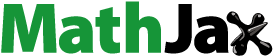
Abstract
Oxidative toxicity has impeded the development of haemoglobin-based oxygen carriers (HBOCs) by causing methaemoglobin (MetHb) formation and inducing oxidative stress. In our previous work, polydopamine-coated haemoglobin (Hb-PDA) nanoparticles have been designed and synthesized with the capacity to reduce oxidative toxicity. In this investigation, the mass ratio of dopamine (DA) to haemoglobin (Hb) and the pH value are found to be the primary factors that influence preparation of Hb-PDA nanoparticles. X-ray photoelectron spectroscopy showed that the catechol groups of DA play a crucial role in the modification of Hb surface. Hb-PDA nanoparticles were found to exhibit oxidative protection from hydrogen peroxide (H2O2) and the change of mitochondrial membrane potential showed that the Hb-PDA nanoparticles reduced H2O2-induced apoptosis. It is demonstrated that modification of PDA could maintain the oxygen-release capacity of Hb. These findings confirm that Hb-PDA nanoparticles possess restrained oxidative toxicity and preserve oxygen-release capacity.
Introduction
High worldwide civilian demands and military conflicts have inspired the development of haemoglobin-based oxygen carriers (HBOCs) over the past three decades [Citation1]. The potential advantages of HBOCs include universal compatibility without blood type matching, elimination of infection and long storage life [Citation2]. However, oxidative toxicity has impeded the development of HBOCs by causing methaemoglobin (MetHb) formation and inducing oxidative stress [Citation3–5]. In our previous work, polydopamine-coated haemoglobin (Hb-PDA) nanoparticles with antioxidant properties were synthesized to reduce Hb auto-oxidation and oxidative damage. Hb-PDA nanoparticles showed protection of Hb by reducing the generation of MetHb, as well as excellent antioxidant properties by scavenging free radicals. Meanwhile, it also indicated that Hb-PDA nanoparticles reduced the intracellular hydrogen peroxide (H2O2)-induced reactive oxygen species generation [Citation6].
Polydopamine (PDA), as a novel coating material, not only protects the underlying materials from external erosion but also confers the new functionalities to them, which have been attracted wide attention in the biological and biomedical sciences. Although the polymerization of dopamine (DA) is an efficient and simple process, formation of PDA is affected by factors including the starting concentration of DA monomer and characteristics of the reaction solution [Citation7–10]. At low concentrations of DA (0.5 mM), the cyclized indole (DHI) is produced. While at higher DA concentrations (10 mM), a greater proportion of uncycled (DA)2/DHI elements are generated because quinone is prone to being trapped by DA [Citation11]. Additionally, pH is critical to the polymerization process. The thickness of PDA film increases when the pH value changed from 5 to 8.5. It should be noted that it would levelled off with further increase in pH value [Citation12]. The detailed mechanism for PDA attachment is not fully understood. A variety of modes about interaction forms have been demonstrated, such as co-ordinate bonding, bidentate chelating bonding and bridged bidentate bonding [Citation7,Citation13–16]. Recently, an interaction scheme has been reported concerning DA binding to metal cations, which demonstrated one hydrogen group of catechol binds metal cations and another hydrogen group forms a hydrogen bridge to a neighbouring surface hydroxide [Citation17]. Establishing the mechanism of PDA-mediated Hb surface modification helps to provide an understanding of the oxygen-release capacity of Hb-PDA nanoparticles.
In vitro measurement of oxygen dissociation curves (ODC) has been used in a number of studies to reflect the oxygen affinity of HBOCs [Citation18–20]. The initial measurement procedure determines the oxygen chemically bound to the Hb after equilibrating the samples with air [Citation21]. Subsequently, the measurements use the optical properties of oxyhaemoglobin and deoxyhaemoglobin to calculate the value of oxygen saturation by adjusting the oxygen pressure [Citation22]. The Hemox Analyser has been universally used to record the ODC by means of an oxygen electrode and spectrophotometry [Citation23]. It should be noted that the optical parameters are related to the size, shape, orientation and refractive index of the samples [Citation24,Citation25]. PDA shows a broadband absorption spectrum, which has influence on measurement of ODC by the Hemox Analyser. Since simple and effective equipment are not available to overcome the influence of optical parameters, evaluation of oxygen-release capacity remains a challenge [Citation22,Citation26].
In this work, the effects of parameters on the fabrication of Hb-PDA nanoparticles were investigated, such as the mass ratio of DA/Hb, type of buffer and pH value. X-ray photoelectron spectroscopy (XPS) characterization focussed on confirming the chemical elements on the surface to uncover the interaction between PDA and Hb. The oxygen-release capacity of Hb-PDA nanoparticles as oxygen carriers was investigated using a novel system to monitor the change of oxygen partial pressure (PO2). Protection of Hb from H2O2-induced MetHb was determined based on absorption at different wavelengths. And the change of mitochondrial membrane potential was monitored and quantified to demonstrate H2O2-induced apoptosis.
Materials and methods
Materials
DA was purchased from Sigma-Aldrich (Santa Clara, CA). Phosphate-buffered saline (10X PBS, pH 7.2–7.4) was provided by Fisher Scientific (San Jose, CA). Tris-HCl buffer (10 mM, pH 8.5) was purchased from Beijing Leagene Biotech (China). Foetal bovine serum (FBS) was purchased from Hyclone (Logan, UT). Antibiotics were purchased from Excell Biology (China). The mitochondrial membrane potential assay kit, including 5, 5′, 6, 6′-tetrachloro-1, 1′, 1, 3′-tetraethylbenzimidazolo-carbocyanine iodide (JC-I), was provided by Beyotime Institute of Biotechnology (China). RPMI 1640 culture media were purchased from Gibco (Grand Island, NY). All chemicals were used without further purification.
Preparation of bovine Hb
Bovine Hb was prepared and purified from bovine red blood cells by hypotonic haemolysis [Citation26]. Fresh bovine red blood cells from packed bovine whole blood were washed three times with ice-cold PBS solution. Following haemolysis by stirring and centrifugation (10,000g, 1 h, 4 °C), the supernatant was purified via anion-exchange chromatography to obtain Hb solutions at a concentration of 5 g/dL, determined by a blood gas analyser (Radiometer ABL90COOX, Denmark). After filtration through a 0.22 µm polyether sulphone filter, Hb solutions were stored at −80 °C.
Preparation of Hb-PDA nanoparticles and BSA-PDA nanoparticles
To prepare the Hb-PDA nanoparticles and BSA-PDA nanoparticles, 48.8 mg of Hb or bovine serum albumin (BSA) was mixed with 9.76 mg DA in Tris-HCl buffer (10 mM, pH 8.5). Following oxidative polymerization with slight stirring for 210 min, the nanoparticles were then dialysed against PBS (pH 7.4) to remove excess DA. The Hb-PDA and BSA-PDA nanoparticles were used for the further measurements.
Influence of material mass ratio on size and zeta potential of nanoparticles
About 48.8 mg of Hb was mixed with DA at different DA/Hb mass ratios of 1:10, 1:5 and 1:2.5 in Tris-HCl buffer (10 mM, pH 8.5). Following oxidative polymerization with slight stirring for 210 min, the Hb-PDA nanoparticles were then dialysed against PBS (pH 7.4) to remove excess DA. The size distribution and zeta potential of nanoparticles were measured using a Zetasizer Nano series Nano-ZS (Malvern Instruments Ltd., 150 Malvern, UK). UV–vis spectra were recorded using a UV–Vis spectrophotometer Hitachi U2800 (Hitachi High Technologies, Japan) over a wavelength range of 350–250 nm.
Influence of different buffer and pH value on size of nanoparticles
To test influence of different buffer, 48.8 mg of Hb was incubated with 9.76 mg DA in deionized water, 0.1 M PBS and 0.9% NaCl (all pH 7.0). To test influence of pH value on size of nanoparticles, 48.8 mg of Hb was incubated with 9.76 mg DA in 0.1 M PBS with pH 6.0, 7.0 and 8.0. Following oxidative polymerization with slight stirring for 210 min, the Hb-PDA nanoparticles were then dialysed against PBS (pH 7.4) to remove excess DA. The size distribution of nanoparticles was measured.
Influence of pH on zeta potential of Hb-PDA nanoparticles
To examine the effect of pH on the zeta potential of Hb-PDA nanoparticles, zeta potential was measured after nanoparticles were suspended in solutions of consistent ionic strength (150 mM) with different pH (6, 6.5, 7, 7.5 and 8).
XPS analysis
The Hb-PDA nanoparticles were dialysed against deionized water for 6 h. The freeze-drying nanoparticles were obtained by a 6 h cold drying process. Changes of the surface elements before and after polymerization were determined by XPS. XPS analyses were carried out using the X-ray photoelectron spectrometer (Thermo Scientific, ESCALAB 250Xi, San Jose, CA) with an Al as the X-ray source. A vacuum pressure is better than 2 × 10−9 mBar. The chemical bonds were analysed via XPSPEAK 4.1.
Measurement of H2O2-induced MetHb
The effect on protection from H2O2-induced MetHb was determined based on absorption at 560, 576, 630 and 700 nm using a microplate reader PowerWave 340 (BioTek Instruments, Winooski, VT, USA) [Citation27]. About 200 µL of 1%, 1.5% and 2% (v/v) Hb or Hb-PDA nanoparticle suspension was diluted with deionized water, and then exposed to H2O2 solution with finally different concentrations (12.5, 25, 50 and 100 µM) at 37 °C for 30, 60 or 90 min. The oxyhaemoglobin (OxyHb), deoxyhaemoglobin (DeoxyHb) and MetHb concentrations were calculated using the following equations:
The changes of percentage of MetHb before and after the addition of H2O2 were calculated as follows:
MetHbbefore is the percentage of MetHb before the addition of H2O2. MetHbafter is the percentage of MetHb after the addition of H2O2. is used to represent the MetHb content of the samples.
H2O2-induced change of mitochondrial membrane potential
Human monocyte THP-1 were cultured in RPMI 1640 media with 10% FBS and 1% antibiotics in six-well plates at a density of ∼1 × 106 cells per well. Cells were cultured at 37 °C in 5% CO2 for 24 h. After 24 h, cells were incubated with Hb-PDA nanoparticles in a final concentration of 5 µM for 6 h. Then H2O2 was added to each well in a final concentration of 1 mM and cells were incubated for 24 h. Cells were then washed with JC-1 washing buffer three times, and suspended in 1 ml JC-1 working solution for 20 min at 37 °C. Each sample was washed three times with JC-1 washing buffer and then centrifuged at 600g for 5 min. The samples were observed using confocal laser microscopy with 488 and 525 nm excitation wavelengths (Leica DMI 4000B, Germany). Cell fluorescence intensity was measured using flow cytometry (CaliberBD FACS Caliber, San Jose, CA).
Evaluation of oxygen-release capacity
Hb, BSA-PDA and Hb-PDA samples were suspended in 0.9% NaCl solution and measured as Scheme 1. The PO2 in 0.9% NaCl solution was successively lowed to 0 mmHg after being mixed with nitrogen (N2). PO2 in samples solutions increased until it levelled off after saturation with air. Samples in oxygenation status were mixed with 0.9% NaCl solution free from oxygen. PO2 in the mixture was recorded by the oxygen electrode to confirm whether Hb-bound oxygen in samples could be released into the surrounding media. When a stable value was reached, the measurement was stopped. A temperature controller was used to maintain the reaction system at 37 ± 0.2 °C.
Scheme 1. Schematic representation of airproof apparatus with temperature controller designed to determine the oxygen-release capacity. Uniformly, all the pumps and switches were illustrated. T is the temperature controller. P is the oxygen electrode. M is the magnetic rotor. Samples are measured as follows: (1) 0.9% NaCl is pumped into chamber A. Connect A with the aeration device providing N2 until PO2 lowed to 0 mmHg. (2) Solution of sample is pumped into chamber B. Connect B with the aeration device providing air until PO2 levelled off. (3) Solution in B is pumped into A and mix them by magnetic rotor. (4) PO2 in the mixture was recorded by the oxygen electrode P. The temperature controller T of the instrument maintained the reaction system at 37 ± 0.2 °C.
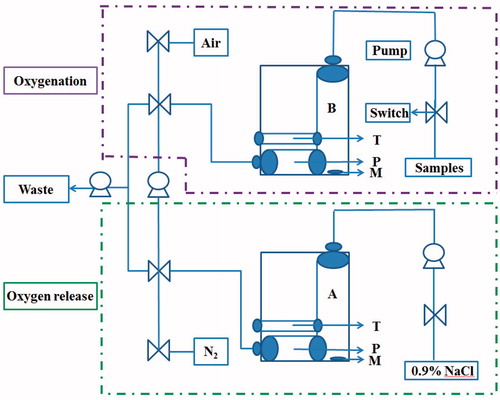
Statistical analysis
Data are expressed in terms of the mean ± standard deviation (SD) and analysed statistically with Mann Whitney test. Statistical differences were considered significant when p < .05.
Results
Influence of preparation conditions on Hb-PDA nanoparticles
The fabrication of Hb-PDA nanoparticles was achieved by the solution oxidation method. DA can be oxidized and self-polymerize in alkaline buffer solution. Despite the simplicity and effectiveness of the polymerization, several parameters affect the outcome such as the mass ratio of DA/Hb, type of the buffer and pH value [Citation28]. DA was added to Hb solution in Tris-HCl buffer solution (pH 8.5) at a DA/Hb mass ratio of 1:5 and left to react for 210 min. As shown in , Hb-PDA nanoparticles were obtained from the DA/Hb mass ratios of 1:10, 1:5 and 1:2.5, as Hb-PDA1, Hb-PDA2 and Hb-PDA3, respectively. The size, namely hydrodynamic diameter, was found to increase after successful PDA modification. In addition, the size changed from ∼7 to ∼10 nm when the mass ratio of starting DA/Hb increased, demonstrating that the thickness of the PDA film could be controlled by the starting mass ratio of DA/Hb, similar as the data of Jiang’s research [Citation29]. shows the UV–vis spectra of Hb-PDA nanoparticles, exhibiting a characteristic band around 280 nm, which demonstrates the generation of the PDA film [Citation6]. The absorbance varied significantly owing to the different degrees of self-polymerization depending on the starting mass of DA. In addition, the pH value is another crucial factor that influences the preparation of Hb-PDA nanoparticles. As depicted in , the DA monomer did not polymerize at pH 6.0. As the pH increased, the hydrodynamic diameter of the Hb-PDA nanoparticles gradually increased. To test if the type of buffer affect the formation of Hb-PDA nanoparticles, water, 0.1 M PBS buffer and 0.9% NaCl solution (all pH 7.0) were used at the same DA/Hb mass ratio 1:5 and no difference in the hydrodynamic diameters was observed as shown in .
Figure 1. Characterizations of synthesized Hb-PDA nanoparticles with different preparation parameters. (a) Particle sizes using different mass ratios of DA/Hb from 1/10 to 1/2.5. (b) UV–vis absorption spectrum of synthesized nanoparticles with different mass ratios of DA/Hb. (c) Particle sizes with different pH value in 0.1 M PBS buffer. (d) Particle sizes in different types of buffers with the same pH (7.0).
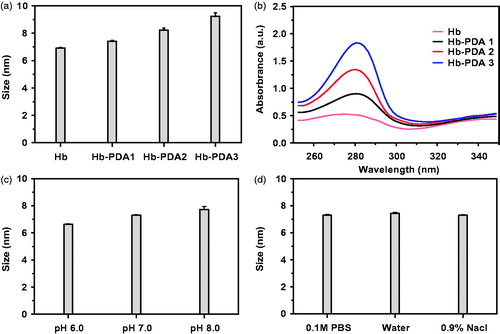
Influence of material mass and pH on zeta potential of nanoparticles
The zeta potential can be altered depending on starting mass ratios of DA/Hb. At low starting ratio such as 1/10 and 1/5, Hb-PDA nanoparticles have a negative surface charge. As shown in , increasing mass ratio results in the decrease in the negative zeta potential and became positive at the mass ratio of 1/2.5. To determine the effects of pH on the surface charge, Hb-PDA nanoparticles were dissolved in the solutions with the same ionic strength (150 mM) and different pH. Nanoparticles have a positive zeta potential when pH value is lower than 6.5, while the zeta potential is negative when pH is higher than 7 as shown in [Citation30].
Figure 2. Influence of mass ratio and pH value on zeta potential of Hb-PDA nanoparticles. (a) Zeta potentials of Hb-PDA nanoparticles in neutral aqueous solution with different mass ratios of DA/Hb from 1/10 to 1/2.5. (b) Zeta potentials of Hb-PDA nanoparticles in solutions with the same ionic strength (150 mM) and different pH (6–8).
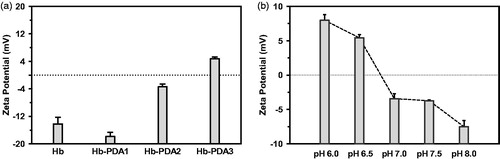
Interaction between PDA and haemoglobin
XPS characterizations were investigated to confirm the bonding state and chemical elements on the surface as shown in . The DA, Hb and Hb-PDA nanoparticles presented essentially similar type of elements on the surface with different elemental compositions in XPS survey spectrum in . Compared with that on Hb surface, elemental compositions ratio of N/O on Hb-PDA nanoparticles surface increased from 1.1 to 1.4 in ). Importantly, iron (Fe) is presented because heame was visualized in Hb. While it was absent on Hb-PDA nanoparticles as shown in ). This finding demonstrated PDA modified the Hb surface to shield the signals of Fe. The de-convoluted high-resolution XPS spectrum of C 1 s exhibited three energies at 284.5, 285.4 and 286.1 eV, associated with C–C/C = C, C–N, C–O from the protein chain, respectively [Citation31]. One peak of C 1 s at 287.6 eV was originated from C = O bond because of protein chains of Hb and the oxidation of catechol to quinones in the process of polymerization, which was absent on DA surface as shown in ). It was found that the O 1 s spectrum was composed of two peaks at 531.0 and 532.0 eV. These peaks could be assigned to C = O and C–O bond in agreement with the previous results C 1 s spectrum on the DA, Hb and Hb-PDA nanoparticles surface as shown in ) [Citation32]. The results from DA spectrum showed C–O bond without C=O bond observed. While the C=O bond, which indicates the polymerization of DA, was observed for the Hb-PDA surface. It can be tentatively concluded that DA self-polymerized on the Hb surface.
Figure 3. XPS analysis of DA, Hb and Hb-PDA nanoparticles. (a) General XPS survey of DA monomers, Hb and Hb-PDA nanoparticles. (b) Atomic composition of the C. (c) Atomic composition of the N and O. (d) N/O composition ratio. (e) De-convoluted high-resolution XPS spectrum of Fe for DA monomers, Hb and Hb-PDA nanoparticles. (f) Atomic composition of Fe for DA monomers, Hb and Hb-PDA nanoparticles.
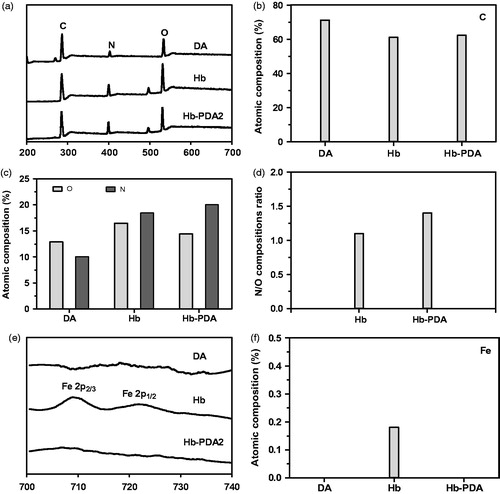
Protection of Hb from H2O2
To test capacity of protection from H2O2 in vitro, H2O2-induced MetHb production was determined by absorbance measurements. The Hb samples rapidly converted to MetHb with the addition of 100 mM H2O2. As the concentration of Hb increased, the MetHb content decreased. After reacting for 30, 60 and 90 min, the MetHb content in all Hb samples with different concentrations increased, as shown in . The MetHb content after the addition of Hb-PDA nanoparticles reduced as shown in . The MetHb content of the Hb samples and Hb-PDA samples increased depending on the H2O2 concentration after incubation for 30 min as shown in ). The MetHb content of Hb-PDA nanoparticles was lower than that of Hb at the same reaction time after the addition of 100 mM H2O2 in (p < .05). Thirty minutes after the addition of H2O2, the MetHb content of Hb-PDA nanoparticles was also lower than that of Hb at the same concentration of H2O2 in (p < .05).
Figure 5. H2O2-induced changes in MetHb contents. The MetHb contents in the (a) Hb and (b) Hb-PDA solutions with different concentration before and after the treatment with 100 mM H2O2 for 30–90 min. The MetHb contents in the (c) Hb and (d) Hb-PDA solutions with different concentration after the incubation for 30 min with different concentration of H2O2. (e) The MetHb contents in 1.5% Hb and Hb-PDA solutions with different time after the addition of 100 mM H2O2. (f) The MetHb contents in 1.5% Hb and Hb-PDA solutions after the addition of H2O2 with different concentration for 30 min. The data are presented as the mean ± SD and *p < .05.
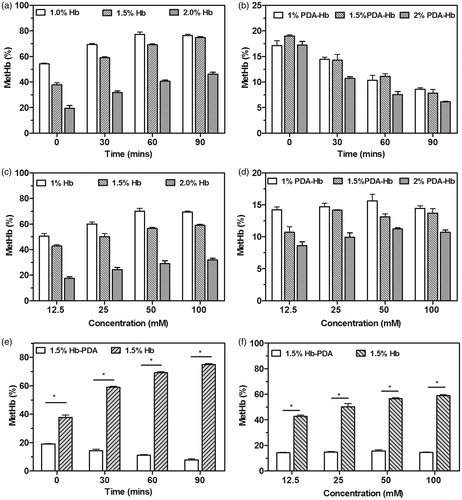
In pathological conditions, the over-generation of H2O2, an essential oxygen metabolite and source of oxidative stress, is known to induce apoptosis through changes of mitochondrial membrane potential. As shown in , red fluorescence represents the J-aggregates, while green fluorescence represents JC-1 monomer as H2O2-induced apoptosis leads to the low potential of the mitochondrial membrane. The two peaks at excitation wavelength 488 and 525 nm correspond to the fluorescence of the monomer and the J-aggregate, respectively. As shown in , the fluorescence intensity of cells treated with Hb-PDA nanoparticles was weaker than that of the H2O2 group, which indicates the suppressive effect of Hb-PDA on the generation of J-monomers.
Figure 6. H2O2-induced change of mitochondrial membrane potential. (a) Mitochondrial membrane potential of non-damaged cells stained with JC-1. The change of mitochondrial membrane potential evaluated by the fluorescence intensity of JC-1 aggregate or JC-1 monomer in the H2O2-induced damaged THP-1 cells after (b) untreated and (c) treated with Hb-PDA nanoparticles. Intensity of fluorescent was conducted by flow cytometry and observed by confocal microscopy. Red fluorescence represents the J-aggregates.
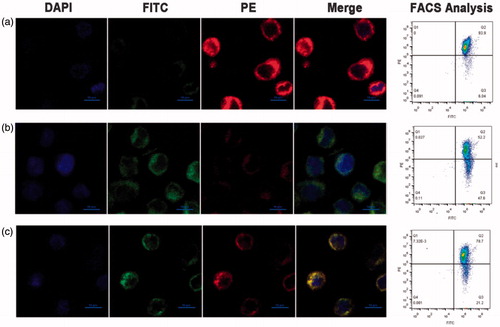
Oxygen-release capacity
BSA-PDA, Hb and Hb-PDA nanoparticles were dispersed in 0.9% NaCl solution. As shown in Scheme 1, 2 ml of 0.9% NaCl was introduced into the chamber A and filled with N2 until the PO2 lowed to 0 mmHg. The samples were then drawn into chamber B to be saturated with air. After mixing A and B, PO2 of the mixture was recorded. PO2 after the addition of BSA-PDA, Hb and Hb-PDA nanoparticles was 48.6 ± 1.5 mmHg, 55.6 ± 1.5 mmHg and 65.6 ± 2.5 mmHg, respectively, as shown in . BSA-PDA nanoparticles, used as a negative control, showed an increase in PO2 due to the oxygen dissolved in solution. The oxygen-release capacity of Hb-PDA nanoparticles was higher than that of Hb with the same Hb concentration, maybe due to the oxidative protection by PDA. The increase in PO2 after the addition of Hb-PDA1, Hb-PDA2 and Hb-PDA3 nanoparticles was 73.6 ± 2.08 mmHg, 72.0 ± 2.64 mmHg and 71.3 ± 2.08 mmHg, respectively, as shown in . The change in PO2 showed no difference, which indicates that mass ratio of DA/Hb has no effect on oxygen-release capacity.
Discussion
To study the effect of preparation conditions on Hb-PDA nanoparticles, the physicochemical properties of the particles were characterized. The polymerization of DA is immediate under mild conditions without requiring a complicated protocol and accompanied by a colour change from red to deep brown over time [Citation6]. It should be noted that elevation of the mass ratios of DA/Hb can accelerate the deposition of PDA on the surface, leading to greater film thickness. However, this can also result in aggregation of the nanoparticles [Citation33]. Therefore, the mass ratio of DA/Hb should be adjusted appropriately. Increasing pH value was found to have a significant effect on the polymerization process, owing to the neutralization of protons produced by oxidation, which facilitates the generation of PDA [Citation34]. The optimum conditions for Hb-PDA nanoparticle preparation were found to be pH 8.5 in 10 mM Tris-HCl buffer, with a DA/Hb mass ratio of 1:5.
Understanding the deposition mechanism of PDA is important. Although there is general acceptance that catechol is the functional group that facilitates the attachment of PDA to the surface of the substrate, the exact mechanism, particularly a protein as the substrate, has so far not been well established [Citation12]. Our present findings show that catechol groups may play an important role during the polymerization, based on the change of elements on the surface. This finding was consistent with researches which supports PDA was shown to be prone to diffusion onto substrates through non-covalent binding interactions such as metal coordination or chelating, and hydrogen bonding via catechol hydroxyl groups in PDA [Citation7,Citation35]. It has been demonstrated that the zeta potential of Hb-PDA nanoparticles becomes less negative with increasing degree of polymerization because the hydroxyl groups of DA are consumed in the reaction with Hb. Thus, the catechol groups of DA play an important role in attachment to the Hb surface.
Prevention of Hb autoxidation is significant to maintain the oxygen delivery capacity of HBOCs. Several strategies about the protection of Hb against autoxidation during HBOCs preparation have been reported recent years, such as Hemotech, platinum nanoparticle-embedded Hb-albumin clusters and haptoglobin-modified Hb [Citation36–38]. However, the preparation procedures of above approaches can be complicated because of chemical cross-linking and the addition of organic reagents. PDA has been recognized as an efficient antioxidant appropriate for biological systems owing to the presence of the distinct hydroquinone moiety without the need for instrumentation or harsh reaction conditions. A novel system for monitoring PO2 was used to show that Hb-PDA nanoparticles reduce MetHb production depending on antioxidant activity of PDA. This illustrates protection of Hb from oxidation, allowing it to maintain oxygen-release capacity. In addition to reduction of MetHb, the results reflect that Hb-PDA nanoparticles exhibited oxidative protection from H2O2. Hb-PDA nanoparticles contributed to relieving H2O2-induced oxidative damage and apoptosis, as indicated from change of mitochondrial membrane potential. Further research is needed to evaluate the anti-oxidation of Hb-PDA nanoparticles in detail. PDA coated on the Hb surface might help to stabilize the Hb tetramer, which has been suggested to reduce the oxidation rate of Hb. Hu et al. reported that oxidation of HbA was less likely when tetramer stability was improved by propylbenzmethylation at Val-1(a) of the PEGylated haemoglobin [Citation39]. The enhancement of Hb oxygen-release capacity as a result of oxidative protection could be beneficial for improving outcomes in ischaemia treatment.
In summary, the primary factors that influence the polymerization process are mass ratio of DA/Hb and the pH value. The catechol groups play a crucial role in Hb surface modification with PDA. It was shown that Hb-PDA nanoparticles exhibit oxidative protection from H2O2. The Hb-PDA nanoparticles exhibited an ideal oxygen-release capacity. These important characteristics have further confirmed that Hb-PDA nanoparticles have potential for application as oxygen carriers.
Disclosure statement
No potential conflict of interest was reported by the authors.
Additional information
Funding
References
- Alayash AI. Oxygen therapeutics: can we tame haemoglobin? Nat Rev Drug Discov. 2004;3:152–159.
- Moderypawlowski CL, Tian LL, Pan V, et al. Synthetic approaches to RBC mimicry and oxygen carrier systems. Biomacromolecules. 2013;14:939–948.
- Zhang J, Wang Y, You GX, et al. Conjugation with 20 kDa dextran decreases the autoxidation rate of bovine hemoglobin. Artif Cells Nanomed Biotechnol. 2017;08:1–8. DOI:10.1080/21691401.2017.1371184
- Amberson WR, Jennings JJ, Rhode CM. Clinical experience with hemoglobin-saline solutions. J Appl Physiol. 1949;1:469–489.
- Bian Y, Chang TM. A novel nanobiotherapeutic poly-[hemoglobin-superoxide dismutase-catalase-carbonic anhydrase] with no cardiac toxicity for the resuscitation of a rat model with 90 minutes of sustained severe hemorrhagic shock with loss of 2/3 blood volume. Artif Cells Nanomed Biotechnol. 2015;43:1–9.
- Wang Q, Zhang R, Lu M, et al. Bio-inspired polydopamine coated hemoglobin as potential oxygen carriers with antioxidant properties. Biomacromolecules. 2017;18:1333–1341.
- Ye Q, Zhou F, Liu W. Bioinspired catecholic chemistry for surface modification. Chem Soc Rev. 2011;40:4244–4258.
- Zhao Y, Zheng Y, Zhao C, et al. Hollow PDA-Au nanoparticles-enabled signal amplification for sensitive nonenzymatic colorimetric immunodetection of carbohydrate antigen 125. Biosens Bioelectron. 2015;71:200–206.
- Shao L, Zhang R, Lu J, et al. Mesoporous silica coated polydopamine functionalized reduced graphene oxide for synergistic targeted chemo-photothermal therapy. ACS Appl Mater Interfaces. 2016;9:1226–1236.
- Dreyer DR, Miller DJ, Freeman BD, et al. Elucidating the structure of poly(dopamine). Langmuir. 2012;28:6428–6435.
- Vecchia NFD, Avolio R, Alfè M, et al. Building‐block diversity in polydopamine underpins a multifunctional eumelanin‐type platform tunable through a quinone control point [J]. Adv Funct Mater. 2013;23:1331–1340.
- Liu Y, Ai K, Lu L. Polydopamine and its derivative materials: synthesis and promising applications in energy, environmental, and biomedical fields. Chem Rev. 2014;114:5057–5115.
- Chen LX, Liu T, Thurnauer MC, et al. Fe2O3 nanoparticle structures investigated by X-ray absorption near-edge structure, surface modifications, and model calculations. J Phys Chem B. 2002;106:8539–8546.
- Frye CL. Pentacoordinate silicon derivatives. II. 1 salts of bis(o-arylenedioxy) organosiliconic acids. J Am Chem Soc. 1964;86:3170–3171.
- Moser J, Punchihewa S, Infelta PP, et al. Surface complexation of colloidal semiconductors strongly enhances interfacial electron-transfer rates. Langmuir. 1991;7:3012–3018.
- Dalsin JL, Lin L, Tosatti S, et al. Protein resistance of titanium oxide surfaces modified by biologically inspired mPEG-DOPA. Langmuir. 2005;21:640–646.
- Rodenstein M, Zu¨rcher S, Tosatti SG, et al. Fabricating chemical gradients on oxide surfaces by means of fluorinated, catechol-based, self-assembled monolayers. Langmuir. 2010;26:16211–16220.
- Alayash AI. Hemoglobin-based blood substitutes: oxygen carriers, pressor agents, or oxidants? Nat Biotechnol. 1999;17:545–549.
- Alayash AI, Fratantoni JC, Bonaventura C, et al. Nitric oxide binding to human ferrihemoglobins cross-linked between either α or β subunits. Arch Biochem Biophys. 1993;303:332–338.
- Eich RF, Li T, Lemon DD, et al. Mechanism of NO-induced oxidation of myoglobin and hemoglobin. Biochemistry. 1996;35:6976–6983.
- Nelson MG, Savage GA, Cooke PJ, et al. Determination of the oxygen dissociation curve and P50 of whole blood. An evaluation of the Hem-O-Scan and instrumentation of laboratory systems. Am J Clin Pathol. 1981;75:395–399.
- Zhang X, Liu C, Yuan Y, et al. A noninvasive method for measuring the oxygen binding-releasing capacity of hemoglobin-loaded polymeric nanoparticles as oxygen carrier. J Mater Sci: Mater Med. 2009;20:1025–1030.
- Guarnone R, Centenara E, Barosi G. Performance characteristics of Hemox-Analyzer for assessment of the hemoglobin dissociation curve. Haematologica. 1995;80:426–430.
- Borovoi A, Naats E, Oppel U. Scattering of light by a red blood cell. J Biomed Opt. 1998;3:364–372.
- Rastar A, Yazdanshenas ME, Rashidi A, et al. Theoretical review of optical properties of nanoparticles. J Eng Fibers Fabr. 2013;8:85–96.
- Haney CR, Buehler PW, Gulati A. Purification and chemical modifications of hemoglobin in developing hemoglobin based oxygen carriers. Adv Drug Delivery Rev. 2000;40:153–169.
- Benesch RE, Benesch R, Yung S. Equations for the spectrophotometric analysis of hemoglobin mixtures. Anal Biochem. 1973;55:245–248.
- Bernsmann F, Ball V, Addiego F, et al. Dopamine-melanin film deposition depends on the used oxidant and buffer solution. Langmuir. 2011;2:2819–2825.
- Jiang J, Oberdörster G, Biswas P. Characterization of size, surface charge, and agglomeration state of nanoparticle dispersions for toxicological studies. J Nanopart Res. 2008;11:77–89.
- Wang Z, Dong Y, Li H, et al. Enhancing lithium-sulphur battery performance by strongly binding the discharge products on amino-functionalized reduced graphene oxide. Nat Commun. 2014;5:5002–5009.
- Zhang L, Shi J, Jiang Z, et al. Facile preparation of robust microcapsules by manipulating metal-coordination interaction between biomineral layer and bioadhesive layer. ACS Appl Mater Interfaces. 2011;3:597–605.
- Zhang R, Su S, Hu K, et al. Smart micelle@polydopamine core-shell nanoparticles for highly effective chemo-photothermal combination therapy. Nanoscale. 2015;7:19722–19731.
- Klosterman L, Bettinger CJ. Calcium-mediated control of polydopamine film oxidation and iron chelation. Int J Mol Sci. 2016;18:1–14.
- Lee H, Scherer NF, Messersmith PB. Single-molecule mechanics of mussel adhesion. Proc Natl Acad Sci USA. 2006;103:12999–13003.
- Dong Z, Gong H, Gao M, et al. Polydopamine nanoparticles as a versatile molecular loading platform to enable imaging-guided cancer combination therapy. Theranostics. 2016;6:1031–1042.
- Schaer CA, Deuel JW, Bittermann AG, et al. Mechanisms of haptoglobin protection against hemoglobin peroxidation triggered endothelial damage. Cell Death Differ. 2013;20:1569–1579.
- Tomita D, Kimura T, Hosaka H, et al. Covalent core-shell architecture of hemoglobin and human serum albumin as an artificial O2 carrier. Biomacromolecules. 2013;14:1816–1825.
- Simoni J, Simoni G, Wesson DE, et al. ATP-adenosine-glutathione cross-linked hemoglobin as clinically useful oxygen carrier. Curr Drug Discov Technol. 2012;9:173–187.
- Hu T, Li D, Wang J, et al. Propylbenzmethylation at val-1 (α) markedly increases the tetramer stability of the PEGylated hemoglobin: a comparison with propylation at Val-1 (α). Biochim Biophys Acta Biomembr. 2012;1820:2044–2051.