Abstract
Delivery of the drugs to the target tissue and reducing their side effects on surrounding tissues is still a significant challenge for pharmaceutical scientists. The aim of this study was to investigate the suitability of PLGA–PEG–PLGA triblock copolymer as a matrix material for a sustained-release system of sodium deoxycholate (NaDC). The copolymer was synthesized by ring-opening polymerization reaction, using microwave irradiation and characterized by different techniques. It was shown that the introduction of NaDC to the PLGA–PEG–PLGA copolymer did not influence its inherent sol–gel transition behaviour, but increased the sol–gel transition. The results showed the appropriate NaDC/polymer interaction and the formation of NaDC/polymer-mixed micelle. The sustained release of NaDC from the copolymer lasted for 2 days. This release can be attributed to the formation of NaDC/polymer-mixed micelles and trapping NaDC in the copolymer matrix. The cytolytic efficacy of NaDC-loaded copolymer and sustained release of NaDC were investigated on human adipocytes. Overall a sustained-release formulation for NaDC can be used to study localized fat dissolution.
Introduction
Obesity has become a worldwide major public health problem. Although some people are doing a lot of exercise and strict diet regimens, but localized fat stays in some parts of their body [Citation1]. Mesotherapy is an efficient drug delivery method to avoid systemic adverse effects. Formulations containing phosphatidylcholine (PC) and sodium deoxycholate (NaDC) have been used in the mesotherapy and NaDC is the active component in this formulation and causes non-specific lysis of cell membranes [Citation2]. NaDC is a type of bile acids, composed of an amphipathic steroid skeleton, with a hydrophilic alpha side, and hydrophobic beta side [Citation3]. Some researchers have sought to use doxycolic acid and ursodeoxycholic acid, as a therapeutic agent with anti-inflammatory, antiviral, anti-cancer, and immune-stimulating properties [Citation4,Citation5]. Subcutaneous injections of NaDC have also shown to reduce size of human lipoma and treat “buffalo hump” in patients infected with human immunodeficiency virus (HIV) [Citation6,Citation7]. Injecting NaDC leads to degrading fat cells via a cytolytic mechanism and skin tightening [Citation8], but may cause necrosis of surrounding fat tissue, such as skin, muscles, nerves, and blood vessels in body [Citation9–11]. The sustained and local release of NaDC in fatty tissues might lead to reduction of aggressiveness, improvement of efficiency, and increase in selectivity of fat cells.
Nowadays, injectable thermogelling hydrogels have been extensively investigated for sustained delivery of a variety of drugs due to their good properties. These hydrogels are in the form of solution at low or room temperature and transform to hydrogel at low critical solution temperature (LCST) [Citation12–16]. Triblock copolymer PLGA–PEG–PLGA is an injectable thermogelling hydrogel. This triblock copolymer forms a regular micelle structure in aqueous medium. The advantage of PLGA–PEG–PLGA consists of the delivery of both hydrophobic and hydrophilic drugs, biodegradability, and biocompatibility. The products of copolymer degradation are lactic and glycolic acid [Citation17–19]. In this work, a sustained-release system for NaDC-based injectable polymer solution of PLGA–PEG–PLGA copolymer was developed to fulfil the localized fat therapy. Due to the interconnections between the triblock copolymers and both ionic and non-ionic surfactants, they can mix together and form mixed micelles which are potentially promising in the pharmaceutical applications [Citation20]. For this purpose, the copolymer was synthesized and characterized, then the properties of the NaDC-loaded PLGA–PEG–PLGA were investigated. Finally, in vitro and drug-release efficacy studies of the copolymer system were conducted.
Experimental
Materials
Glycolide (GA), D, L-lactide (LA), stannous 2-ethylhexanoate, poly (ethylene glycol) (PEG, Mn 1500), NaDC and 3–(4,5-dimethyl-thiazol-2-yl)-2,5-diphenyl tetrazolium bromide (MTT) were purchased from Sigma-Aldrich (USA). Human preadipocyte cells were obtained from the Cell Technology Research Center, Iran. Human breast cancer cells (cell line MDA-MB-231) were obtained from the China Infrastructure of Cell Line Resources, China.
Synthesis of PLGA–PEG–PLGA triblock copolymers using microwave irradiation
The PLGA–PEG–PLGA triblock copolymer [LA:GA =3:1] was synthesized by ring-opening polymerization from LA and GA with PEG1500 as the initiator in the presence of Stan-2-ethylhexanocyte, using microwave irradiation [Citation12]. LA, GA, PEG1500 and stannous 2-ethylhexanoate were loaded into a flask. After sealing the system at 150 °C, the mixture was stirred at 250 rpm. Irradiation was performed at 800 W with a microwave (Milestone MicroSYNTH, Italy) for approximately 5 min. Finally, the product was purified by dissolving in 4 °C distilled water and washing in 80 °C water for three times.
Characterization of PLGA–PEG–PLGA triblock copolymers
The chemical structure and composition of the copolymers were determined by proton nuclear magnetic resonance (1H–NMR) spectrometry (Bruker spectrometer, DMX300, Germany). Spectra were recorded at 300 MHz on a Varian spectrometer at 25 °C with deuterated chloroform (CDCl3) as the solvent. A tetramethylsilane signal was taken as the zero chemical shifts. Number average molecular weight (Mn) and [LA:GA] were determined by integrating the signals pertaining to each monomer such as the peaks from CH and CH3 of LA, CH2 of ethylene glycol and CH2 of GA. Molecular weights and distributions of copolymers were obtained by gel permeation chromatography (GPC) at 20 °C. Tetrahydrofuran was used as the eluent at a flow rate of 1 mL/min. Monodispersed polystyrene was used as a standard to generate the calibration curve for GPC. The results are summarized in . The Tg of copolymer was determined by differential scanning calorimetry (DSC) (PerkinElmer, DSC 8000, USA) in the temperature range of −50 °C to 50 °C with a heating rate of 20 °C/min.
Table 1. Results of 1H–NMR and GPC of PLGA–PEG–PLGA.
Phase diagram of PLGA–PEG–PLGA in water
In order to determine the sol–gel transition of the copolymer aqueous solution, the vial inverting method [Citation15] was used as the temperature increased from 10 to 50 °C, with a temperature interval of 1 °C per step. The different weight concentrations of polymer were prepared in phosphate-buffered saline (PBS) (pH 7.4) at 4 °C. The sol–gel transition was determined by inverting the vial horizontally after keeping the sample at a constant temperature for 2 min to reach the equilibrium. It is noteworthy that the contents of the vials do not flow after inverting once the hydrogel is formed. In order to confirm the result of the inverted test tube, the sol–gel transition temperature was also determined by DSC (PerkinElmer, DSC 8000, USA). Then 10 μL of 22% w/v copolymer solution were inserted into the pan and heated from 4 to 55 °C at a rate of 3 °C/min. Distilled water was used as a blank.
Drug loading
Injection of NaDC high concentrations is associated with more severe and prolonged side effects and only low concentration of NaDC (1% w/v) can be uniformly tolerated by the body. Considering the effect of surfactants on polymer structure, LCST, and the toxic effects of high concentrations of the NaDC, 1% (w/v) of NaDC was selected for loading in the PLGA–PEG–PLGA copolymer.
Rheological studies
The rheological properties of 22% w/v PLGA–PEG–PLGA copolymer solution were studied by dynamic rheological method [Citation14], using a rheometer (Malvern, Kinexus, USA) equipped with parallel plates of 60-mm diameter. The elastic G′, viscous modulus G″ and the complex viscosity η of copolymer as a function of temperature were studied. It is noteworthy that G′ expresses the elastic behaviour or the energy stored in the sample during the deformation, while G″ shows the viscous character or the energy dissipated as heat. Cold samples were dropped on the parallel plates with an initial temperature of 15 °C. The storage modulus G′ and loss modulus G″ were determined along with the temperature increase to 50 °C with a heating rate of 0.5 °C/min and an oscillatory frequency of 10 rad/s.
Micelle size and morphology
The interactions between NaDC and copolymer in water and the size of PLGA–PEG–PLGA copolymer micelles in buffer were determined using a dynamic light scattering (DLS; Malvern, USA). The morphology of the micelles was visualized by a transmission electron microscopy (TEM; HT7700, Hitachi, Japan) at an accelerating voltage of 200 kV. Briefly, the samples were mounted on copper grids coated with a thin carbon film and observed by TEM after air-drying.
X-ray diffraction
Powder X-ray diffraction (XRD) patterns of the pure NaDC, copolymer (22% w/v) and NaDC-loaded copolymer (1% w/v, 22% w/v) were studied using a RINT2000 vertical goniometer (Rigaku Corporation, Japan) with Cu–Kα radiation (=1.54056 Å) within the 2θ range of 3–80 at 40 kV and 100 mA.
In-vitro release
First, 22% w/v PLGA–PEG–PLGA aqueous solution containing 1% w/v NaDC was prepared. Then 0.5 mL of the mixed solution was put into a vial with an inner diameter of 16 mm, and incubated at 37 °C for 10 min to form the NaDC-loaded hydrogels. At desirable time intervals, 3-mL supernatant was withdrawn and replaced with fresh release medium (PBS) of the same volume. The amount of NaDC in the release medium was evaluated by UV spectrophotometer (A590, AOE, Shanghai, China) at a wavelength of 202 nm. The supernatant of the hydrogel was considered as blank. In order to confirm the results of NaDC release, some tests were repeated by a reverse-phase high-performance liquid chromatography (HPLC) system (Knauer, Berlin, Germany) on an analytical Inertsil ODS-3 column (Inertsil, Eindhoven, Netherlands) equipped with a refractive index (RI) detector (Knauer 2300, Germany). A mobile phase composed of acetonitrile: methanol: sodium acetate (20 mM in acetic acid with pH 4.3) in the proportion of 60:05:35 at flow rate of 1 mL/min. NaDC was detected by a RI detector.
Cell culture and cell viability studies
In order to transform the solutions into hydrogels, 0.1 mL of NaDC-loaded copolymer (22% w/v polymer and 1% w/v NaDC) were transferred to transwell (MILLIPORE, 24 Well Millicell, 8.0 µm, Germany) and incubated at 37 °C for 30 min. The transwells were soaked in the 24-well culture plates filled with pre-cultured adipocytes. The plates were incubated in CO2 incubator (Sanyo MCO-20AIC CO2, japan) at 37 °C in a humidified atmosphere consisting of 5% vol CO2. To best evaluate the sustained release of drug from the mixed micelles, copolymer matrix, and efficacy of NaDC in inducing cell death after release from copolymer matrix, MTT assay was fulfilled after 2 and 24 h. Cell viability studies were monitored on adipocyte, and human breast cancer cells (cell line MDA-MB-231).
Results
Synthesis, characterization and thermogellability of copolymer
The PLGA–PEG–PLGA triblock copolymer was synthesized by microwave irradiation method. The copolymer synthesized was characterized via 1H–NMR and GPC. 1H–NMR spectra and GPC chromatogram are shown (Figure S1A and 1B in Supplementary Material). The characteristic copolymer peaks include CH of LA (5.2 ppm), CH3 of LA (1.5 ppm), CH2 of GA (4.8 ppm) and CH2 of ethylene glycol (3.6 ppm and 4.3 ppm). The NMR and GPC results are listed in . The glass transition temperatures (Tg) of synthetized polymer was also determined by DSC. Tg was shown about –1 °C (Figure S1C in Supplementary Material), which indicates that the PLGA–PEG–PLGA system has the lower chain mobility at body temperature [Citation14].
The PLGA–PEG–PLGA aqueous solutions revealed a sol–gel phase transition with increasing temperature as determined by the vial inverting method (). The result exhibited that as the polymer concentration increased from 5 to 22% w/v, the sol-to-gel transition temperature decreased, while the gel-to-precipitation transition temperature increased. Sol-to-gel transition temperature of 22% w/v polymer was ∼29 °C. Thus, gel window of polymer/water system seem to be suitable for biomedical applications in the body temperature. Notably, the transition temperature obtained by DSC was in good agreement with that determined by the inverted test tube method (). The rheological properties of triblock copolymer also were investigated by dynamic rheological measurements (). The elastic G′, viscous modulus G″ and the complex viscosity η of copolymer as a function of temperature were studied. In the low temperature, it was indicated that G″ was larger than G′, and both of them began to increase noticeably. The temperature that the G″ and G′ curves intersected is the sol–gel transition temperature (at ∼28 °C). This temperature was close to the temperature reported by the vial inverting method (29 °C). In the gel state, G′ became over G″. While, in the sol state, complex viscosity η´ was independent of temperature, but it showed a temperature-dependent behaviour during the onset of gelation.
Figure 1. Termogellability of copolymer aqueous solutions. (A) Phase diagrams of PLGA–PEG–PLGA triblock copolymer in PBS (pH 7.4). (B) The sol–gel transition temperature of PLGA–PEG–PLGA (22% w/v) solution obtained by DSC (C) Storage modulus G′, loss modulus G″ and complex viscosity η´ of PLGA–PEG–PLGA (22% w/v) solution in PBS (pH 7.4) as a function of temperature. Heating rates: 0.5 °C/min, oscillatory frequency: 10 rad/s.
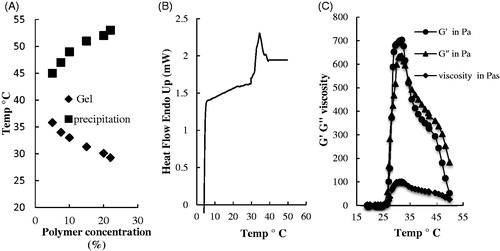
Interactions between NaDC and PLGA–PEG–PLGA triblock copolymer
The interactions between NaDC and copolymer in water were first monitored by DLS. The PLGA–PEG–PLGA triblock copolymer formed micelles in water. Distribution of hydrodynamic diameter of copolymer micelles was examined in water, in the absence and presence of NaDC in a molar ratio of 2 to 1 (copolymer/NaDC) by DLS at 25 °C. The incorporation and interaction of NaDC with copolymer micelles increased the micelle size. DLS measurements revealed that NaDC penetrated into the core–corona region of PLGA–PEG–PLGA micelles and formed mixed micelles of bigger size (). In order to support this observation and monitoring the morphology of the micelles of polymer after loading NaDC, we fulfilled TEM observations. The images showed that the micelles maintained a spherical shape after loading NaDC (). The average size of micelles in the microscopic image was examined by ImageJ software (win32) to be about 32 nm.
Figure 2. Distribution of hydrodynamic diameter of copolymer micelles in water based on the intensity (A) in the absence of NaDC (Z-average= 177) and (B) the presence of NaDC (Z-average= 201) in a molar ratio of 2:1 copolymer/NaDC by DLS at 25 °C. (C) TEM images of the copolymer–NaDC-mixed micelle at 2: 1 molar ratio of copolymer and NaDC, scale bar 100 nm. (D) Effects of NaDC on storage modulus G′ of PLGA–PEG–PLGA (22% w/v) solution in PBS (pH 7.4) at different temperatures. Drug-loading concentration 1% w/v, heating rates: 0.5 °C/min, oscillatory frequency: 10 rad/s. (E) Powder X-ray diffraction patterns of NaDC, pure PLGA–PEG–PLGA copolymer and the NaDC-loaded copolymer. (1) Pure NaDC, (2) pure polymer, and (3) NaDC-loaded copolymer (1% w/v, 22% w/v). (F) Cumulative release of NaDC from PLGA–PEG–PLGA copolymer in PBS (pH 7.4) at 37 °C. The data are represented as the mean ± SD, n = 3. The polymer concentration was 22% w/v and the drug-loading amount was 1% w/v. The lines are used just for the guides of the eyes.
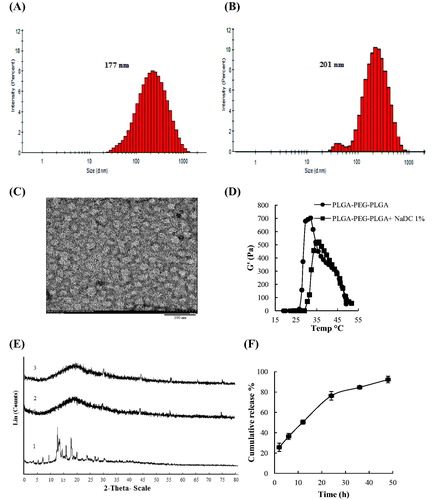
The rheological properties of PLGA–PEG–PLGA copolymer with NaDC were investigated ( and Figure S2 in Supplementary Material). The loading of 1% w/v NaDC into the polymer solution led to an increase on their sol–gel transition temperature (28 °C to 32 °C). Nevertheless, the presence of NaDC reduced G′, G″ and viscosity η of polymer solution. It is noteworthy that reducing G″ with loading drug in this study is advantageous since it might lead to simplicity in injecting. Additionally, G′ and G″ reduction of the NaDC-loaded copolymer could be a sign of decreasing gel strength. It is notable that the decrease of G′ and η are also attributed to the interactions between the copolymer chains and NaDC micelles.
XRD analysis is often used to investigate drug–polymer interactions and the state of a drug in a polymer matrix [Citation16, Citation21]. The drug–polymer interactions result in a change in the drug molecular mobility, ultimately leading to an inhibitory effect on crystallization of drug molecules and an amorphous dispersion in the polymer [Citation22]. The XRD patterns of pure NaDC, copolymer, and NaDC-loaded copolymer (1% w/v, 22% w/v) were reported (). The presence of a broad peak in the XRD patterns for the copolymer approved that it was a semi-crystalline polymer. Crystallization state of NaDC was presented by the numerous sharp peaks in the XRD pattern. While, the board peak of NaDC-loaded copolymer suggests that NaDC is either in an amorphous state or in the molecular dispersion.
In-vitro release of NaDC from copolymer
We investigated the in-vitro release profiles of NaDC from the copolymer system in PBS (pH 7.4) at 37 °C ( and Figure S3 in Supplementary Material). The sustained release of NaDC from the copolymer continued up to 2 days. This result demonstrated that the copolymer matrix was capable of providing a prolonged release of NaDC. This finding can be attributed to the physical interactions between NaDC molecules and the hydrophobic cores of copolymer micelles, through generating mixed micelles, which effectively delayed the diffusion of drug. The diffusion-controlled manner in the initial stage and the combined mechanism of diffusion and degradation in the later stage are two types of mechanism which might be involved in the drug release from the hydrogels [Citation14]. The release profiles of NaDC from the PLGA–PEG–PLGA copolymer are in agreement with the results in the literature.
Cell viability
The cytotoxicity of NaDC on cultured human adipocyte was shown to be dose and time dependent. Cell viability was greatly reduced after 2 h (Figure S4 in Supplementary Material). In order to evaluate efficacy of sustained release of drug from copolymer matrix, MTT assay was fulfilled after 2 and 24 h (. For the 2-h test, in comparison with non-treated control, viability of cells incubated with NaDC was ∼10%, while the copolymer without NaDC was 100%, and copolymer formulated with NaDC was over 90% (). This test indicated the sustained release of NaDC from the copolymer matrix. To assess the cytotoxic effects of NaDC after the release from copolymer and biocompatibility of the copolymer, the adipocytes were exposed to the same samples for 24 h (). The cell viability of copolymer was determined above 90%, indicating good biocompatibility of the copolymer. For the copolymer formulated with NaDC, the cell viability was about 10%, showing cytotoxic effect of released NaDC from copolymer. The same results of cell viability were obtained for human breast cancer cell line MDA-MB-231 (Figure S5 in Supplementary Material).
Discussion
A large number of clinical studies have shown that formulations including PC and NaDC can reduce local fat in different parts of the body [Citation23, Citation24]. Later, it was found that NaDC is the main substance and PC alone cannot cause cell lysis. NaDC as surfactant causes the destruction of cell membranes [Citation25, Citation26]. It is noteworthy that the side effects of NaDC are dose-dependent and limited to the injection site [Citation10]. Moreover, the NaDC aggressiveness and the sudden destruction of fat cells cause the severe pain in injection site in the early days. The cell culture studies also confirmed that NaDC destroys different types of cells such as fat, fibroblast, endothelial and muscle cells [Citation4]. In physiological conditions, NaDC is inactivated and transmitted to the gastrointestinal tract by binding to proteins, including albumin. As fat tissue contains less protein in comparison with most body tissues, fat tissue is expected to be more sensitive to NaDC [Citation27,Citation28]. However, unspecific cytotoxic effects of NaDC are prone to affect not only the fat cells but also the muscles, skin and blood vessels. Thereby, reducing its side effects on the surrounding tissues is necessary. The sustained release of NaDC in the target tissue, and reducing the aggressiveness of the drug can greatly reduce the concerns about its potential application.
Injectable thermosensitive hydrogels have been suggested as potential carriers for sustained delivery of various drugs such as proteins, polypeptides and small-molecule drugs [Citation29, Citation30]. In the present study, triblock copolymer PLGA–PEG–PLGA was synthesized due to its solubility, biodegradability and optimal safety in pharmaceutical applications. The LCST of PLGA–PEG–PLGA is close to the body temperature. The PLGA–PEG–PLGA copolymer contains a sequence of hydrophobic–hydrophilic–hydrophobic units that form a regular micelle structure in aqueous medium. This micelle forms the core/shell structure. At lower temperatures than LCST, some of the micelles are interconnected. As the temperature increases to LCST, the PLGA hydrophobicity increases and a network of interconnected micelles is formed. Then the gelation occurs and the drug compound is trapped [Citation31, Citation32]. Herein, the formation of copolymer micelles was also verified by DLS and TEM studies, and the size of the copolymer micelles was shown to be increased by the addition of NaDC. The interaction between the different copolymer and surfactants has been studied. It has been reported that NaDC with Pluronic P-123 micelles and sodium dodecyl sulphate with Pluronic F127 micelles interacted together and formed mixed micelles [Citation20]. Castro et al. reported such changes in the size of diblock copolymer micelles with SDS [Citation33]. Ghahremankhani et al. also reported physical interactions between SDS and the hydrophobic core of PLGA–PEG–PLGA copolymer micelles [Citation34]. The mixed micelles are potentially of interest in the pharmaceutical field. Bayati et al. investigated the utilization of block copolymer micelles as carriers of bile salts to treat bile acid diseases as well as hypercholesterolemia [Citation35]. Considering NaDC as an amphiphilic molecule, we further speculated that the hydrophobic side of NaDC interacts with the PLGA hydrophobic chains, forming mixed micelles. Furthermore, our study indicated the disappearance of the diffraction peak of NaDC in XRD pattern of NaDC-loaded copolymer as an indication of amorphous form of NaDC in copolymer matrix, which confirms the complete entrapment of the drug in the polymeric micelles () [Citation22]. Meanwhile, the introduction of NaDC resulted in changes of the LCST and the rheological properties of the polymer (). Notably, the copolymer-NaDC system had a higher LCST and less gel strength than the pure copolymer, since, the LCST of the polymers depends on the chemical compositions of both the polymer and the aqueous medium. Many studies have reported that the incorporation of surfactants results in the LCST increase [Citation36, Citation37]. This behaviour is due to the solubilization of the polymer in water by the amphiphilic structure of the surfactants. The efficiency of the polymer solubilization by surfactants is different from one to the other. The effect of a sulphate group of SDS has been shown to be much more effective than a carboxylate group of sodium cholate on polymer solubilization [Citation38]. It is noteworthy that sodium cholate is less efficient in the solubilization of the polymers due to the rigidity and bulkiness of its structure. In this study, a sustained-release behaviour of NaDC from the PLGA–PEG–PLGA copolymer was observed. This behaviour was attributed to the interactions between NaDC molecules and the copolymer micelles, formation of the mixed micelles, and trapping NaDC in the copolymer matrix which effectively delayed the drug release. To assess whether the observed cytotoxicity of NaDC may change after releasing from the copolymer, cell culture studies were performed on human adipocyte using transwells. These studies indicated a sustained drug release for NaDC, the preservation of NaDC toxicity after releasing from the PLGA–PEG–PLGA, and lack of polymer toxic effect (biocompatibility) on adipocytes. It seems that this sustained release of NaDC has been able to reduce drug aggressiveness. This studied also demonstrated cytolytic efficacy of NaDC-loaded copolymer on human breast cancer cell. This study proposes the potential of NaDC-loaded PLGA–PEG–PLGA for the cancer treatment studies.
Conclusions
In this study, a sustained-release formulation for NaDC was reported for localized fat dissolution. This formulation was composed of a thermosensitive, injectable, and biodegradable PLGA–PEG–PLGA copolymer as carrier. Thus, a sustained release of NaDC from the copolymer was achieved for 2 days, while the NaDC alone causes rapid cell destruction due to its high aggressiveness. This aggressiveness can cause severe and painful necrosis of adipose tissue and surrounding tissues. This result was attributed to the appropriate interaction between NaDC molecules and the copolymer micelles. Furthermore, the formation of NaDC/polymer-mixed micelles and trapping NaDC in the copolymer matrix plays a key role. This delivery system of NaDC can be further investigated in other fields such as improving targeting of the fat tissue, reducing the aggressiveness and the impact of NaDC on the surrounding tissue, increasing NaDC efficiency on fat tissue in the body and utilization of this system for greater adipose tissues like lipoma and “buffalo hump”. In addition, NaDC has other pharmaceutical applications, as a result, this developed drug delivery system might be of interest for other diseases in the future.
supplemental_file.docx
Download MS Word (2.7 MB)Disclosure statement
No potential conflict of interest was reported by the authors.
Additional information
Funding
References
- El Kamshoushy A, Abel MR, El MN. Evaluation of the efficacy of injection lipolysis using phosphatidylcholine/deoxycholate versus deoxycholate alone in treatment of localized fat deposits. J Clin Exp Dermatol Res 2012;3:2.
- Rotunda AM, Suzuki H, Moy RL, et al. Detergent effects of sodium deoxycholate are a major feature of an injectable phosphatidylcholine formulation used for localized fat dissolution. Dermatol Surg. 2004; 30:1001–1008.
- Tamminen J, Kolehmainen E. Bile acids as building blocks of supramolecular hosts. Molecules 2001;6:21–46.
- Hwang SR, Kim IJ, Park JW. Formulations of deoxycholic for therapy: a patent review (2011 - 2014)). Expert Opin Ther Pat. 2015;25:1423–1440.
- Salvador JA, Carvalho JF, Neves MA, et al. Anticancer steroids: linking natural and semi-synthetic compounds. Nat Prod Rep. 2013;30:324–374.
- Rotunda AM, Ablon G, Kolodney MS. Lipomas treated with subcutaneous deoxycholate injections. J Am Acad Dermatol. 2005;53:973–978.
- Nanda S. Treatment of lipoma by injection lipolysis. J Cutan Aesthet Surg. 2011;4:135
- Cinti S, Mitchell G, Barbatelli G, et al. Adipocyte death defines macrophage localization and function in adipose tissue of obese mice and humans. J Lipid Res. 2005;46:2347–2355.
- Klein SM, Schreml S, Nerlich M, et al. In vitro studies investigating the effect of subcutaneous phosphatidylcholine injections in the 3T3-L1 adipocyte model: lipolysis or lipid dissolution? Plast Reconstr Surg. 2009; 124:419–427.
- Gupta A, Lobocki C, Singh S, et al. Actions and comparative efficacy of phosphatidylcholine formulation and isolated sodium deoxycholate for different cell types. Aesth Plast Surg. 2009;33:346–352.
- Yagima Odo ME, Cuce LC, ODO L, et al. Action of sodium deoxycholate on subcutaneous human tissue: local and systemic effects. Dermatol. Surg. 2007;33:178–189.
- Khodaverdi E, Tekie FS, Mohajeri SA, et al. Preparation and investigation of sustained drug delivery systems using an injectable, thermosensitive, in situ forming hydrogel composed of PLGA–PEG–PLGA. AAPS PharmSciTech. 2012;13:590–600.
- Xing Y, Chen H, Li S, et al. In vitro and in vivo investigation of a novel two-phase delivery system of 2-methoxyestradiol liposomes hydrogel. J. Liposome Res. 2014;24:10–16.
- Chen Y, Li Y, Shen W, et al. Controlled release of liraglutide using thermogelling polymers in treatment of diabetes. Sci Rep. 2016;6:31593.
- Ci T, Chen L, Yu L, et al. Tumor regression achieved by encapsulating a moderately soluble drug into a polymeric thermogel. Sci Rep. 2015; 4:5473.
- Gao Y, Ren F, Ding B, et al. A thermo-sensitive PLGA-PEG-PLGA hydrogel for sustained release of docetaxel. J Drug Target. 2011;19:516–527.
- Nguyen MK, Lee DS. Injectable biodegradable hydrogels. Macromol Biosci. 2010;10:563–579.
- Ebrahimi E, Akbarzadeh A, Abbasi E, et al. Novel drug delivery system based on doxorubicin-encapsulated magnetic nanoparticles modified with PLGA-PEG1000 copolymer. Artificial Cells, Nanomedicine, and Biotechnology. 2016; 244:290–297.
- Tabatabaei Mirakabad FS, Akbarzadeh A, Milani M, et al. A comparison between the cytotoxic effects of pure curcumin and curcumin-loaded PLGA-PEG nanoparticles on the MCF-7 human breast cancer cell line. Artif Cells Nanomed Biotechnol. 2016;44:423–430.
- Roy A, Kundu N, Banik D, et al. How does bile salt penetration affect the self-assembled architecture of pluronic P123 micelles?–light scattering and spectroscopic investigations. Phys Chem Chem Phys. 2015;17:19977–19990.
- Liu J, Xiao Y, Allen C. Polymer-drug compatibility: a guide to the development of delivery systems for the anticancer agent, ellipticine. J Pharm Sci. 2004;93:132–143.
- Yoshioka M, Hancock BC, Zografi G. Inhibition of indomethacin crystallization in poly(vinylpyrrolidone) coprecipitates. J Pharm Sci. 1995;84:983–986.
- Atiyeh BS, Ibrahim AE, Dibo SA. Cosmetic mesotherapy: between scientific evidence, science fiction, and lucrative business. Aesth Plast Surg. 2008;32:842–849.
- Sivagnanam G. Mesotherapy – the French connection. J Pharmacol Pharmacother. 2010;1:4
- Schuller‐Petrovic SA, Wölkart G, Hoefler G, et al. Tissue‐toxic effects of phosphatidylcholine/deoxycholate after subcutaneous injection for fat dissolution in rats and a human volunteer. Dermatol Surg. 2008;34:529–543.
- Palumbo P, Melchiorre E, La Torre C, et al. Effects of phosphatidylcholine and sodium deoxycholate on human primary adipocytes and fresh human adipose tissue. Int J Immunopathol Pharmacol. 2010;23:481–489.
- Rzany B, Griffiths T, Walker P, et al. Reduction of unwanted submental fat with ATX‐101 (deoxycholic acid), an adipocytolytic injectable treatment: results from a phase III, randomized, placebo‐controlled study. Br J Dermatol. 2014;170:445–453.
- Thuangtong R, Bentow JJ, Knopp K, et al. Tissue‐selective effects of injected deoxycholate. Dermatol. Surg 2010;36:899–908.
- Zentner GM, Rathi R, Shih C, et al. Biodegradable block copolymers for delivery of proteins and water-insoluble drugs. J Control Release. 2001;72:203–215.
- Chen L, Li XQ, Cao LP, et al. An injectable hydrogel with or without drugs for prevention of epidural scar adhesion after laminectomy in rats. Chin J Polym Sci. 2016; 34:147–163.
- Gong C, Qi T, Wei X, et al. Thermosensitive polymeric hydrogels as drug delivery systems. Curr Med Chem. 2013; 20:79–94.
- Jeong B, Bae YH, Kim SW. Drug release from biodegradable injectable thermosensitive hydrogel of PEG–PLGA–PEG triblock copolymers. J. Control. Release. 2000;63:155–163.
- Castro E, Taboada P, Barbosa S, et al. Size control of styrene oxide − ethylene oxide diblock copolymer aggregates with classical surfactants: DLS, TEM, and ITC Study. Biomacromolecules 2005;6:1438–1447.
- Ghahremankhani AA, Dorkoosh F, Dinarvand R. PLGA-PEG-PLGA tri-block copolymers as in situ gel-forming peptide delivery system: effect of formulation properties on peptide release. Pharm. Dev. Technol. 2008;13:49–55.
- Bayati S, Galantini L, Knudsen KD, et al. Effects of bile salt sodium glycodeoxycholate on the self-assembly of PEO–PPO–PEO triblock copolymer P123 in aqueous solution. Langmuir 2015;31:13519–13527.
- Schild HG, Tirrell DA. Microcalorimetric detection of lower critical solution temperatures in aqueous polymer solutions. J Phys Chem. 1990;94:4352–4356.
- Schild HG, Tirrell DA. Interaction of poly (N-isopropylacrylamide) with sodium n-alkyl sulfates in aqueous solution. Langmuir 1991;7:665–671.
- Benrebouh A, Avoce D, Zhu XX. Thermo-and pH-sensitive polymers containing cholic acid derivatives. Polymer 2001;42:4031–4038.