (Supporting Information), the HU value of right side increases steadily from 6.2 to 101, and that of the left side from 5.8 to 108.
Discussion
At present, various NPs are being investigated as SPECT and CT imaging agents, such as liposomes, micelles, dendrimers, Au and metal oxide NPs [Citation9,Citation29]. In this work, we have explored 99mTc-labelled Au PENPs for dual mode SPECT/CT imaging applications. First, PEI was modified with DTPA chelator for a final step of radiolabeling of 99mTc. Then the surface of PEI was PEGylated to improve the biocompatibility, weaken the uptake by the reticuloendothelial system (RES), and extend the blood circulation time of the particles. Via the reduction of Au(III) to Au(0) using NaBH4, the DTPA- and mPEG-conjugated PEI was used as a template to synthesize Au-PENPs for CT imaging. It has been reported that the metabolism and biodistribution of NPs in vivo were mainly determined by their physicochemical properties [Citation26,Citation40,Citation41]. Therefore, we modified the PEI remaining surface amines with acetyl and hydroxyl groups, respectively to gain Au-Ac-PENPs and Au-Gly-PENPs, which can be finally radiolabelled with 99mTc. It seems that different surface modifications make no difference in terms of the high radiochemical yields and radiostabilities.
Before further evaluation in vivo, the cytotoxicity of Au PENPs with different surface groups was studied in vitro. Clearly, both Au-Ac-PENPs and Au-Gly-PENPs exhibited negligible cytotoxicity in the given Au concentration range. For in vivo toxicology study, blood compatibility is a critical factor to consider for subsequent imaging evaluation. The haemolysis percentages of both nanomaterials at a concentration as high as 400 μg/ml were less than 4%, suggesting their excellent haemocompatibility. Moreover, H&E stained organ slices of both test groups did not show any perceptible lesions, hydropic damages and other adverse effects, and the morphology of these organ sections was as normal as that of the control group. Furthermore, behaviour observation, body weight measurements, and histological changes of the major organs of mice were carried out to confirm their relatively long-term toxicity. Obviously, our results revealed that the Au PENPs did not display apparent toxicological behaviours.
Since the formed nanomaterials could be significantly accumulated in liver and spleen and excreted through the urinary system, serum biochemistry analysis and haematological assessment were carefully performed to assess the potential adverse effects on hepatorenal and splenic functions. The normal level of BUN value and liver function markers (ALP, A/G, ALT and AST) in test groups indicated the good function of the liver and renal system. In terms of haematological assessment, all parameters obtained from the mice in test groups had the same trend with those of mice in the control group, proving the biosafety to the spleen. All these in vivo results indicated that Au PENPs exhibited excellent biocompatibility and biosafety, which is important for their further studies in vivo.
The feasibility to use 99mTc-labelled Au PENPs for SPECT/CT imaging was investigated in vivo. In the initial time frame, 99mTc-Au-Ac-PENPs were mainly accumulated in the lung, while 99mTc-Au-Gly-PENPs were in blood, heart, kidneys and postcaval vein. Then, the accumulation of both 99mTc-Au-Ac-PENPs and 99mTc-Au-Gly-PENPs in liver became higher with time, indicating the uptake by the RES, which is similar to the distribution of many other NPs developed in the literature [Citation26]. After that, the signal intensity of kidneys declined continually, suggesting the excretion through the urinary system, and 99mTc-Au-Gly-PENPs had a relatively faster clearance. The results suggest that different surface modifications endow the formed nanomaterials with distinct behaviours in vivo due to their different hydrodynamic sizes and surface functional groups. The CT results were similar to the obtained SPECT images, indicating the co-existence of Au PENPs and 99mTc with acceptable stability in vivo. Moreover, the biodistribution of 99mTc-Au-PENPs was well consistent with the gained SPECT and CT images. The lung, liver and spleen were the major organs with 99mTc-Au-Ac-PENPs accumulation, while 99mTc-Au-Gly-DENPs were taken up in the blood, heart and kidneys at 1 h postinjection. For long-term in vivo biodistribution, the largest proportion of Au PENPs was found in liver and spleen, and the content presented a sustained decline with time. Most of Au PENPs could be eliminated from the body after a week. Therefore, the designed Au PENPs have the potential to be an excellent dual mode contrast agent for SPECT/CT imaging of different organs.
SLN mapping is an important tool to evaluate the nodal status for clinical cancer patient treatment [Citation42]. However, current contrast agents for SLN imaging suffer from the deficiencies of rapid diffusion and accumulation into nonsentinel lymph nodes, making it difficult to distinguish selectively SLN from other subsequent nodes [Citation43]. Since NPs possess extended retention time at the SLN and low diffusion into nonsentinel lymph nodes, they can be excellent candidates for SLN imaging. Therefore, in this study, the possibility to utilize the formed 99mTc-Au-PENPs for dual modal SPECT/CT imaging of lymph-node was also tested. As expected, the accumulation in the popliteal lymph node could be clearly seen at 1 h postinjection and the SLN regions steadily turned brighter with time, indicating the efficient diffusion of both 99mTc-Au-Ac-PENPs and 99mTc-Au-Gly-PENPs to the SLN from the injection region. The 99mTc-Au-Gly-PENPs seemed to diffuse quicker than 99mTc-Au-Ac-PENPs, due to the relatively smaller hydrodynamic size. The excellent SLN dual-mode imaging performance made the designed contrast agent as potential candidates for lymph node SPECT/CT imaging as well.
Conclusion
In summary, we developed 99mTc-labelled multifunctional Au PENPs for dual-mode SPECT/CT imaging applications. Via the versatile PEI-enabled nanotechnology, Au NPs were loaded within the PEI interior and 99mTc was labelled on the PEI surface via chelating. The formed Au PENPs displayed excellent stability, biocompatibility, enhanced X-ray attenuation property and SPECT/CT imaging performance. Remarkably, the formed Au PENPs with surface acetyl or hydroxyl groups have different in vivo behaviours in dual mode SPECT/CT imaging and biodistribution studies, allowing for the preferential SPECT/CT imaging of different organs. Moreover, the developed Au PENPs show their potential for efficient SLN SPECT/CT imaging. Taken consideration into the potential of PEI for facile further functionalization with various targeting molecules and outstanding gene delivery ability, the developed nanoplatform in this study may be explored for targeted imaging and therapy of different types of cancer.
Lingzhou_et_al._Supplementary_Material.doc
Download MS Word (3.6 MB)Acknowledgements
L. Zhao thanks the support from the Shanghai Sailing Program (16YF1409300). M. Zhu thanks the support from the Ningxia Medical University special talent project (XT201405). X. Shi also acknowledges the support by FCT-Fundação para a Ciência e a Tecnologia (project PEst-OE/QUI/UI0674/2013, CQM, Portuguese Government funds), and through Madeira 14-20 Program, project PROEQUIPRAM – Reforço do Investimento em Equipamentos e Infraestruturas Científicas na RAM (M1420-01-0145-FEDER-000008) and by ARDITI-Agência Regional para o Desenvolvimento da Investigação Tecnologia e Inovação through the project M1420-01-0145-FEDER-000005-Centro de Química da Madeira-CQM + (Madeira 14-20).
Disclosure statement
Authors do not have any conflict of interest to declare.
Additional information
Funding
References
- Lee D, Koo H, Sun I, et al. Multifunctional nanoparticles for multimodal imaging and theragnosis. Chem Soc Rev. 2012;41:2656–2672.
- Li J, He Y, Sun W, et al. Hyaluronic acid-modified hydrothermally synthesized iron oxide nanoparticles for targeted tumor MR imaging. Biomaterials. 2014;35:3666–3677.
- Pysz MA, Gambhir SS, Willmann JK. Molecular imaging: current status and emerging strategies. Clin Radiol. 2010;65:500–516.
- Ametamey SM, Honer M, Schubiger PA. Molecular imaging with PET. Chem Rev. 2008;108:1501–1516.
- Dobrucki LW, Sinusas AJ. PET and SPECT in cardiovascular molecular imaging. Nat Rev Cardiol. 2010;7:38–47.
- Drzezga A, Souvatzoglou M, Eiber M, et al. First clinical experience with integrated whole-body PET/MR: comparison to PET/CT in patients with oncologic diagnoses. J Nucl Med. 2012;53:845–855.
- Mariani G, Bruselli L, Kuwert T, et al. A review on the clinical uses of SPECT/CT. Eur J Nucl Med Mol Imaging. 2010;37:1959–1985.
- von Schulthess GK, Steinert HC, Hany TF. Integrated PET/CT: current applications and future directions. Radiology. 2006;238:405–422.
- Qiao Z, Shi X. Dendrimer-based molecular imaging contrast agents. Prog Polym Sci. 2015;44:1–27.
- Xie J, Lee S, Chen X. Nanoparticle-based theranostic agents. Adv Drug Deliv Rev. 2010;62:1064–1079.
- Daraee H, Etemadi A, Kouhi M, et al. Application of liposomes in medicine and drug delivery. Artif Cells Nanomed Biotechnol. 2016;44:381–391.
- Zhu W, Wang D, Peng L, et al. An experimental study on the application of radionuclide imaging in repairing bone defects. Artif Cells Nanomed Biotechnol. 2013;41:304–308.
- Jahangiri S, Akbarzadeh A. Preparation and in vitro evaluation of methotrexate-loaded magnetic nanoparticles modified with biocompatible copolymers. Artif Cells Nanomed Biotechnol. 2016;44:1733–1740.
- Garg T. Current nanotechnological approaches for an effective delivery of bio-active drug molecules in the treatment of acne. Artif Cells Nanomed Biotechnol. 2016;44:98–105.
- Zeinali Sehrig F, Majidi S, Nikzamir N, et al. Magnetic nanoparticles as potential candidates for biomedical and biological applications. Artif Cells Nanomed Biotechnol. 2016;44:918–927.
- Chen Q, Li K, Wen S, et al. Targeted CT/MR dual mode imaging of tumors using multifunctional dendrimer-entrapped gold nanoparticles. Biomaterials. 2013;34:5200–5209.
- Liu H, Wang H, Xu Y, et al. Lactobionic acid-modified dendrimer-entrapped gold nanoparticles for targeted computed tomography imaging of human hepatocellular carcinoma. ACS Appl Mater Interfaces. 2014;6:6944–6953.
- Wen S, Li K, Cai H, et al. Multifunctional dendrimer-entrapped gold nanoparticles for dual mode CT/MR imaging applications. Biomaterials. 2013;34:1570–1580.
- Wen S, Zheng F, Shen M, et al. Surface modification and PEGylation of branched polyethyleneimine for improved biocompatibility. J Appl Polym Sci. 2013;128:3807–3813.
- Wen S, Zheng F, Shen M, et al. Synthesis of polyethyleneimine-stabilized gold nanoparticles for colorimetric sensing of heparin. Colloids Surf A Physicochem Eng Asp. 2013;419:80–86.
- Zhou B, Zheng L, Peng C, et al. Synthesis and characterization of PEGylated polyethylenimine-entrapped gold nanoparticles for blood pool and tumor CT imaging. ACS Appl Mater Interfaces. 2014;6:17190–17199.
- Zhou S, Wu Z, Chen X, et al. PEGylated polyethylenimine as enhanced T1 contrast agent for efficient magnetic resonance imaging. ACS Appl Mater Interfaces. 2014;6:11459–11469.
- Zhu J, Zheng L, Wen S, et al. Targeted cancer theranostics using alpha-tocopheryl succinate-conjugated multifunctional dendrimer-entrapped gold nanoparticles. Biomaterials. 2014;35:7635–7646.
- Luo Y, Zhao L, Li X, et al. The design of a multifunctional dendrimer-based nanoplatform for targeted dual mode SPECT/MR imaging of tumors. J Mater Chem B. 2016;4:7220–7225.
- Li X, Xiong Z, Xu X, et al. 99mTc-labeled multifunctional low-generation dendrimer-entrapped gold nanoparticles for targeted SPECT/CT dual-mode imaging of tumors. ACS Appl Mater Interfaces. 2016;8:19883–19891.
- Wen S, Zhao L, Zhao Q, et al. A promising dual mode SPECT/CT imaging platform based on 99mTc-labeled multifunctional dendrimer-entrapped gold nanoparticles. J Mater Chem B. 2017;5:3810–3815.
- Albernaz MS, Ospina CA, Rossi AM, et al. Radiolabelled nanohydroxyapatite with 99mTc: perspectives to nanoradiopharmaceuticals construction. Artif Cells Nanomed Biotechnol. 2014;42:88–91.
- Thorek DLJ, Ulmert D, Diop N-FM, et al. Non-invasive mapping of deep-tissue lymph nodes in live animals using a multimodal PET/MRI nanoparticle. Nat Commun. 2014;5:3097.
- Xing Y, Zhao J, Conti PS, et al. Radiolabeled nanoparticles for multimodality tumor imaging. Theranostics. 2014;4:290–306.
- Yan X, Song X, Wang Z. Construction of specific magnetic resonance imaging/optical dual-modality molecular probe used for imaging angiogenesis of gastric cancer. Artif Cells Nanomed Biotechnol. 2017;45:399–403.
- Yamano S, Dai J, Hanatani S, et al. Long-term efficient gene delivery using polyethylenimine with modified Tat peptide. Biomaterials. 2014;35:1705–1715.
- Lin C, Ge J. Multifunctional polyethylenimine-conjugated superparamagnetic nanoparticles for drug delivery and imaging. J Control Release. 2011;152:e58–e60.
- Cai H, An X, Cui J, et al. Facile hydrothermal synthesis and surface functionalization of polyethyleneimine-coated iron oxide nanoparticles for biomedical applications. ACS Appl Mater Interfaces. 2013;5:1722–1731.
- Li J, Zheng L, Cai H, et al. Polyethyleneimine-mediated synthesis of folic acid-targeted iron oxide nanoparticles for in vivo tumor MR imaging. Biomaterials. 2013;34:8382–8392.
- Chen KJ, Wolahan SM, Wang H, et al. A small MRI contrast agent library of gadolinium(III)-encapsulated supramolecular nanoparticles for improved relaxivity and sensitivity. Biomaterials. 2011;32:2160–2165.
- Li J, Hu Y, Yang J, et al. Hyaluronic acid-modified Fe3O4@Au core/shell nanostars for multimodal imaging and photothermal therapy of tumors. Biomaterials. 2015;38:10–21.
- Farvadi F, Tamaddon A, Sobhani Z, et al. Polyionic complex of single-walled carbon nanotubes and PEG-grafted-hyperbranched polyethyleneimine (PEG-PEI-SWNT) for an improved doxorubicin loading and delivery: development and in vitro characterization. Artif Cells Nanomed Biotechnol. 2017;45:855–863.
- Dube B, Pandey A, Joshi G, et al. Hydrophobically modified polyethylenimine-based ternary complexes for targeting brain tumor: stability, in vitro and in vivo studies. Artif Cells Nanomed Biotechnol. 2017;45:1685–1698.
- Wen S, Zhao Q, An X, et al. Multifunctional PEGylated multiwalled carbon nanotubes for enhanced blood pool and tumor MR imaging. Adv Healthc Mater. 2014;3:1568–1577.
- Albanese A, Tang PS, Chan WCW. The effect of nanoparticle size, shape, and surface chemistry on biological systems. Annu Rev Biomed Eng. 2012;14:1–16.
- He C, Hu Y, Yin L, et al. Effects of particle size and surface charge on cellular uptake and biodistribution of polymeric nanoparticles. Biomaterials. 2010;31:3657–3666.
- Swartz MA, Lund AW. Lymphatic and interstitial flow in the tumour microenvironment: linking mechanobiology with immunity. Nat Rev Cancer. 2012;12:210–219.
- Pons T, Pic E, Lequeux N, et al. Cadmium-free CuInS2/ZnS quantum dots for sentinel lymph node imaging with reduced toxicity. ACS Nano. 2010;4:2531–2538.