Abstract
Skate (Dipturus chilensis) cartilage extract was utilized as a green reducing agent for the synthesis of spherical gold nanoparticles with an average size of 16.7 ± 0.2 nm. The gold nanoparticle solution showed a surface plasmon resonance at 543 nm with a wine-red colour. A strong X-ray diffraction pattern and clear lattice structure in high-resolution transmission electron microscopy indicated a face-centred cubic structure of the gold nanoparticles. The gold nanoparticles retained excellent colloidal stability. Gold nanoparticles showed strong antioxidant activity in terms of 2,2-diphenyl-1-picrylhydrazyl radical scavenging activity. In vitro cytotoxicity was observed for seven cancer cells assessed by the water-soluble tetrazolium assay. Among the seven cancer cells, the highest cytotoxicity was observed for MDA-MB-231 (human breast adenocarcinoma cell) followed by HeLa (human epithelial cervix adenocarcinoma cell) and lastly by HT-29 (human colorectal adenocarcinoma cell). Furthermore, gold nanoparticles showed excellent haemocompatibility, indicating the possibility of their use as a future nanomedicine. These results strongly suggest that gold nanoparticles green-synthesized by upcycling skate cartilage waste extract will be valuable carriers or vehicles for the delivery of drugs or bioactive molecules, such as anti-cancer agents, for the treatment of cancers.
Introduction
Natural products have been beneficially used in many areas of research. Specifically, natural products have been proposed to offer many advantages for use in green nanotechnology. As reducing and stabilizing agents, natural products have been used for the green synthesis of metallic nanoparticles to perform a reduction reaction of metal salts to nanoparticles. Plant extracts are commonly selected for this purpose [Citation1–4].
Among metallic nanoparticles, gold nanoparticles (AuNPs) have attracted intense interests and been widely applied in drug delivery, photothermal therapy, photodynamic therapy, cancer diagnosis, catalysis in chemical reactions, and sensing of chemicals and biological molecules [Citation5–9]. The major advantages of AuNPs include the following: (i) their biocompatibility is excellent; (ii) cytotoxicity is low; (iii) surface modification is very facile, as sulfhydryl, disulphide and amine functional groups exhibit specific binding to the surface; (iv) the synthesis is simple because the reduction reaction of Au salts to AuNPs can be directly performed in one-pot and/or as a one-step reaction; and finally (v) the AuNPs themselves possess biological activities, including anticancer, anti-inflammatory and antioxidant effects [Citation10,Citation11].
A green synthetic process uses green reducing agents to change metal salts to their corresponding nanoparticles; natural products play a key role as reducing and stabilizing (or capping) agents. The green synthetic strategy eliminates the use of chemical reducing agents and minimizes chemical interferences for in vitro and in vivo experiments with metallic nanoparticles. The goal to further the global sustainability initiatives can be entirely accomplished. In our laboratory, we synthesized AuNPs and silver nanoparticles (AgNPs) using diverse natural products by green strategies. Excellent reviews were reported by the author about the utilization of plant extracts and polysaccharides in the green synthesis of AuNPs and AgNPs [Citation12]. We have used plant extracts (Artemisia capillarys [Citation13], Leonurus japonica [Citation14], Polygala tenuifolia [Citation15,Citation16], Caesalpinia sappan [Citation17,Citation18], Bupleurum falcatum [Citation19], and Galcinia mangostana [Citation20]), invertebrate animal extracts (Eisenia andrei and Achatina fulica) [Citation21], pure compounds from plant origins (resveratrol [Citation22], tannic acid [Citation23,Citation24], rosmarinic acid [Citation25], caffeic acid [Citation26], chlorogenic acid [Citation27], gallic acid [Citation28] and catechin [Citation29]) and pure compounds from animal origins (heparin [Citation30], chondroitin sulfate [Citation31,Citation32], acharan sulfate [Citation31] and sialyllactose [Citation33]). Medicines, such as ampicillin [Citation34], vancomycin [Citation35] and ethambutol [Citation36], were also utilized as reducing agents. Most interestingly, the prepared AuNPs and AgNPs generated augmented biological activities, suggesting that a combination of metallic nanoparticles and natural products created synergism to exert their biological activities. However, the use of the by-products of marine organisms is rarely employed for the green synthesis of metallic nanoparticles, even though marine organisms contain diverse active biomolecules.
In our previous report, we isolated and characterized chondroitin sulphates from the by-products of marine organisms, including skate, salmon, yellow goosefish and flatfish [Citation37]. Chondroitin sulphate is a glycosaminoglygan, which is a type of linear polysaccharide with a high degree of sulphation. Cartilages and bones of these marine organisms contain chondroitin sulphates.
Herein, the objective of the present report is to synthesize AuNPs using skate (Dipturus chilensis) cartilage waste extract as a green reducing agent. Before synthesizing AuNPs, an amino acid composition analysis of the extract was performed to determine information regarding the major amino acids that contribute to the synthesis. Next, various spectroscopic and microscopic approaches were employed to characterize the AuNPs. The optical properties were monitored by UV–visible spectrophotometry to observe surface plasmon resonance (SPR). High-resolution X-ray diffraction (HR-XRD) was typically performed to determine the crystalline nature of the nanoparticles. The reaction yield was estimated by inductively coupled plasma optical emission spectroscopy (ICP-OES). High-resolution transmission electron microscopy (HR-TEM) was a very convenient tool to visualize nanoparticles. The size of the nanoparticles in the solution was measured by dynamic light scattering (DLS) to obtain their hydrodynamic size. The colloidal stability was observed by acquiring UV–visible spectra, measuring the hydrodynamic size and zeta potentials, and obtaining HR-TEM images. Then, the in vitro antioxidant activity was assessed by 2,2-diphenyl-1-picrylhydrazyl (DPPH) radical scavenging activity. The in vitro cytotoxicity was screened using the following seven cancer cells with a water-soluble tetrazolium assay (WST assay); A549 (human lung carcinoma cell), AGS (human gastric adenocarcinoma cell), HeLa (human epithelial cervix adenocarcinoma cell), HepG2 (human hepatocyte carcinoma cell), HT29 (human colorectal adenocarcinoma cell), MDA-MB-231 (human breast adenocarcinoma cell) and PANC-1 (human pancreas ductal adenocarcinoma cell). Additionally, an evaluation of the haemocompatibility was conducted using human blood cells to consider the possibility for a future use in nanomedicine.
Materials and methods
Preparation of the extract
The product of gold nanoparticles synthesized with skate cartilage extract will be referred to hereafter as sk-AuNPs. The skate was purchased and prepared according to our previous report [Citation37]. Briefly, skate cartilage was cleaned, lyophilized and ground. The ground powder of skate cartilage was extracted with boiled water for 30 min. After boiling, a supernatant was pooled after the centrifugation process. Then, lyophilized powder was obtained by freeze-drying. The freeze-dried powder (399.6 mg) was dissolved in de-ionized water (19.98 ml) and vortexed for 1 min. This solution was subjected to sonication for 10 min and then centrifuged (3000 g force, 20 min, 18 °C). After centrifugation, the supernatant was pooled and filtered using a cellulose acetate syringe filter (pore size 0.45 μm). The filtrate was lyophilized. This lyophilized powder was labelled ‘the extract’ in the present report and used for the synthesis of sk-AuNPs. The initial freeze-dried powder (399.6 mg) produced the final extract (85.1 mg), which afforded a 21.3% yield. The stock solution (2%, w/v) of the extract was prepared in de-ionized water.
Reagents and instruments
Potassium gold(III) chloride (KAuCl4) was purchased from Sigma-Aldrich (St. Louis, MO). 2,2-Diphenyl-1-picrylhydrazyl (DPPH) was obtained from Alfa Aesar (Heyshham, England). UV–visible spectra were recorded on a Shimadzu UV-2600 (Shimadzu Corporation, Kyoto, Japan). A Rigaku Miniflex high-resolution X-ray diffractometer equipped with a Cu-Kα radiation source (λ = 0.154056 nm) in the range of 20°–80° (2θ scale) was utilized for obtaining the HR-XRD pattern (Rigaku, Tokyo, Japan). HR-TEM images were collected with a JEM-2100F microscopy, which was operated at 200 kV (JEOL Ltd., Tokyo, Japan). A NanoBrook 90Plus Zeta was used to determine the hydrodynamic size and zeta potentials at 20 °C (Brookhaven Instruments, Corporation, Holtsville, NY). The centrifugation was conducted on a 5424 R centrifuge (Eppendorf AG, Hamburg, Germany). Cell morphology was photographed using microscopy (100 × magnification) in a Cytation hybrid multi-mode reader (BioTek Instruments, Winooski, VT).
Amino acid composition analysis of the extract
The amino acid composition analysis was conducted by using the Waters Pico-Tag™ system in the Korea Basic Science Institute (Daejon, Republic of Korea). The Pico-Tag™ reagent kit was purchased from the Waters Corporation (Milford, MA, USA). The extract was hydrolyzed followed by labelling with phenylisothiocyanate (PITC) according to the Waters Pico-Tag™ protocol. The hydrolyzed and PITC-labelled samples were dissolved in the buffer (400 µL, 140 mM of sodium acetate in 6% acetonitrile). The sample was taken (10 µL) and injected onto the Waters high-performance liquid chromatography (HPLC) system. The HPLC system was composed of a Waters 510 pump, Waters gradient controller, Waters automatic sampler, Waters Pico-Tag™ column (3.9 × 300 mm, 4 µm), Waters 2487 UV detector and data analysis system. The PITC-labelled samples were detected at 254 nm. Samples were eluted by a gradient elution with buffers A and B at a flow rate of 1 mL/min for 25 min (buffer A: 140 mM of sodium acetate in 6% acetonitrile, buffer B: 60% acetonitrile). The gradient elution was as follows: 0 min (100% A, 0% B), 9 min (86% A, 14% B), 9.2 min (80% A, 20% B), 17.5 min (54% A, 46% B), 17.7 min (0% A, 100% B), and 21–25 min (100% A, 0% B).
Green synthesis of sk-AuNPs and cf-sk-AuNPs
All stock solutions (2% extract, 10 mM KAuCl4, and 100 mM NaOH) were prepared in de-ionized water. Three variables (i.e. the concentrations of the extract, KAuCl4 and NaOH) were tested to optimize the reaction conditions. One variable was changed, while the other variables were held constant. The UV–visible spectra ranging from 200 to 900 nm were acquired to monitor the reaction progress and completion. We selected the optimal reaction condition when the highest absorbance was gained in the range of 500–550 nm. The optimal reaction condition was as follows; the extract (0.05%), KAuCl4 (0.4 mM) and NaOH (0.6 mM). Each concentration given is the final concentration in a 2 mL total volume. The mixture was vortexed for 10 s and incubated in an 80 °C dry oven for 24 h. This solution was labelled sk-AuNPs. A schematic representation of the synthetic procedure is illustrated in . To remove excess extract, sk-AuNPs were centrifuged (14,500g force, 10 min, 18 °C). The supernatant was removed. The pellet was re-dispersed in the same volume of de-ionized water. This re-dispersed solution was labelled cf-sk-AuNPs.
Estimation of reaction yield for sk-AuNPs
ICP-OES was performed to calculate a reaction yield of the sk-AuNPs. For obtaining the reaction yield, the solution of sk-AuNPs was centrifuged (18,410g force, 7 h, 18 °C) and the supernatant, along with a sample of the original sk-AuNPs, were submitted for ICP-OES analysis.
Assessment of colloidal stability on the shelf
The on-the-shelf colloidal stability of cf-sk-AuNPs was monitored by UV–visible spectrophotometry over 27 d at ambient temperature. UV–visible spectra were acquired at fixed time intervals in the range of 200–900 nm. In addition, the hydrodynamic size determined by DLS and zeta potentials was also obtained.
Assessment of colloidal stability in PBS, DMEM and full media
The colloidal stability of the nanoparticles in several solutions (i.e. PBS, a high glucose Dulbecco’s modified Eagle’s medium (DMEM) and full media) was tested prior to in vitro biological experiments. sk-AuNPs were prepared with the optimized reaction conditions according to the previous section. sk-AuNPs (1 mL) was subjected to ultracentrifugation (14,500g force, 30 min, 18 °C), and the supernatant (900 μL) was discarded. Then, the following solution (900 μL) was added to the pellet: PBS, DMEM and full media. In the DMEM solution, phenol red was excluded as its absorbance overlaps that of sk-AuNPs. For the preparation of full media, FBS (10%) was added to the abovementioned DMEM solution. The re-dispersed solutions were subjected to the assessment of colloidal stability for 48 h by acquiring UV–visible spectra and measuring hydrodynamic size by DLS HR-TEM images were also acquired.
DPPH radical scavenging activity
The DPPH radical scavenging activity of cf-sk-AuNPs was tested to evaluate the in vitro antioxidant activity according to the previous report with slight modifications [Citation20]. The sk-AuNPs solution (1 mL) was centrifuged (18,500g force, 3 h, 18 °C), and the pellet was recovered. Then, de-ionized water was added to the pellet, and the pellet was re-dispersed. When adding de-ionized water, the volume of the added-water was varied to generate five different cf-sk-AuNPs with different Au concentrations (15.7 μg/mL, 31.4 μg/mL, 62.7 μg/mL, 125.5 μg/mL and 251.0 μg/mL). The Au concentration in each sample was determined by ICP-OES analysis (Optima 8300 model, PerkinElmer, Shelton, CT). For the DPPH radical scavenging activity, each sample (50 μL) was mixed with DPPH (0.2 mM, 150 μL) solution, and the incubation was conducted in the dark for 20 min. The absorbance was measured at 517 nm using a multiplate detector reader (Synergy HT, BioTek Instruments, Winooski, VT). A negative control was prepared by mixing de-ionized water with DPPH solution. Vitamin C was used as a positive control. Measurements were performed in triplicate. Further, the resulting antioxidant activity was expressed as the vitamin C equivalent antioxidant capacity (VCEAC) along with the Au concentration of the tested solutions.
Cell culture
Cancer cells were purchased from the Korean Cell Line Bank (Seoul, Republic of Korea). Cells were grown in DMEM supplemented with 10% FBS, penicillin (100 unit/mL) and streptomycin (100 μg/mL) (Gibco BRL, Grand Island, NY). Cells were grown in a 37 °C (supplied 5% CO2) incubator and maintained approximately 80% confluence before trypsinization.
Cytotoxicity
In vitro cytotoxicity (WST assay) was measured to determine the effect of cf-sk-AuNPs on cancer cells. For the WST assay, the EZ-CYTOX kit was obtained from DoGenBio (Gyonggi, Republic of Korea). The cells were seeded on 96-well plates at a density of 5.0 × 103 cells/well. Then, the cells were incubated for 24 h in an incubator at 37 °C under a CO2 (5%) atmosphere. Next, different concentrations of cf-sk-AuNPs (0, 4, 8, 16, 32, 63 and 126 μg/mL, based on the Au concentration) were treated and incubated for another 24 h at 37 °C under a CO2 (5%) atmosphere. The WST reagent (10 μL) from the EZ-CYTOX kit was added and incubated in the CO2 incubator for additional 1 h. The absorbance was measured at 450 nm using a Cytation hybrid multi-mode reader (BioTek Instruments, Winooski, VT). The intrinsic absorbance of sk-AuNPs interfered with the measurement of cytotoxicity at 450 nm. Thus, the background absorbance of sk-AuNPs was subtracted from the absorbance of samples measured at 450 nm. The background absorbance was measured in a WST-free condition. In addition, the AuNP-untreated cells were utilized as a control.
Haemocompatibility assay
For the haemocompatibility assay, blood samples from healthy volunteers were collected into vials treated with heparin and EDTA. The haemolysis experiments were performed using “the American Society for Testing and Materials protocol E2524–08, the standard test method for analysis of haemolytic properties of nanoparticles” with slight modifications. Red blood cells (RBC) were separated by centrifuging fresh blood at 800g for 10 min. Then, the pellet was washed with PBS (pH 7.4) twice. The RBC suspension was prepared by diluting the washed RBC with PBS (pH 7.4) to make a final concentration of 10%, and this suspension was used in the following procedures. Likewise, a positive control was prepared by mixing the RBC suspension (10%, 400 μL) with the same volume of PBS (pH 7.4, 400 μL). For the negative control, distilled water (400 μL) was mixed with the RBC suspension (10%, 400 μL). Different amounts of sk-AuNPs (100 μL and 200 μL) were mixed with the RBC suspension (10%, 400 μL). The final volume was adjusted to 800 μL by adding PBS. The incubation was performed for 60 min at 37 °C on a shaking incubator. After the 60 min-incubation, the samples were centrifuged at 800g for 10 min. Then, the supernatants were again centrifuged at 21,000g for 10 min. UV absorbance of the final supernatant was measured at 540 nm using an ELISA reader (Tecan, Männedorf, Switzerland).
Statistical analysis
All experiments were performed in triplicate, and all data are presented as the mean ± standard deviation (SD). Statistical significance was assessed using Student’s t-test, and values of p < .05 were considered significant.
Results and discussion
Amino acid composition analysis
The amino acid composition analysis of the extract is summarized in . The most abundant amino acids were found to be, in order, glycine (89.2 μg/mg), glutamate/glutamine (49.9 μg/mg), proline (41.6 μg/mg), alanine (35.2 μg/mg) and aspartate/asparagine (30.5 μg/mg). These amino acids composed 54.2% of the total amino acids. The composition was very similar to collagen, whose major amino acids are glycine, proline, hydroxyproline, alanine, glutamate and aspartate [Citation38]. Negative charges of amino acids and chondroitin sulphates also provided excellent colloidal stability by generating large negative zeta potentials of sk-AuNPs.
Table 1. Amino acid composition analysis of the extract.
UV–visible spectra
In the search for optimal reaction conditions, three variables (concentrations of Au salts, the extract and NaOH) were varied. After the concentration variables were optimized, the reaction time and reaction temperature were optimized. One variable was varied, while the others were held constant. The synthetic progress and completion were followed by obtaining UV–visible spectra. The observation of SPR indicated that the synthesis of AuNPs was successful. As shown in , one SPR band was observed at 543 nm, suggesting the formation of spherical AuNPs. The colour of the solution changed from pale yellow to wine-red after the synthesis (, inset).
Reaction yield
The reaction yield was estimated by ICP-OES analysis via an indirect way. Total Au concentration was measured by analyzing the sk-AuNPs solution. The unreacted Au concentration was determined by analyzing the supernatant after centrifugation of the sk-AuNPs. The reaction yield of the sk-AuNPs determined by ICP-OES was 85.4%.
HR-XRD analysis
HR-XRD analysis efficiently provides evidence of the crystallinity of metallic nanoparticles. The observation of distinct diffraction angles (2θ) was a key clue to determine a crystalline structure. The HR-XRD pattern of the cf-sk-AuNPs is shown in . Four diffraction angles at 38.1°, 44.2°, 64.6° and 77.7° were observed that corresponded to the (111), (200), (220) and (311) planes of the face-centred cubic (fcc) structure, respectively. Among these four planes, the (111) plane was the most intense, which suggested that (111) was the main plane of the fcc structure. The estimation of particle size was calculated by employing the Scherrer equation (D = 0.89·λ/W·Cosθ) based on the (111) plane. Each term in the Scherrer equation is defined as follows: D is the particle size, W is the full-width at half-maximum, θ is the diffraction angle and λ is the wavelength of the X-ray used. The estimated size from the equation was calculated as 14.6 nm. This size was very close to that of the HR-TEM size measurement (16.7 ± 0.2 nm), which will be discussed in the following section.
HR-TEM images
HR-TEM provides useful information regarding the size and shape of the AuNPs. As demonstrated in the HR-TEM images of the sk-AuNPs, the spherical AuNPs were clearly visualized in . The average size was measured from 150 discrete nanoparticles in the images using Image J software (NIH Image J system, Bethesda, MD), which was 16.7 ± 0.2 nm (). A Gaussian distribution was observed in the size histograms. The crystalline structure was also clearly observed in one enlarged nanoparticle in . The distance between two neighbouring lattices were measured as 0.23 nm and 0.25 nm, suggesting that the (111) plane was dominant in the fcc structure. This result was well corroborated by the HR-XRD result in the previous section.
Figure 4. HR-TEM images of sk-AuNPs. The scale bars represent (A) 20 nm, (B) 50 nm and (C) 2 nm. The distances between two neighbouring lattice fringes were measured as 0.23 nm and 0.25 nm. (D) Size histogram of sk-AuNPs.
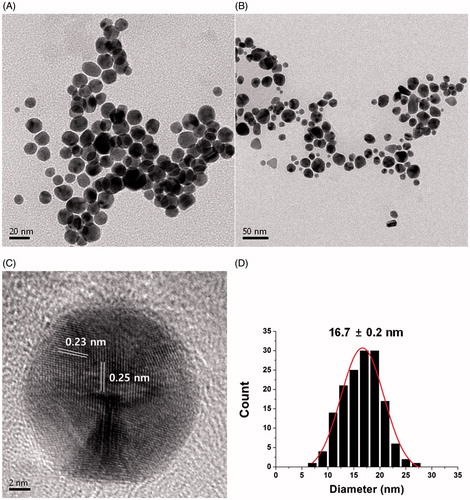
We also obtained three kinds of HR-TEM images of the cf-sk-AuNPs (). After centrifugation of the sk-AuNPs, the pellet was recovered and re-dispersed in (i) deionised water, (ii) PBS and (iii) full media. The spherical shapes of the AuNPs were retained, regardless of the solvent for re-dispersion (). The size did not change significantly after re-dispersion with the three different solvents. The size was measured from 150 discrete nanoparticles as 14.2 ± 0.1 nm with re-dispersion with deionised water (). When PBS was utilized for re-dispersion, the size from 150 discrete nanoparticles was slightly larger (19.5 ± 0.6 nm) (). The mixture of DMEM and 10% FBS produced the average size of 14.9 ± 0.4 nm, which was obtained from 150 discrete nanoparticles ().
On-the-shelf colloidal stability
The colloidal stability of nanoparticles is a significant consideration for their use as therapeutic tools. Nanoparticles must retain their colloidal stability under cell culture conditions and in the bloodstream. Furthermore, the stability on the shelf is also crucial. Thus, on-the-shelf stability of the cf-sk-AuNPs was evaluated for 27 d at ambient temperature. We measured the hydrodynamic size along with the polydispersity index (PDI) and zeta potentials for 27 d (). Upon the synthesis (day 0), the hydrodynamic size was measured as 243.4 nm with a polydispersity index of 0.21. The zeta potential was determined to be −42.8 mV. The hydrodynamic size tended to decrease to 198 nm over 27 d with a minimum size of 185.9 nm. However, there were no significant size changes. In addition, the PDI was in the range of 0.19–0.21 for 27 d, suggesting that the cf-sk-AuNPs were polydisperse in the colloidal solution. The hydrodynamic size was much larger than that of the size measured from HR-TEM images. This discrepancy was because the hydrodynamic size measures the metallic cores along with the extract on the surface. Large negative values of zeta potentials (−37.1 to −44.3 mV) were maintained over 27 d. This result strongly indicated that the cf-sk-AuNPs on the shelf were quite stable in the solution at ambient temperature for 27 d.
Table 2. On-the-shelf colloidal stability of cf-sk-AuNPs for 27 days.
In the previous section, the major amino acids in the extract are glycine, proline, alanine, glutamine, glutamate, asparagine and aspartate. Most likely, the large negative zeta potential originated from the negative charges of glutamate and aspartate. The skate cartilage also contained chondroitin sulphates (our unpublished results); thus, the sulphate groups also endowed the samples with large negative zeta potentials and excellent colloidal stability.
Colloidal stability in PBS, DMEM and full media
As mentioned in the previous section, the colloidal stability is a crucial consideration for the nanoparticles. In this section, the colloidal stability in PBS, DMEM and full media was assessed for in vitro biological experiments. The colloidal stability results in each solution for 48 h are summarized in and . The measurement at the time of synthesis was labelled 0 h. First, when PBS was used for re-dispersion, the maximum SPR was constant at 538 nm for 48 h without showing decreasing UV absorbance ( and ). The shape of SPR retained for the 48 h incubation. The hydrodynamic size was not changed significantly for 48 h (292.4 nm, 298.9 nm and 295.2 nm). The PDI was observed to be 0.27–0.30, indicating that the nanoparticle solution was polydisperse. Second, when DMEM was used for re-dispersion, the maximum SPR was red-shifted from 541 nm (0 h) to 545 nm (48 h) without changing the SPR band shape ( and ). It also did not decrease the UV absorbance. The hydrodynamic size increased from 284.8 nm to 736.8 nm. Over 48 h, the PDI was maintained in the range of 0.24–0.29, suggesting that the nanoparticle solution was polydisperse. Lastly, with a re-dispersion in full media, the maximum SPR did not change significantly (539–540 nm) ( and ). After 48 h incubation in full media, the UV absorbance decreased from 1.19 (0 h) to 0.96 (48 h) while retaining the SPR shape. The hydrodynamic size decreased from 287.5 nm to 266.9 nm and then slightly increased to 272.8 nm. The nanoparticle solution was also polydisperse (PDI 0.27–0.28). From these results, the shape of SPR and hydrodynamic size were retained well for the 48 h incubation period after the re-dispersion of the sk-AuNPs in three solvents (PBS, DMEM and full media). There was no aggregation and/or agglomeration; thus, the sk-AuNPs are highly stable, which is sufficient enough to perform in vitro biological experiments, including those to determine cytotoxicity and haemocompatibility in the present report.
Figure 6. Colloidal stability of sk-AuNPs in PBS, DMEM and full media. sk-AuNPs were centrifuged and re-dispersed in the following solutions: (A and B) PBS, (C and D) DMEM and (E and F) full media. (A, C and E) UV–visible spectra and (B, D and F) hydrodynamic size were measured over 48 h on the shelf.

Table 3. Colloidal stability of sk-AuNPs which were centrifuged and re-dispersed in PBS, DMEM and full media.
In vitro antioxidant activity
The in vitro antioxidant activity was measured by DPPH radical scavenging activity, and the result is shown in and . The DPPH radical scavenging activity has been extensively utilized to observe the capability of AuNPs to perform as free radical scavengers. When the Au concentration was 15.7 µg/mL, the scavenging activity was 9.7%. When the Au concentration was increased 16-fold (251.0 µg/mL), the scavenging activity was enhanced by 6.5-fold (63.2%). This result clearly supports that the DPPH radical scavenging activity showed dose-dependence on the Au concentration. Furthermore, the antioxidant activity of the cf-sk-AuNPs was due to the direct involvement of AuNPs in scavenging the DPPH radicals. The DPPH radicals readily accept electrons or hydrogens released from the AuNPs and neutralize themselves [Citation39].
Table 4. DPPH radical scavenging activity of cf-sk-AuNPs.
Sanna et al. [Citation40] reported that polyphenol-conjugated AuNPs exerted antioxidant activity. They synthesized AuNPs with three polyphenols (epigallocatechin-3-gallate, resveratrol and fisetin) via a green synthetic strategy. In other reports, the DPPH antioxidant activity was also assessed using AuNPs green synthesized by using the extracts of Angelica pubescens and Citrullus lanatus [Citation41,Citation42]. In both reports, it has been described that antioxidant activity of green-synthesized AuNPs showed dose-dependence. Markus et al. [Citation42] have proposed that the DPPH scavenging activity of AuNPs green-synthesized with Angelica pubescens can be attributed to the antioxidant capacities of the phytochemicals (flavonoids, sesquiterpenes and phenols). However, in the present report, for DPPH radical scavenging activity, the extract was removed by centrifugation to minimize the amount of biomolecules on the surface of the sk-AuNPs. The DPPH radical scavenging activity showed Au concentration-dependence. Thus, a main force for the scavenging radicals is the direct involvement of the AuNPs.
Cytotoxicity on cancer cells
The cytotoxicity of cf-sk-AuNPs on seven human cancer cells was examined using the WST assay (). Remarkably, HeLa (, HT29 () and MDA-MB-231 () cells showed significant cytotoxicity compared with the other four cells. Among these three cells, at 126 μg/mL, the highest cytotoxicity was observed on MDA-MB-231 (cytotoxicity 95%) followed by HeLa (cytotoxicity 68%) and lastly by HT-29 (cytotoxicity 40%). There was no significant cytotoxicity observed in A549, AGS, HepG2 and PANC-1 at all tested concentrations. Therefore, it is noteworthy that the cytotoxicity was dependent on tissue type. Specifically, three cancer tissues, including breast, uterus and colon, showed high cytotoxicity. Accordingly, the cf-sk-AuNPs have great possibilities to be potential anticancer agents for MDA-MB-231, HeLa and HT-29 cells. Sabella et al. have reported that a general mechanism for the intracellular toxicity of metallic nanoparticles is an endocytosis-driven process that results in the release of metal ions in an acidic lysosomal environment [Citation43]. The enhancement of metal ion release induces enzyme depletion and/or inactivation and protein denaturation, which consequently results in the increased levels of reactive oxygen species, apoptosis, DNA and membrane damage [Citation43]. The strong cytotoxicity toward three cancer cells, MDA-MB-231, HeLa and HT-29 cells is possibly due to a selective endocytosis-driven mechanism. The exploration of a detailed mechanism in the selective cytotoxicity will be our future work.
Cell morphology of HeLa cells
To confirm and visualize the cytotoxicity of the cf-sk-AuNPs, the cell morphology of HeLa cells was observed and shown in . Under no treatment with the cf-sk-AuNPs, the cells are normal without any morphological changes (). However, as the concentration of cf-sk-AuNPs was increased (), the cell morphology was distorted compared with the untreated cell. The cytoplasm was distorted to a round shape followed by the cell dying and rupturing. Furthermore, for the cells that were treated with the highest concentration, 126 μg/mL, round cells along with ruptured cells, were clearly observed due to the cytotoxicity of the cf-sk-AuNPs.
Haemocompatibility assays
For the development of nanoparticle delivery systems, a haemolysis experiment that demonstrates the compatibility of nanoparticles with blood constituents is crucial. The haemolysis percentage of the sk-AuNPs is shown in . Haemolysis (0.7%) was observed when the Au concentration was 14.7 μg/mL. When the Au concentration was increased two-fold (29.4 μg/mL), the haemolysis only increased to 1.2%. Based on these results, the sk-AuNPs were reasonably haemocompatible with human blood cells at the tested Au concentrations.
Conclusion
An upcycling strategy has been expanding to recycle natural wastes for producing highly valuable materials. Specifically, this strategy also achieves sustainability initiatives for preserving our global environment. In the present report, skate cartilage waste was efficiently utilized as reducing and stabilizing agents for the synthesis of sk-AuNPs. The extract and Au salts were employed for the synthesis, and no other chemicals were used, which provides an entirely green synthetic route. Spherical sk-AuNPs possessed large negative zeta potentials. Negative charges originated from amino acids and chondroitin sulphates from the extract possibly play a role as stabilizing and capping agents to provide excellent colloidal stability. The size and shape of the sk-AuNPs were retained on the shelf and under cell culture conditions. The most remarkable applications of AuNPs are medical and biomedical applications, specifically, drug delivery. Owing to their high haemocompatibility and excellent colloidal stability, sk-AuNPs are capable of being drug delivery vehicles. Most remarkably, the cytotoxicity toward cancer cells was tissue-specific on HeLa, HT29 and MDA-MB-231. Facile modification of the surface of AuNPs is one of the merits for medical and biomedical applications. The surface functionalization of sk-AuNPs with anticancer agents and/or targeting ligands recognizing these three cancer cells can be applied as promising drug delivery vehicles for the treatment of cancers.
Disclosure statement
No potential conflict of interest was reported by the authors.
Additional information
Funding
References
- Mittal AK, Chisti Y, Banerjee UC. Synthesis of metallic nanoparticles using plant extracts. Biotechnol Adv. 2013;31:346–356.
- Rafique M, Sadaf I, Rafique MS, et al. A review on green synthesis of silver nanoparticles and their applications. Artif Cells Nanomed Biotechnol. 2017;45:1272–1291.
- Park Y. New paradigm shift for the green synthesis of antibacterial silver nanoparticles utilizing plant extracts. Toxicol Res. 2014;30:169–178.
- Akhtar MS, Panwar J, Yun YS. Biogenic synthesis of metallic nanoparticles by plant extracts. ACS Sustainable Chem Eng. 2013;1:591–602.
- Daraee H, Eatemadi A, Abbasi E, et al. Application of gold nanoparticles in biomedical and drug delivery. Artif Cells Nanomed Biotechnol. 2016;44:410–422.
- Abadeer NS, Murphy CJ. Recent progress in cancer thermal therapy using gold nanoparticles. J Phys Chem C. 2016;120:4691–4716.
- Bayford R, Rademacher T, Roitt I, et al. Emerging applications of nanotechnology for diagnosis and therapy of disease: a review. Physiol Meas. 2017;38:R183.
- Poornima V, Alexandar V, Iswariya S, et al. Gold nanoparticle-based nanosystems for the colorimetric detection of Hg2+ ion contamination in the environment. RSC Adv. 2016;6:46711–46722.
- Priyadarshini E, Pradhan N. Gold nanoparticles as efficient sensors in colorimetric detection of toxic metal ions: a review. Sens Actuators B Chem. 2017;238:888–902.
- Adabi M, Naghibzadeh M, Adabi M, et al. Biocompatibility and nanostructured materials: applications in nanomedicine. Artif Cells Nanomed Biotechnol. 2017;45:833–842.
- Li F, Lu J, Kong X, et al. Dynamic nanoparticle assemblies for biomedical applications. Adv Mater. 2017;29:1605897.
- Park Y, Hong Y, Weyers A, et al. Polysaccharides and phytochemicals: a natural reservoir for the green synthesis of gold and silver nanoparticles. IET Nanobiotechnol. 2011;5:69–78.
- Park Y, Noh HJ, Han L, et al. Artemisia capillaris extracts as a green factory for the synthesis of silver nanoparticles with antibacterial activities. J Nanosci Nanotechnol. 2012;12:7087–7095.
- Im AR, Han L, Kim ER, et al. Enhanced antibacterial activities of Leonuri herba extracts containing silver nanoparticles. Phytother Res. 2012;26:1249–1255.
- Jun SH, Kim HS, Koo YK, et al. Root extracts of Polygala tenuifolia for the green synthesis of gold nanoparticles. J Nanosci Nanotechnol. 2014;14:6202–6208.
- Jun SH, Cha SH, Kim J, et al. Crystalline silver nanoparticles by using Polygala tenuifolia root extract as a green reducing agent. J Nanosci Nanotechnol. 2015;15:1567–1574.
- Jun SH, Cha SH, Kim JH, et al. Silver nanoparticles synthesized using Caesalpinia sappan extract as potential novel nanoantibiotics against methicillin-resistant Staphylococcus aureus. J Nanosci Nanotechnol. 2015;15:5543–5552.
- Lee YJ, Park Y. Anisotropic snowman-like silver nanoparticles synthesized by Caesalpinia sappan extract and in vitro antibacterial activity. J Nanosci Nanotechnol. 2018;18:3880–3887.
- Lee YJ, Cha SH, Lee KJ, et al. Plant extract (Bupleurum falcatum) as a green factory for biofabrication of gold nanoparticles. Nat Prod Commun. 2015;10:1593–1596.
- Park JS, Ahn EY, Park Y. Asymmetric dumbbell-shaped silver nanoparticles and spherical gold nanoparticles green-synthesized by mangosteen (Garcinia mangostana) pericarp waste extracts. Int J Nanomed. 2017;12:6895.
- Han L, Kim YS, Cho S, et al. Invertebrate water extracts as biocompatible reducing agents for the green synthesis of gold and silver nanoparticles. Nat Prod Commun. 2013;8:1149–1152.
- Park S, Cha SH, Cho I, et al. Antibacterial nanocarriers of resveratrol with gold and silver nanoparticles. Mater Sci Eng C Mater Biol Appl. 2016;58:1160–1169.
- Kim TY, Cha SH, Cho S, et al. Tannic acid-mediated green synthesis of antibacterial silver nanoparticles. Arch Pharm Res. 2016;39:465–473.
- Kim TY, Park Y. Green synthesis and catalytic activity of gold nanoparticles/graphene oxide nanocomposites prepared by tannic acid. J Nanosci Nanotechnol. 2018;18:2536–2546.
- Lim SH, Park Y. Green Synthesis, Characterization and catalytic activity of gold nanoparticles prepared using rosmarinic acid. J Nanosci Nanotechnol. 2018;18:659–667.
- Seo YS, Ahn EY, Park J, et al. Catalytic reduction of 4-nitrophenol with gold nanoparticles synthesized by caffeic acid. Nanoscale Res Lett. 2017;12:7.
- Noh HJ, Kim HS, Jun SH, et al. Biogenic silver nanoparticles with chlorogenic acid as a bioreducing agent. J Nanosci Nanotechnol. 2013;13:5787–5793.
- Park J, Cha SH, Cho S, et al. Green synthesis of gold and silver nanoparticles using gallic acid: catalytic activity and conversion yield toward the 4-nitrophenol reduction reaction. J Nanopart Res. 2016;18:1–13.
- Choi Y, Choi MJ, Cha SH, et al. Catechin-capped gold nanoparticles: green synthesis, characterization, and catalytic activity toward 4-nitrophenol reduction. Nanoscale Res Lett. 2014;9:103.
- Kim HS, Jun SH, Koo YK, et al. Green synthesis and nanotopography of heparin-reduced gold nanoparticles with enhanced anticoagulant activity. J Nanosci Nanotechnol. 2013;13:2068–2076.
- Im AR, Kim JY, Kim HS, et al. Wound healing and antibacterial activities of chondroitin sulfate- and acharan sulfate-reduced silver nanoparticles. Nanotechnology. 2013;24:395102.
- Cho HJ, Oh J, Choo MK, et al. Chondroitin sulfate-capped gold nanoparticles for the oral delivery of insulin. Int J Biol Macromol. 2014;63:15–20.
- Noh HJ, Im AR, Kim HS, et al. Antibacterial activity and increased freeze-drying stability of sialyllactose-reduced silver nanoparticles using sucrose and trehalose. J Nanosci Nanotechnol. 2012;12:3884–3895.
- Hur YE, Kim S, Kim JH, et al. One-step functionalization of gold and silver nanoparticles by ampicillin. Mater Lett. 2014;129:185–190.
- Hur YE, Park Y. Vancomycin-functionalized gold and silver nanoparticles as an antibacterial nanoplatform against methicillin-resistant Staphylococcus aureus. J Nanosci Nanotechnol. 2016;16:6393–6399.
- Ahn EY, Park Y. Facile Fabrication of gold nanoparticles with ethambutol. J Nanosci Nanotechnol. 2017;17:4851–4857.
- Im AR, Sim JS, Park Y, et al. Isolation and characterization of chondroitin sulfates from the byproducts of marine organisms. Food Sci Biotechnol. 2009;18:872–877.
- Muralidharan N, Shakila RJ, Sukumar D, et al. Skin, bone and muscle collagen extraction from the trash fish, leather jacket (Odonus niger) and their characterization. J Food Sci Technol. 2013;50:1106–1113.
- Shah S, ud Din S, Khan A, et al. Green synthesis and antioxidant study of silver nanoparticles of root extract of Sageretia thea and its role in oxidation protection technology. J Polym Environ. 2018; 26:2323–2332.
- Sanna V, Pala N, Dessí G, et al. Single-step green synthesis and characterization of gold-conjugated polyphenol nanoparticles with antioxidant and biological activities. Int J Nanomed. 2014;9:4935–4951.
- Patra JK, Baek KH. Novel green synthesis of gold nanoparticles using Citrullus lanatus rind and investigation of proteasome inhibitory activity, antibacterial, and antioxidant potential. Int J Nanomed. 2015;10:7253.
- Markus J, Wang D, Kim YJ, et al. Biosynthesis, characterization, and bioactivities evaluation of silver and gold nanoparticles mediated by the roots of chinese herbal Angelica pubescens Maxim. Nanoscale Res Lett. 2017;12:46.
- Sabella S, Carney RP, Brunetti V, et al. A general mechanism for intracellular toxicity of metal-containing nanoparticles. Nanoscale. 2014;6:7052–7061.