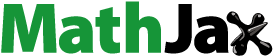
Abstract
Second generation E. coli DH5α (pKAU17) was successfully encapsulated by means of atomization (MA), inkjet printing (MI) and double-encapsulation (DDMI) for the purpose of urea degradation in a simulated uremic medium at 37 °C. Experimentally determined values of the effectiveness factor are 0.83, 0.28 and 0.34 for the MI, MA and DDMI capsules, respectively, suggesting that the catalytic activity of the E. coli DH5α (pKAU17) immobilized in MI capsule (d = 52 μm ± 2.7 μm) is significantly less diffusion-limited than in the case of the MA (d = 1558 μm ± 125 μm) and DDMI (d = 1370 μm ± 60 μm) bio-encapsulation schemes at the 98.3% CI. The proposed novel double encapsulation biofabrication method for alginate-based microspheres, characterized by lower membrane degradation rates due to secondary containment is recommended compared to the standard atomization scheme currently adopted across immobilization-based therapeutic scenarios. A Fickian-based mechanism is proposed with simulations mimicking urea degradation for a single capsule for the atomization and the inkjet schemes.
Introduction
For the past 50 years, encapsulation of cells and enzymes has been a promising approach for the treatment of metabolic deficiencies and cancer [Citation1–3]. Recent clinical trials have been providing increasing data supporting the benefits for the use of encapsulated biological materials to treat metabolic deficiencies and other acquired diseases [Citation3–5]. In some cases, the use of a single enzyme may be sufficient to provide a therapeutic effect; however, in other cases, the replacement of metabolic function may be better provided by a microorganism [Citation6]. Non-pathogenic bacterial cells can be genetically engineered to overproduce enzymes or to metabolize and degrade large amounts of toxic metabolites. For example, encapsulated Escherichia coli strain DH5 expressing Klebsiella aerogenes urease (E. coli DH5α (pKAU17)) can be used to metabolize small urea molecules that diffuse into microcapsules [Citation7,Citation8]. Genetically engineered bacteria are readily available since they are easy to grow quickly in large amounts and less expensive than enzymes [Citation8].
Bioencapsulation of cells membranes has been widely conducted using atomization [Citation9] producing capsule diameters between 200–2000 µm. With the advances in stem cell technology, molecular cloning, and tissue engineering, the demand for reliable high throughput production of smaller (<100 microns) structures is increasing [Citation10–15]. In order to meet this demand, biofabrication of miniaturized structures (<100 µm) by inkjet printing [Citation16], microfluidic technology including nano-imprinting [Citation17–19], and electro-spraying [Citation20,Citation21] characterized by high cell viability have been recently reported.
Regardless of biofabrication technique, the most common membrane material used in transplantation and cell therapy is alginate. Alginate is a linear diblock polysaccharide. The monomers can appear in homopolymeric blocks of consecutive G-residues (G-blocks) or consecutive M-residues (M-blocks), alternating M- and G-residues (MG-blocks), or randomly organized blocks. While the M-block segments develop into linear and flexible structures, the G-block residues give rise to folded and rigid structures that are responsible for a pronounced stiffness in the molecular chains [Citation22]. No alginate degrading enzymes exist in humans [Citation23]. Alginate is hydrophilic because of the presence of –OH and –COOH groups in its chain. At neutral pH, water penetrates into the chains of alginate to form hydrogen bridges through the –OH and COO− groups, and fills up the space along the chains and/or the centre of wide pores or voids [Citation24]. As a consequence, the cross-linked alginate tends to swell substantially. Additional swelling and destabilization are promoted by the presence of non-gelling ions and chelators, such as sodium, magnesium, phosphate, lactate and citrate present in physiological fluids and resulting in the dissociation of the cross-linked matrix [Citation23]. In order to further improve mechanical stability, alginates have been subjected to double-encapsulation [Citation25], photopolymerization [Citation26] and thermopolymerization [Citation27] to produce covalently crosslinked gels characterized by low cytotoxicity microenvironments [Citation28,Citation29]. Cross-linking by genipin also reduces swelling and physical disintegration of microcapsules induced by non-gelling ions and calcium sequestrants [Citation30]. The biocompatibility aspect of depolymerization-induced cytokine reactions has been addressed by the optimization of the G/M ratio content followed additional purification steps [Citation31], while the use a higher initial alginate concentrations can compensate for the viscosity loss due to depolymerization after sterilization [Citation32]. More recently the conjugation of triazole-thiomorpholine dioxide to alginate has enabled encapsulated islet implantation into the intraperitoneal space of nonhuman primates for at least six months without scar tissue building up [Citation33].
As an expected outcome of alginate capsule miniaturization by inkjet printing, the ratios of the outer layer diffusivities have been 4.25 and 5.07, respectively, for 4 and 70 kDa FITC markers, indicative of the enhanced diffusive potential of the miniaturized alginate-based capsules [Citation34]. In the same study, an analytical model was derived based on Fick’s second law for calculating the effective membrane diffusivity (De). The diffusion-adjusted kinetic rate equations for immobilized enzyme systems in macroporous membranes are given by EquationEquation (1)(1)
(1) [Citation35,Citation36] where rp is the rate of catalysis on the surface of the capsule and η is the effectiveness factor, Vmax and Km are Michaelis–Menten constants, S is the substrate concentration. Neglecting inhibition and competition, when S ≫ Km then the Michaelis–Menten kinetics can be approximated by a first-order decay characterized by the kinetic constant K.
(1)
(1)
In this study, the potential of E. coli DH5α (pKAU17) to degrade urea in simulated uremic media is reported. The objectives of this study are threefold: (a) to measure the effect of miniaturization on urea degradation; (b) to compare membrane integrity under simulated physiological conditions for different biofabrication schemes consisting of atomization, inkjet printing and double encapsulation of inkjet-printed capsules; and (c) to establish a theoretical framework for capsule miniaturization by mathematical simulation. Pairwise comparisons between the metabolic potential of free and immobilized bacteria were also conducted. The hydrogel biofabrication schemes developed in this paper may contribute to the development of “bottom–up” tissue engineering approaches and other therapeutic scenarios where transport within alginate membranes is limited by hydrogel mechanical strength and diffusion rate.
Materials and methods
Materials
All chemicals used to make the membranes were purchased from Sigma-Aldrich (Milwaukee, OH): low-molecular-weight sodium-alginate (LV) (A0682, 12–80 kDa), medium molecular weight alginate (A2033, µ > 2000 cP) and low-molecular-weight chitosan (44,886–9, 75% deacetylated, 3.8–6.0 kDa) . The piezo jetting device (MJ-B10–49-02) was purchased from Microfab Technologies (Texas, USA). Unless specified otherwise, all reagent grade salts, solvents, were procured from Fisher Scientific (Pittsburgh, PA).
Methods
Cell preparation
Urease enzyme expression in E. coli
Urease enzyme was produced by E. coli DH5α (pKAU17) [Citation37]. The pKAU17 plasmid contains the three genes for synthesis of the urease enzyme as well as four accessory proteins involved in nickel-dependent activation of the enzyme [Citation38,Citation39].
Induction
E. coli DH5α (pKAU17) were cultured in autoclaved 30 mL glass vials with 20 mL of autoclaved Luria-Bertani (LB) (Sigma Aldrich) medium containing autoclaved 1 mM nickel chloride and the pH was adjusted to 7.5 with 1.0 N. The optical density value (OD 600 nm) was monitored and 5 µL samples were collected and transferred hourly to a Cell-Vu hemocytometer (Fisher Scientific, USA) for cell count determination according to a standard growth monitoring procedure [Citation40]. When the medium reached an absorbance of 0.2–0.4 (∼6 h), isopropyl β-D-1-thiogalactopyranoside (IPTG) was added to a final concentration of 0.2 mM to induce expression of the urease gene. The culture was incubated overnight (additional ∼16 h) in an incubator shaker at 37 °C and 100 rpm and 1 mL of log phase cells (∼3.0 × 108 cells/mL) were harvested by centrifugation at ∼4000 rpm and 4 °C for 10 min. The LB supernatant was discarded and the pellet washed twice and resuspended in 100 µL sterilized 20 mM Na2HPO4, 1 mM EDTA, 2 mM 2-mercaptoethanol (i.e. protein extraction buffer – PEB – at pH 7.4) from Sigma-Aldrich Co. to remove all media components. No antibiotic was used throughout the study to select for the pKAU17 ampicillin-resistant plasmid.
Second generation E. coli DH5α growth curve
The pellet containing the induced bacteria was in turn cultured overnight (37 °C and 100 rpm) in sterilized 30 mL glass vials containing Luria-Bertani (LB) and 0.200 mL of 0.1 M nickel chloride solution. Growth was modelled during the exponential growth phase using Monod kinetics given by EquationEquation (2)(2)
(2)
(2)
(2)
where N is the number of bacteria in 1 mL culture solution, No is the initial cell count, kp is the growth constant. Based on the cell growth curve generated, bacteria cultures were used between 12-h and 19-h fermentation period to ensure bacteria growth in the log-phase region. Bacteria cells from this second generation were then used for urea degradation studies using different cell immobilization methodologies.
Microcapsule fabrication and size measurement
Shown in is a summarized diagram of the biofabrication methods explored in this research. An initial concentration of 3 × 108 cells/mL of bacteria (Ni) was used for the atomization and inkjet bio-printing schemes.
Atomization
Macrocapsules (MA) were fabricated using atomization according to previously established methodology [Citation41]. A 3.0% medium viscosity sodium-alginate autoclaved solution (µ = 500 cP, γ= 45 dyn/cm) mixed with bacteria (1 mL centrifuged bacteria/mL of alginate) was jetted into a 1.5% (w/v) CaCl2 bath for a cross-linking time period of one hour. The air (FA) and liquid (FL) flowrates were adjusted to 1.5 mL/min and 0.5 mL/min, respectively. The atomizer needle assembly is a concentric 24 G needle surrounded by a 16 G needle, through which the sodium alginate and air flows. The calcified sodium-alginate beads were then washed with 0.9% (w/v) NaCl twice.
Inkjet bio-printing
Microcapsules (MI) were fabricated using the Microfab’s Jetlab System based on the modification of a previously established method [Citation42]. The apparatus consisted of a CCD camera (30 fps), a control unit, a printhead, a triggering unit, a fluid delivery unit, and a PC equipped with proprietary software (MicroFab JetServer) to tune the bio-ink formulation to the jetting parameters. The printhead had an aperture of 60 µm and was rated for µ= 40 cP and γ= 72 dyn/cm. After inputting the jetting variable settings summarized in , droplet generation began with the triggering box sending electrical signals to the inkjet control unit and to the CCD camera control PC, simultaneously. The inkjet engine fired the 0.5% (w/v) filter-sterilized low viscosity sodium alginate solution [µ = 5 cP, γ = 43 dyn/cm] mixed with cells (1 mL centrifuged cells/mL of alginate), into a 15% (w/v) CaCl2 receiving solution. The beads were allowed to cross-link for 30 min. Following the cross-linking step, 1% (w/v) low viscosity chitosan was added into the stirred receiving solution to make the final chitosan concentration 0.5% (w/v). This physical adsorption step carried out for 30 min at room temperature was necessary to confer mechanical strength to the suspension during the subsequent concentration and centrifugation steps. The capsules were then centrifuged at 8,000 rpm for 5 min and washed with a 0.9% (w/v) NaCl solution three times.
Table 1. Bio-printer jetting parameters.
Double encapsulation
A concentrated pellet of inkjet-printed microcapsules was re-suspended into 1 mL of 3.0% medium viscosity sodium-alginate autoclaved solution and left to mix for one hour.
Droplet generation and cross-linking were carried out according to the procedure described in the atomization section. The double-membrane capsules (DDMI) were washed thrice with a 0.9% (w/v) NaCl solution.
Size measurement
Microcapsule sizes and membrane thicknesses were measured using a Nikon transmission microscope/camera equipped with an Interline CCD camera and imaging software NIS-Elements v.3.2.2.
Reaction medium preparation
A test solution consisting of 1 g/L of urea comparable to 1.2 g/L (200 mEq/L) detected in blood of patients with uremia was prepared [Citation43]. The base media consisted of 1.00 g/L glucose, 1.40 g/L Na2HPO4, 0.3 g/L KH2PO4, 0.07 g/L thiamin hydrochloride, 0.02 g/L MgSO4, dissolved in 1 L DI water. The test medium, in addition to the above constituents, also included 10% (w/v) LB. The reaction media was filter-sterilized using 0.22-µm Millex filters and the initial pH was measured to be 7.4.
Encapsulation efficiency
Encapsulation efficiency (E) given by EquationEquation (3)(3)
(3) is the ratio of the encapsulated (Nf) over the initial (Ni) bacterial count.
(3)
(3)
To measure the efficiency of encapsulation of the MA (EMA) and MI (EMI) capsules, the membranes were suspended in a sodium citrate solution for 10 min followed by cell count. The sodium citrate concentration used was 1.3 mg/mL and 50 mg/mL for the MA and MI, respectively. For the double encapsulation procedure, the encapsulation efficiency was assumed to be a product of the encapsulation yield from each step (EMI × EMA).
Urea concentration measurements
Urea absorbance was measured using a colorimetric assay (QuantiChrom Urea Assay Kit (DIUR-500), BioAssay Systems, (Hayward, CA)) at 520 nm using an Agilent 8453 UV-Vis spectrometer. The upper concentration limit for the absorbance versus concentration calibration curve was set at 50 mg/dL with a coefficient of determination close to unity for a linear fit (R2 = 0.93).
Kinetic and diffusive measurements
Urea concentration sampling and monitoring were carried at 37 °C using a volumetric ratio of 1:10 for the reactant (immobilized and free bacteria) to reaction medium. Urea degradation was carried out in a hot shaker set to 100 rpm and 37 °C throughout a sampling period of 120 min every 15 min in triplicate. The concentration (Cs) of the urea in the supernatant was monitored by sampling 200 µL every 15 min for two hours.
Baseline control experiments were conducted with empty capsules in uremic media by monitoring the concentration ratio (CS/CS0) where (CS0) is the urea concentration in the supernatant prior to diffusion under stagnant conditions.
Statistical analysis
Pairwise comparisons of urea degradation profiles between the biofabrication schemes and free bacteria were conducted using Excel 2013 software. The metric examined was the slope of the concentration profile from the beginning of the experiment until the 15 min sampling time. A one-tailed Student t-test at the 98.3% confidence interval using the Bonferroni adjustment method was chosen as the discrimination criteria [Citation44].
Simulation
A mathematical simulation of reaction and diffusion was conducted to obtain the radial concentration (C) of urea inside a single capsule as a function of time using Comsol v.5.2.r. The fundamental equation used was the convection–diffusion reaction [Citation36] applied to a single capsule incubated in uremic medium for 15 min. The two-dimensional mesh generation system was used to solve the partial differential equation (EquationEquation (4)(4)
(4) ) numerically using a finite-element method. Diffusivities were obtained by scaling those of fluorescent markers used in previous studies [Citation34] to that of urea and keeping the ratio of membrane to core diffusivity constant. The first-order kinetic constant (K) associated with reaction (R) was varied to reflect the difference in catalytic activity between the first- and second-generation E. coli. Simulation inputs are tabulated in :
(4)
(4)
Table 2. Simulation inputs for single capsule convection–diffusion reaction modelling.
The experimentally determined encapsulation efficiency was factored into the models. Assuming no mass transfer limitations, the bulk concentration (CB) set equal to the initial surface concentration (CS0) was assumed to be constant throughout the simulation set to 16.67 mol/m3 equivalent to a concentrated solution of 1 g/L of glucose. The value of partition coefficient (Kpart) was set to unity since the Stokes’ radius of urea is significantly smaller than the cross-linked membrane pore sizes [Citation34]. The mass transfer coefficient (Kf) was assigned a value of 0.05 m/s throughout the diffusive layers, this norm being inconsequential due the imposed net zero flux boundary conditions (multiple boundaries).
Results
Microcapsule fabrication and size measurement
Shown in are MA, MI and DDMI artificial cells. Measured diameters and determined encapsulation efficiencies are presented in .
Figure 2. Immobilized E. coli DH5α (pKAU17). (A) Atomized macrocapsules (MA) with an average size of 1370 μm ± 60 μm. (B) Inkjet-printed microcapsules (MI) with an average size of 52 μm ± 2.7 μm. (C) Double encapsulated capsules (DDMI) with an average size of 1558 μm ± 125 μm. Arrows indicate sample bacterium location.

Table 3. Size measurements and encapsulation efficiencies for the different biofabrication schemes.
Growth curve
Illustrated in is the growth curve and the timing of induction log phase bacteria (Generation 1). Shown in is the growth curve for the induced bacteria (Generation 2). Log phase bacteria from Generation 2 were used throughout the microencapsulation process. The growth constant (kp) for second generation bacteria was calculated to be 0.0014 (s−1).
Membrane integrity in physiological media
Shown in are images of MA, MI and DDMI artificial cells before and after one hour incubation in the urea reaction medium. As seen in , the MA capsules underwent significant membrane degradation, while, random degradation of the MI capsules was observed. Shown in is the outer shell degradation of the double membrane in the DDMI capsules consistent with the observations in ; meanwhile, the inner membrane of the MI remained intact. Random sampling indicates degradation of the MA capsules as early as 15 min in uremic media. Hence, in order to separate catalysis from membrane degradation, kinetic calculations were confined to a maximum of 15-min reaction time.
Baseline diffusion measurements
Presented in , are the concentration ratios for the MI, DDMI and MA capsules where the highest decrease in bulk concentration is illustrated for the inkjet capsules ((CS/CS0)DDMI = 0.49) the Stokes’ radius of urea (MW= 60.06 g/gmole) is 0.29 nm which is smaller than the reported 70 kDa molecular weight cut-offs for both MA and MI capsules types [Citation34] and the associated membrane pore sizes of 5–7 nm [Citation45].
Kinetic studies
The kinetic constant (K) for the first-order urea degradation also used for the simulation of first generation and second generation bacteria were calculated to be 0.01 (s−1) and 0.00123 (s−1), respectively. Presented in , are comparative urea degradation profiles adjusted to the volume of alginate used on which a Student t-test was conducted at the 15-min sampling interval. Results are summarized in . According to the analysis, the samples closest in behaviour are the free bacteria and the MI capsules (p = .0264). Also, there is a significant difference in degradation profile between the free versus MA and the free versus DDMI schemes.
Table 4. Statistical pairwise comparisons of biofabrication schemes.
Based on the dataset above, values of the effectiveness factor η were generated by dividing the reduction in urea concentration for the encapsulated bacteria by that of the free bacteria. Results are summarized in . As indicated by the values of η less than unity for all three immuno-isolation schemes, urea degradation is diffusion limited for the second generation bacteria with the highest effectiveness factor associated with the MI membrane (η = 0.83) and consistent with the concentration ratio (CS /CS0) generated in baseline diffusion experiments . Meanwhile, the previous gap in the baseline concentration ratios ((CS /CS0)DDMI = 0.65) vs. ((CS/CS0)MA = 0.89) is not reflected by the norm of the equivalent effectiveness factors (ηMA = 0.28 ≤ ηDDMI = 0.34).
Table 5. Kinetic data and effectiveness factors for the free and encapsulated E. coli DH5α (pKAU17).
Simulation
Shown in are simulation results for the second and first generation immobilized E. coli DH5α (pKAU17). Based on , a twofold comparison can be drawn between the degradation behaviour as a function of encapsulation method: At both levels of catalytic activity, the MI capsule () is significantly less diffusion-limited than the MA capsule (), and the first-generation encapsulated bacteria (, second row and , fourth row) is more effective in (lowering the urea concentration than the second generation (), first row and , third row).
Figure 7. Time lapse simulations of radial urea degradation in single capsules for second and first generation immobilized E. coli DH5α (pKAU17). (A) First row a (t = 0s), b (t = 420s), c (t = 900s), d (t = [60s, 240s, 480s, 720s, 900s], (K = 0.00123 s−1), and second row a (t = 0s), b (t = 420s), c (t = 900s) and d (t = [60s, 240s, 480s, 720s, 900s]) (K = 0.01s−1) for (MI) capsules. (B) Third row a (t = 0s), b (t = 420s), c (t = 900s), d (t = [60s, 240s, 480s, 720s, 900s], (K = 0.00123 s−1), and fourth row a (t = 0s), b (t = 420s), c (t = 900s) and d (t = [60s, 240s, 480s, 720s, 900s]) (K = 0.01 s−1) for (MA) capsules.
![Figure 7. Time lapse simulations of radial urea degradation in single capsules for second and first generation immobilized E. coli DH5α (pKAU17). (A) First row a (t = 0s), b (t = 420s), c (t = 900s), d (t = [60s, 240s, 480s, 720s, 900s], (K = 0.00123 s−1), and second row a (t = 0s), b (t = 420s), c (t = 900s) and d (t = [60s, 240s, 480s, 720s, 900s]) (K = 0.01s−1) for (MI) capsules. (B) Third row a (t = 0s), b (t = 420s), c (t = 900s), d (t = [60s, 240s, 480s, 720s, 900s], (K = 0.00123 s−1), and fourth row a (t = 0s), b (t = 420s), c (t = 900s) and d (t = [60s, 240s, 480s, 720s, 900s]) (K = 0.01 s−1) for (MA) capsules.](/cms/asset/45fefd84-d760-450f-823b-f63df2b63da5/ianb_a_1469026_f0007_c.jpg)
At low levels of catalytic activity (K = 0.00123 s−1) diffusion (,), first row) dominates over reaction while at higher levels (K = 0.01 s−1) reaction takes place close the surface () second row) faster than the diffusion time scale.
Discussion
Destabilization of calcium cross-linked junctions in physiological environments reported by many sources [Citation46,Citation47] is the primary root cause of membrane disintegration: the mechanical stability of the ionically crosslinked alginate microcapsules will erode since the extracellular concentration of monovalent cations and chelators exceeds the concentration of the divalent calcium cations, resulting in the exchange by diffusion of the non-gelling cations and subsequent weakening of the ionic bond. In addition to contributing as chelating agents of calcium in forms of calcium phosphate or calcium carbonate phosphate, it has been shown that carbonate and phosphate ions present in the reaction medium, function in the β elimination reaction owing to the general base-catalyzed nature of the alginate depolymerization reaction [Citation48]. Researchers have shown that purified non-crosslinked alginates in solution are depolymerized primarily by acid catalysed hydrolysis and alkaline catalysed mechanisms leading to the cleavage of the glycosidic bond [Citation49,Citation50]. Theoretically, the MI capsules should have not have degraded to the same extent because a higher concentration of cross-linker has been used for preparation (10% (w/v) CaCl2 for MI vs. 1.5% (w/v) CaCl2 for MA) and the membrane was coated with chitosan while cross-linking in the receiving solution. However, a lower molecular weight, mechanically weaker alginate (LV alginate) instead of MV alginate was used for inkjet printing of the MIs. The membrane thickness of both formulations have been measured to be in the 5–7 nm range [Citation34], and thus not a factor in this case for membrane disintegration.
Diffusion-limited kinetics contradicts previous findings at least for the atomization biofabrication scheme [Citation7,Citation51]. A threefold explanation could be offered for the sources of discrepancy: (1) Growth behaviour described by first-order kinetics was designed to detect the difference between the diffusive capacities of membranes. In studies referred to above urea degradation was characterized by zero-order kinetics; (2) Since no antibiotics were used to select for the pKAU17 ampicillin-resistant plasmid, loss of plasmid maybe pronounced in the catalytic activity of the second-generation bacteria; and (3) In the current study, kinetic studies were conducted immediately after encapsulation. Thus, lack of acclimation to the immuno-isolated environment manifested as temporary loss of proliferative ability/cellular activity is being mistaken for diffusion limitations. Regarding the latter, it has been demonstrated that the lag between free and micro-encapsulated growth curves is microorganism-dependent [Citation16].
Given the fact the membrane diffusivity of the MIs (4.3 × 10−13 m2/s) is approximately half that of MAs (7.3 × 10−13 m2/s), the baseline concentration ratio should have been lower for the MAs (CS /CS0 = 0.89) than the MIs (CS /CS0 = 0.49) as reflected in . This suggests that the effect of adsorption–concentration polarization due to the higher surface area of the MAs prior to diffusion is rate-limiting. When coupled with convection and catalysis as shown in and the experimental trends remain unchanged (ηMA = 0.28 < ηMI = 0.83). The difference in concentration ratios detected in baseline diffusion experiments between the MA (CS /CS0 = 0.89) and DDMI capsules (CS /CS0 = 0.65) could be attributed to the individual permeable MI nodes close to the surface within the former immuno-isolation scheme. As for the equivalence of the effectiveness factors (ηDDMI ≅ η MA) more experiments need to be conducted in order to separate the postulated effect of adsorption–concentration polarization from the aforementioned lack of acclimation of the bacteria to the double-encapsulated structure. Integrating the above-stated hypothesis and experimental findings it can be extrapolated that although the bulk measured concentration (CB) is decreasing the capsules’ surface concentration remains constant. This premise gives in turn validity to the Dirichlet boundary condition (CB = CS0) due to capsule symmetry and an infinite concentration used to model diffusion using a Fickian-based model (EquationEquation (4)(4)
(4) ). The ranking of the experimentally determined effectiveness factor (ηMI > ηMA) is consistent with simulation trends illustrated in (top row) in terms of the atomized membrane exhibiting the highest diffusion barrier. As stated above, the simplest pore transport model has been used in this study. Future efforts will encompass examining the effects of the polarization layer at the capsule membrane vicinity proposed by the Pore and Polarization Transport Model consisting of the extended Nerst–Planck equation and the partitioning of the membrane solution interfaces [Citation52,Citation53]. Coupled to the aforementioned model are recent findings of the use of alginate in reverse osmosis research for shedding light on the mechanisms of concentration polarization and electrolyte rejection [Citation54–56].
The 3 X increase in effectiveness factor (ηMI/ηMA) as a result of miniaturization could be further enhanced by switching from microspheres to microfibers. The ratio of experimentally measured membrane diffusivities for alginate-based MIs [Citation35] and microfibers [Citation57,Citation58] for the 4 kDa fluorescent marker is 264 (2.03 × 1 0−11 m2/s/7.7 × 10−14 m2/s). The emergence of composite living microfiber technology in the fields of tissue engineering and regenerative medicine has simultaneously addressed the benefits of increasing the aspect ratio as well overcoming the susceptibility to swelling and degradation. [Citation59,Citation60]. While the current emphasis of cutting-edge 3 D Bio-Printing and additive manufacturing technologies is on bio-ink formulation [Citation61–63] layer by layer deposition is still enabled by ionotropic gelation of alginate susceptible to swelling in physiological media.
Conclusions
The comparative urea degradation potential of second generation log-phase E. coli DH5α (pKAU17) in atomized (MA), inkjet-printed (MI) and double-encapsulated inkjet-printed (DDMI) alginate-based capsules was conducted in in uremic media. Fastest urea diffusion and degradation was observed for the MI capsule (d = 52 μm ± 2.7 μm) characterized by an effectiveness factor of 0.83, a 3 X improvement over the urea degradation potential of MA capsules (d = 1370 μm ± 60 μm) in alignment with simulation trends. All membranes underwent partial degradation between 15 min in uremic media, with the DDMI capsules (d = 1558 μm ± 125 μm) offering complete protection against cell leakage due to the secondary containment as compared to the atomization scheme. Although the double-encapsulation is not an original approach in drug, cell immobilization/implantation research, the feasibility of this biofabrication concept for miniaturized (≤100 μm) alginate-based capsules has not been documented in literature and can be extended to immobilization of other cell lines.
E. coli DH5α (pKAU17) growth under acclimated conditions for all three biofabrication schemes elaborated and genipin cross-linking to confer anti-swelling properties and extend membrane survival in uremic media will be encompassed in future studies. Furthermore, experimental measures will be taken to separate concentration polarization from pure diffusion in order to examine the validity of the proposed Fickian model.
Acknowledgements
The authors would like to acknowledge the C-SUPERB Joint Venture Grant “Bio-Printing of Mammalian Cells” (2011–2012) for funding this effort.
Disclosure statement
No potential conflict of interest was reported by the authors.
Additional information
Funding
References
- Chang TMS. Artificial cells: biotechnology, nanomedicine, regenerative medicine, blood substitutes, bioencapsulation and cell/stem cell therapy. Regenerative Med Artificial Cells Nanomed. 2007;1:130–251.
- Chang TMS. Selected topics in nanomedicine. Regenerative Med, Artificial Cells Nanomed. 2013;3411–428.
- Chang TMS, Wang Y. Biodegradable nanocapsules containing a nanobiotechnological complex for the in-vitro suppression of a melanoma cell line B16F10. J Nanosci Curr. 2016;1:102.
- Jones ML, Martoni CJ, Parent M, et al. Cholesterol-lowering efficacy of a microencapsulated bile salt hydrolase-active lactobacillus Reuteri NCIMB 30242 yoghurt formulation in hypercholesterolaemic. Br J Nutr. 2012;107:1505–1513.
- Strand BL, Coron AE, Skjak-Braek G. Current and future perspectives on alginate encapsulated pancreatic islet. Stem Cells Transl Med. 2017;6:1053–1058.
- Paul A, Chen G, Khan A, et al. Genipin-cross-linked microencapsulated human adipose stem cells augment transplant retention resulting in attenuation of chronically infarcted rat heart fibrosis and cardiac dysfunction. Cell Transplant. 2012;21:2735–2751.
- Prakash S, Chang TMS. Microencapsulated Genetically Engineered Live E. Coli DH5 cells administered orally to maintain normal plasma urea level in uremic rats. Nat Med. 1996;2:893–887.
- Zajkoska P, Rebroš M, Rosenberg M. Biocatalysis with immobilized Escherichia coli. Appl Microbiol Biotechnol. 2013;97:1441–1455.
- Prakash S, Chang TMS. Preparation and in vitro analysis of microencapsulated genetically engineered E. Coli DH5 cells for urea and ammonia removal. Biotechnol Bioeng. 1995;46:621–626.
- Thomas Ming Swi C. High throughput methods for miniaturization of implantable artificial cells. In: Selected topics in nanomedicine: regenerative medicine, artificial cells and nanomedicine. Singapore: World Scientific; 2013. p. 411–427.
- Chen W, Kim J-H, Zhang D, et al. Microfluidic one-step synthesis of alginate microspheres immobilized with antibodies. J R Soc. 2013;10:20130566.
- Choi C-H, Wang H, Lee H, et al. One-step generation of cell-laden microgels using double emulsion drops with a sacrificial ultra-thin oil shell. Lab Chip. 2016;16:1549–1555.
- Velasco D, Tumarkin E, Kumacheva E. Microfluidic encapsulation of cells in polymer microgels. Small. 2012;8:1633–1642.
- Ahn J, Sei YJ, Jeon NL, et al. Tumor microenvironment on a chip: the progress and future perspective. Bioengineering. 2017;4:64.
- Zhang W, Yang G, Zhang A, et al. Preferential vitrification of water in small (∼100 ⌠m) alginate microcapsules significantly augments cell cryopreservation by vitrification. Biomed Microdevices. 2010;12:89–96.
- Mobed-Miremadi M, Dharba S. Immobilization of R. erythropolis in alginate-based artificial cells for simulated plaque degradation in aqueous media. J Microencapsul. 2014;31:115–126.
- Zahn JD. Methods in bioengineering: biomicrofabrication and biomicrofluidics. Norwood (MA): Artech House. 2010.
- Agarwal P, Zhao S, Bielecki P, et al. One-step microfluidic generation of pre-hatching embryo-like core-shell microcapsules for miniaturized 3D culture of pluripotent stem cells. Lab Chip. 2013;13:4525–4533.
- Szymanski JM, Feinberg AW. Fabrication of freestanding alginate microfibers and microstructures for tissue engineering applications. Biofabrication. 2014;6:024104
- Zhang W, He X. Encapsulation of living cells in small (∼100 μm) alginate microcapsules by electrostatic spraying: a parametric study. J Biomech Eng. 2009;131:074515–074515-6.
- Park H, Kim PH, Hwang T, et al. Fabrication of cross-linked alginate beads using electrospraying for adenovirus delivery. Int J Pharm. 2012;427:417–425.
- Russo R, Malinconico M, Santagata G. Effect of cross-linking with calcium ions on the physical properties of alginate films. Biomacromolecules. 2007;8:3193–3197.
- Andersen T, Strand BL, Formo K, et al. Alginates as biomaterials in tissue engineering carbohydr. Chem. 2012;37:227–258.
- Skjak-Braek G, Murano E, Paoletti S. Alginate as immobilization material II: Determination of polyphenol contaminants by fluorescence spectroscopy, and evaluation of methods for their removal. Biotechnol Bioeng. 1989;33:90–94.
- Wong H, Chang T. A novel two-step procedure for immobilizing living cells in microcapsules for improving xenograft survival. Biomaterials, Artif Cells Immoboli Biotechnol. 1991;19:687–697.
- Jeon O, Bouhadir KH, Mansour JM, et al. Photocrosslinked alginate hydrogels with tunable biodegradation rates and mechanical properties. Biomaterials. 2009;14:2724–2734.
- Liang HF, Hong MH, Ho RM, et al. Novel method using a temperature-sensitive polymer (methylcellulose) to thermally gel aqueous alginate as a pH-sensitive hydrogel. Biomacromolecules. 2004;5:1917–1925.
- He SL, Yaszemski MJ, Yasko AW, et al. Injectable biodegradable polymer composites based on poly(propylene fumarate) crosslinked with poly(ethylene glycol)-dimethacrylate. Biomaterials. 2000;21:2389–2394.
- Sun J, Tan H. Alginate-based biomaterials for regenerative medicine applications. Materials. 2013;6:1285–1309.
- Chen H, Ouyang W, Lawuyi B, et al. Reaction of chitosan with genipin and its fluorogenic attributes for potential microcapsule membrane characterization. J Biomed Mater Res. 2005;75:917–927.
- Paredes-Juarez GA, Haan BJ, Faas MM, et al. A technology platform to test the efficacy of purification of alginate. Materials. 2014;7:2087–2103.
- Shan Y. Viability of Microencapsulation of E. Coli DH5 for urea removal using inkjet bio-printing. San Jose (CA): San Jose State University; 2011.
- Vegas AJ, Veiseh O, Gürtler M, et al. Long-term glycemic control using polymer-encapsulated human stem cell-derived beta cells in immune-competent mice. Nat Med. 2016;22:306–311.
- Mobed-Miremadi M, Asi B, Parasseril J, et al. Comparative diffusivity measurements for alginate-based atomized and inkjet-bioprinted artificial cells using fluorescence microscopy. Artif Cells Nanomed Biotechnol. 2013;41:196–201.
- Smith JM. Chemical engineering kinetics. New York City (NY): McGraw-Hill; 1970.
- RL. Fournier Basic transport phenomena in biomedical engineering. 3rd ed. Boca Raton (FL): CRC Press; 2011.
- Mulrooney SB, Pankratz HS, Hausinger RP. Regulation of gene expression and cellular localization of cloned klebsiella aerogenes (K. pneumoniae). Urease Microbiol. 1989;135:1769–1776.
- Lee MH, Mulrooney SB, Renner MJ, et al. Klebsiella aerogenes urease gene cluster: sequence of ureD and demonstration that four accessory genes (ureD, ureE, ureF, and ureG) are involved in nickel metallocenter biosynthesis. J Bacteriol. 1992;174:4324–4330.
- Carter EL, Flugga N, Boer JL, et al. Interplay of metal ions and urease. Metallomics. 2009;1:207–221.
- Selvarasu S, Ow DS-W, Lee SY, et al. Characterizing escherichia coli DH5a growth and metabolism in a complex medium using genome-scale flux analysis. Biotechnol Bioeng. 2009;102:923–934.
- Garofalo FA, Chang TMS. Immobilization of P. pictorum in open pore agar, alginate and polylysine-alginate microcapsules for serum cholesterol depletion. Biomater Artif Cells Artif Org. 1989;17:271–289.
- Mobed-Miremadi M, Acks E, Polasaward S, et al. High throughput miniaturization of artificial cells. Artif Cells Blood Substit Biotechnol. 2011;39:310–316.
- Guyton AC. 1991. Textbook of medical physiology. Philadelphia (PA): WB Saunders;
- King M, Mody N. Numerical and statistical methods for bioengineering. Cambridge (GBR): Cambridge University Press; 2011.
- Simpliciano C, Clark L, Asi B, et al. Cross-linked alginate film pore size determination using atomic force microscopy and validation using diffusivity determinations. Jsemat. 2013;3:1–12.
- Lee KY, Alsberg E, Mooney DJ. Degradable and injectable poly(aldehydeguluronate) hydrogels for bone tissue engineering. J Biomed Mater Res. 2001;56: 228–233.
- Malafaya PB, Silva GA, Reis RL. Natural-origin polymers as carriers and scaffolds for biomolecules and cell delivery in tissue engineering applications. Adv Drug Deliv Rev. 2007;59: 207–233.
- Haug A, Larsen B, Smidsrød O, et al. Alkaline degradation of alginate. Acta Chem Scand. 1967;21:2859–2870.
- Holme HK, Davidsen L, Kristiansen A, et al. Kinetics and mechanisms of depolymerization of alginate and chitosan in aqueous solution. Carbohyd Polym. 2008;73:656–664.
- BHA. Rehm Alginates: biology and Applications. Springer-Verlag Berlin Heidelberg: Springer; 2009.
- Chang TM, Prakash S. Artificial cells for bioencapsulation of cells and genetically engineered E. coli: for cell therapy, gene therapy, and removal of urea and ammonia. Methods Molecul Biol. 1997;63:343–358.
- Bhattacharjee S, Chen JC, Elimelech M. Coupled model of concentration polarization and pore transport in crossflow nanofiltration. AIChE J. 2001;47:2733–2745.
- Déon S, Dutournié P, Fievet P, et al. Concentration polarization phenomenon during the nanofiltration of multi-ionic solutions: influence of the filtrated solution and operating conditions. Water Res. 2013;47:2260–2272.
- Sondus J, Chang S, Zhou H. Filtration behaviour and fouling mechanisms of polysaccharides. Membranes. 2014;4:319–332.
- Marpani F, Luo J, Valentina Mateiu R, et al. In Situ formation of a biocatalytic alginate membrane by enhanced concentration polarization. ACS Appl Mater Interfaces. 2015;7:17682–17691
- Tow EW, Lienhard V, John H. Unpacking compaction: effect of hydraulic pressure on alginate fouling. J Membr Sci. 2017;544:221–233.
- Djomehri S, Zeid H, Yavari A, et al. Simulation and Verification of Macroscopic Isotropy of Hollow Alginate-Based Microfibers. Artif Cells Nanomed Biotechnol. 2015;43:390–397.
- Mobed-Miremadi M, Djomehri S, Keralapura M, et al. Fickian-based empirical approach for diffusivity determination in hollow alginate-based microfibers using 2D fluorescence microscopy and comparison with theoretical predictions. Materials. 2014;7:7670–7688.
- Akbari M, Tamayol A, Laforte V, et al. Composite living fibers for creating tissue constructs using textile techniques. Adv Funct Mater. 2014;24:4060–4067.
- Motealleh B, Zahedi P, Rezaeian I, et al. Morphology, drug release, antibacterial, cell proliferation, and histology studies of chamomile-loaded wound dressing mats based on electrospun nanofibrous poly(ɛ-caprolactone)/polystyrene blends: Morphology, Drug Release, Antibacterial, Cell Proliferation, and Histology Studies. J Biomed Mater Res. 2014;102:977–987.
- Ji S, Guvendiren M. Recent Advances in Bioink Design for 3D Bioprinting of Tissues and Organs. Front Bioeng Biotechnol. 2017;5:23.
- Zhang YS, Yue K, Aleman J, et al. 3D bioprinting for tissue and organ fabrication. Ann Biomed Eng. 2017;45:145–163.
- Yang X, Lu Z, Wu H, et al. Collagen-alginate as biolink for three-dimensional (3D) cell printing based cartilage tissue engineering. Mater Sci Eng C Mater Biol Appl. 2018;83:195–201.