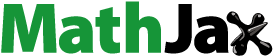
Abstract
Silver nanoparticles have a wide range of research, industrial and biomedical applications that make it essential to develop a low cost and eco-friendly approach with scaling up potential. Green synthesis of nanoparticles through bio-reactions leads to a reduction of silver ions to particles could be an acceptable selection using no additional reducing chemicals. Moreover, the simplicity of scale-up processes of the method makes it more efficient than chemical and physical synthesis methods. In this study, Datura stramonium leaf extract and sodium citrate were used as biological and chemical reducing and stabilizing agents to make silver nanoparticles. The main goal is to comprise properties and evaluate antibacterial activity of nanoparticles synthesized through two approaches. Size and morphology compared between the two types of the synthesized nanoparticle by UV-Visible spectroscopy, DLS, AFM, TEM and their antibacterial effects were evaluated through growth inhibition MIC and MBC methods. The results showed narrow size range, spherical shape, high anti-oxidant, antibacterial and DNA cleavage activities of green synthesized silver nanoparticles comparing to less average size, wider range of nanoparticle size, no anti-oxidant activity and less antibacterial and DNA cleavage activities of chemically synthesized nanoparticles. The green synthesized silver nanoparticles had more desirable characteristics and biological activities compared to chemically synthesized nanoparticles. For instance, the green nanoparticles showed narrow size range, spherical shape, high anti-oxidant, antibacterial and DNA cleavage activities versus the chemically synthesized which had less average size, higher range of nanoparticles size, no anti-oxidant activity and less antibacterial and DNA cleavage activities.
Introduction
Metallic nanoparticles are classified into most useful products applying as adsorbents, catalysts, reinforcers, drug carriers and nano-fertilizers in the various area of science and technologies including healthcare, electronics, new medicine, cosmetics and agriculture [Citation1–5]. Among this nanoscale category, silver nanoparticles (AgNPs) have proven their potential to apply in research and development centres resulting from some unique physical, chemical and biomedical properties [Citation6]. Especially nanoscale size of the particles improved their antibacterial activity, through specific effect such as adsorption at bacterial surfaces [Citation7–9]. There are different strategies for AgNP production such as physical, chemical and green synthesis methods [Citation10–12]. The main process in chemical and green synthesis methods are similar in which silver ions reduce to AgNPs in the presence of reducing and stabilizing agents. The agents are responsible for coat and maintain particles in nanoscale sizes and anisotropic shapes. Decreasing environmental pollution due to use of no chemical reducing agent at green synthesis methods makes them attractive for researchers [Citation13]. There are various ways that nanoparticles influence human body and cause toxicity. For example, dermal contact, oral administration, inhalation and injection in the blood circulation of the silver nanoparticles could induce the changes of cell shape, reduce cell viability, increase lactate dehydrogenase (LDH) release and finally result in cell apoptosis and necrosis. Therefore, the use of the nanoparticle could be restricted in biological applications due to the toxicities [Citation14–17]. However, surface coating of nanoparticles by non-toxic organic compounds could make them safe for biomedical and healthcare purposes [Citation18]. Also, it has been confirmed that useful effects of phytochemicals having reducing/stabilizing properties could be exposed at the surface of nanoparticles by a deposition mechanism in synthesis reaction [Citation19]. Low-cost, energy-efficient, nontoxic and environmental friendly metallic nanoparticles have been numerously synthesized by use of bacteria, yeast, fungi, and plants [Citation20–23]. Due to the simplicity of growing plants, using them for nanoparticles synthesis can be advantageous over other biological methods [Citation22]. Also, plant-mediated approaches can be easily scaled up for large-scale synthesis of nanoparticles. Plants act as bioreactors and the phytochemicals such as sugars, terpenoids, polyphenols, alkaloids, phenolic acids and proteins play an important role in the reduction of metal ions into nanoparticles and in supporting their subsequent stability [Citation24]. Moreover, a phytochemical coating of metal nanoparticles can make the nanoparticles more stable to maintain particles at nano-scale range [Citation25]. It has also been reported that the concentration of silver salt, pH, temperature and reaction time are effective parameters in size, stability, shape and amount of produced nanoparticle [Citation26]. As a plant reducing/stabilizing agent, leaf extract of Datura species from Solanaceae family containing phytochemicals such as flavonoids, carrying OH groups in their structure, could have a reducing potential for nanoparticle synthesis [Citation27]. Although extracts of various species of Datura genus are reported as reducing agents [Citation28,Citation29], there is a little effort for nanoparticle synthesis and analysing its properties by use of Datura stramonium. The plant is a well-known medicinal source including alkaloids, glycosides and flavonoids, and also has desirable reductant activity [Citation30–32].The good antioxidant activity of the aqueous plant extract is reported previously [Citation33]. Also, the antibacterial effects of D. stramonium have repeatedly been shown [Citation34]. Due to the antibacterial effects of the plant extract and high aspect ratio and penetration capability of nanoparticles, an additional effect of corresponding phytochemicals by deposition on AgNPs could induce more antibacterial effects. On the other hand, one of the basic mechanisms for the antibacterial effect of AgNPs is through reactive oxygen species (ROS) production process [Citation35]. This process is induced by both AgNPs and silver ions, inside the cells, by their exposure groups via a Trojan-horse-type mechanism. Intracellular release of silver ions from nanoparticles starts three main pathways by interacting with respiratory chain proteins on the membrane, interrupting reduction of intracellular O2 and finally inducing ROS production [Citation36]. In the current study, a green method using D. stramonium aqueous leaf extract was used for the production of AgNPs. Ions in silver nitrate solution were reduced and stabilized by use of the extract leading to AgNP synthesis in the absence of any chemical reducing/stabilizing agent. Also, chemical synthesis of AgNP performed by use of sodium citrate as a powerful reducing/stabilizing agent providing monodisperse nanoparticles [Citation37]. The green and chemically synthesized nanoparticles were precisely characterized. Products of the two approaches were compared in size by dynamic light scattering (DLS) and transmission electron microscopy (TEM) methods. The X-ray diffraction (XRD) method was used to reveal the silver content of nanoparticles. Fourier-transform infrared spectroscopy (FTIR) analyses were applied to show the phytochemicals deposition on the nanoparticles. Finally, antibacterial properties were demonstrated by good diffusion and minimum inhibitory concentration (MIC) and minimum bactericidal concentration (MBC) methods.
Materials and methods
Preparation of the plant extract
The D. stramonium seeds were purchased from Pakan bazr co (Isfahan, Iran) and cultured in separated pots at greenhouse of University of Isfahan. Harvesting the leaves was done after three months when the grown plants were in the flowering stage having the maximum amount of phytochemicals [Citation38]. The leaves were cut and washed three times by double distilled water and dried completely at room temperature. Two grams of powdered dry leaves were mixed by 100 ml double distilled water, heated for 20 min at 80 °C and filtered through Whatman No.1 filter paper. The extract solution was dehydrated and scraped to obtain a dry extract. One gram of the dried extract was dissolved in 99 ml of double distilled water and pH was adjusted to make a one wt % extract solution. Then, the solution was heated at 50 °C for 10 min under vigorous stirring to complete dissolving of the dried extract.
Preparation of the chemical reducing solution
One gram of sodium citrate salt was dissolved in 99 ml double distilled water under vigorous stirring at 50 °C for 10 min to make a one wt % sodium citrate solution.
Synthesis of silver nanoparticles
The AgNPs synthesized through both green and chemical synthesis methods differ in reducing/stabilizing agents. Different parameters including the concentration of silver nitrate and the plant extract, temperature, pH and time of the reaction were systematically investigated to optimize the synthesis of stable colloidal AgNPs with controlled shape and size. Different reaction conditions, applied for the synthesis of AgNPs by D. stramonium extracts or sodium citrate solution are shown in . All the reactions were performed in the final volume of 50 ml in 50 ml flasks as follows: appropriate concentrations of silver nitrate solution (according to ) were mixed with 1 ml of D. Stramonium 1% plant extract or 1% sodium citrate solution and suitable volumes of double distilled water at desired temperature. The reaction was performed at appropriate times under vigorous stirring. In green synthesis method, silver nitrate 1 mM used as silver ion solution and the plant extract was added with a portion of 1 ml extract to 50 ml silver nitrate at 80 °C under vigorous stirring. Chemical synthesis was also performed by use of the same silver nitrate solution, while sodium citrate was added to reduce silver ions to AgNPs at 80 °C under vigorous stirring. After 120 min both reactions were removed from the heater and centrifuged/washed three times to isolate nanoparticles. Also, to improve the long-term stability of AgNPs, both types of nanoparticles were freeze dried completely using Laboratory freeze dryer (VaCo 5, Germany).
Table 1. Synthetic conditions for the preparation of stable aqueous colloidal solutions of silver nanoparticles by using D. stramonium extracts.
Characterization of synthesized silver nanoparticles
Nanoparticle synthesis process was monitored through UV-vis (model Biowave II, UK) for both green and chemical synthesis methods from initial reaction time over 120 min with a time interval of 10 min. Differential light scattering (DLS) analysis was done to compare size distribution diagrams of green and chemical synthesized nanoparticles by a nanoparticle size analyzer (VASCOTM, Cordouan-tech, France). Before insertion of the sample in the machine, it was sonicated for 15 min to obtain nanoparticle solution with minimum agglomeration. Stability evaluation was done through zeta potential analyzing of the nanoparticles by zeta sizer (Malvern, zen3600, England). Deposition of phytochemicals on green synthesized nanoparticle surfaces were analyzed by placing separated, freeze-dried nanoparticles in an FTIR spectrometer (FT-IR Spectrometer 6300, Jasco, Japan). Morphological characterizations of two resulted types of nanoparticles were also performed through atomic force microscopy (AFM, DualScope™ DME equipped, Germany). Samples were dropped on a glass slide, dried quickly to avoid particle aggregation during water evaporation and finally placed under the tip to scan. The X-ray diffractometer (D8 Advanced X-ray diffractometer, Bruker, Germany) using a wavelength at 1.540598°A (Cu Kα) was used to confirm the silver content of synthesized nanoparticles.
Antioxidant activity assay
The antioxidant activity of the synthesized nanoparticles was measured by the free radical scavenging activity by the DPPH (1,1-diphenyl 2-picrylhydrazyl) method (41–43). The stock solution of DPPH was prepared by dissolving 3.9432 mg DPPH in 100 ml of methanol (0.1 mM) and stored at 4 °C until use. 2 ml of DPPH solution was mixed with 1 ml of different concentrations (20–100 mg ml_1) of the synthesized nanoparticles. Ascorbic acid (100 mg ml−1) was used as the reference standard. A mixture of 1 ml distilled water and 2 ml DPPH solution was used as the control. The reaction mixture was added and incubated at room temperature in the dark for 30 min. The absorbance was recorded spectrophotometrically at 517 nm. The antioxidant activity of the synthesized nanoparticles was estimated based on the percentage of DPPH radical scavenged according to the following equation:
Antibacterial assay
Well diffusion test:
The in vitro antibacterial activity was evaluated by using agar well diffusion assay with Nutrient Agar (NA) as growth media and determination of zone of inhibition measurement in millimetres [Citation39]. Bacterial suspensions of Escherichia coli (ATCC 25922) and Staphylococcus aureus (ATCC 33591) were prepared at a concentration of 0.5 McFarland Scale. Agar plates were inoculated, and 35 μl of the plant extract, silver nitrate, green and chemically synthesized AgNPs solutions containing equal concentrations of 1000 mg ml−1 were added separately into four wells provided in the agar medium. Then the plates were incubated at 37 °C for 24 h. The diameter of the bacterial growth inhibition zone denoted the antibacterial activity.
Minimum inhibitory concentration (MIC) assay:
The antibacterial activity of the NPs suspension was determined by measurement of their minimum inhibitory concentrations (MICs) using the standard micro dilution method [Citation40]. The tested bacteria were E. coli and S. aureus. The MICs were determined using 96-well microtiter plates. A suspension of 0.5 McFarland Scale of bacteria in nutrient broth was prepared. The antibacterial solutions were made using serial two-fold (1:2) dilutions of AgNPs in eight concentrations ranging from 10.24 mg ml−1 to 0.08 mg ml−1 and incubated with the bacterial suspensions at 37 °C for 24 h. The AgNPs-free broth media were used as a negative control. The microbial growth was indexed by measuring the optical density (OD = 600).
Minimum bactericidal concentration (MBC) assay:
The minimum bactericidal concentration (MBC) endpoint is defined as the lowest concentration of antimicrobial agent that kills 99.9% of the initial bacterial population. After the MIC determination of the Ag-NPs, aliquots of 15 μl from all tubes which showed no visible bacterial growth were dropped in agar plates and incubated for 24 h at 37 °C. The MBC was observed for presence or absence of bacterial growth in agar plates [Citation41].
DNA cleavage
The DNA cleavage study of the synthesized nanoparticles was determined by agarose gel electrophoresis using E. coli (ATCC 25922) extracted genomic DNA as a target. Two types of nanoparticles (100 mM) were mixed with the target plasmid (1:1). The mixture was then incubated at 37 °C for 2 h. After incubation, the DNA and the compound mixtures were loaded in wells, and electrophoresis process started at a voltage of 70 V for 30 min. Untreated genomic DNA was used as a negative control [Citation42].
Results
Optical analysis of silver nanoparticles:
After optimizing silver nitrate concentration, temperature and reaction time applied on reaction mixtures to make AgNPs, it was confirmed that optimum condition for the reaction was 2 mM silver nitrate at 80 °C for 120 min. In the green synthesis method, a colour change from light yellow to dark red was considered as an evidence of the nanoparticle synthesis resulted from their surface properties. In the case of UV–visible analysis during synthesis, maximum wavelength absorption was 425 nm that is specific for surface plasmon resonance (SPR) of AgNPs and graphs were symmetrical at this point. The reaction showed an increase in absorbance peak intensity from 0.0 at the moment of starting reaction (0 min) to 1.25 after 120 min demonstrating reduction of silver ions to nanoparticles. Also, there was no significant red shift in the graph during synthesis, a sign of size increasing of the nanoparticles. The same setting was applied for chemical synthesis method. In the chemical synthesis of AgNPs, the colour of the solution changed from colourless (initial moment of the reaction) to grey (after 2 h). Spectroscopic detection showed an increase in absorbance during synthesis reaction from 0.0 at the first time of reaction to 0.34 after 2 h at a wavelength of 425 nm corresponding to the Plasmon absorbance of the AgNPs [Citation43]. However, as the reaction proceeds (from 0 to 2h) a slight trend towards longer wavelengths (redshift) was observed in the graph indicating particle size increasing [Citation44].
Silver nanoparticle characterization:
DLS and zeta potential characterization:
The physicochemical properties such as size distribution and stability index were analyzed by DLS and Zeta potential analyzing methods (). The size distribution of chem-AgNPs was found to be monomodal, with an average particle size of 14.47 nm. Also, a monomodal size distribution was found for green-AgNPs produced by D. stramonium with an average particle size of 20.43 nm. However, a narrower size distribution range was observed in the case of green- AgNPs between 13–60 nm in comparison with chem-AgNPs, ones between 9.5–63 nm. The 15.2 mV and 17.8 mV values were obtained from zeta potential analysis for green and chemically synthesized nanoparticles respectively. After two months, zeta potential was measured again and 0.740 mV and 0.00483 mV values were obtained for green and chemically synthesized nanoparticles respectively.
FTIR analysis:
The FTIR analysis was performed to confirm phytochemical coating during synthesis process of green-AgNPs. As indicated in , the FTIR spectrum of green-AgNPs presented absorption peaks at 3315 cm−1, 2920 cm−1, 1637 cm−1, 1590 cm−1, 1387 cm−1 and 1058 cm−1 are related to O–H, C–H, Alkene C = C, Aromatic C = C, Bending C–H, C–O respectively.
TEM analysis:
More morphological analysis of green synthesized AgNPs presented through TEM images, and spherical, isotropic nanoparticles were clearly observable (). The TEM images show that there is a layer of nano-scale particles, which are less than 100 nm on the surface.
XRD analysis:
The Ag content of the synthesized nanoparticles was confirmed by XRD spectra for both green and chemically synthesized nanoparticles (). Many distinct diffraction peaks at approximately 38.14°, 46.9°, 64.25°, 77.32° and 81.56° were assigned to reflections from the 2θ region for chem-AgNPs spectra.
AFM analysis:
Morphological characterization of the green-AgNPs and chem-AgNPs was analyzed by AFM method. The results demonstrated spherical crystals for green-AgNPs and rectangular and spherical shapes for chem-AgNPs. Also, an aggregation of nanoparticle was observable resulted from both reaction methods ().
Antioxidant activity analysis:
An antioxidant stops oxidation by neutralizing the free radicals produced. To neutralize the free radicals, the antioxidant itself undergoes oxidation. The free radical scavenging activity of the extract demonstrates its potential as a reducing agent for nanoparticle synthesis from their salts. In this study, the DPPH radical scavenging activity of green-AgNPs and chem-AgNPs was tested and compared with the plant extract (). The antioxidant activity of the green-AgNPs was evaluated using DPPH scavenging assay and the results exhibited more effective inhibition activity for green- AgNPs comparing with chem-AgNPs. The chem-AgNPs exhibited no free radical scavenging potent.
Antibacterial assays
Well diffusion assay:
Antibacterial studies were conducted against Staphylococcus aureus and Escherichia coli as models for Gram-positive and Gram-negative bacteria. The results of well diffusion method revealed that green-AgNP and chem-AgNP showed antibacterial activity with varying magnitudes, depending on the type of nanoparticles (). The largest zone in comparison with chem-AgNP, silver nitrate and the plant extract with diameters of 1 mm, 4 mm and 1 mm respectively. The green-AgNPs and silver nitrate also had the largest inhibition zone with diameters of 4 mm against S. aureus in comparison with an identical inhibition zone with diameters of 1 mm for chem-AgNP and the plant extract. Tetracycline was used as a positive control for both types of the bacteria.
MIC assay:
Evaluation of the bacteriostatic activity of AgNPs was carried out by determining the MIC of the AgNPs for both E. coli and S. aureus. The results of bacteriostatic analysis of AgNPs are shown in . When bacterial growth at different concentrations of green-AgNPs was assessed after 24 h, the maximum MIC was observed for a concentration of 5.12 mg/ml in E. coli and 10.24 mg/ml in S. aureus. The chem-AgNPs showed maximum MIC for an identical concentration of 10.24 mg/ml in both bacteria.
MBC assay:
Quantitative antibacterial concentrations were evaluated by determining the MBC. The MBCs 2.56 μg/mL and 1.28 μg/mL were achieved by green-AgNPs for E. coli and S. aureus respectively. In the case of chem-AgNP growth of the microorganisms was observed in any concentration albeit a slight inhibition effect in the highest concentration of the nanoparticles ().
Figure 11. MBC of AgNPs against E. coli (a: green-AgNPs and b: chemical-AgNPs) and S. aureus (c: green-AgNPs and d: chemical-AgNPs) determined through sampling from different concentrations (A: 1024 μg ml−1, B: 512 μg ml−1, C: 256 μg ml−1, C: 128 μg ml−1, D: 64 μg ml−1, E: 32 μg ml−1, F: 16 μg ml−1, H: 8 μg ml−1) in MIC test.

DNA cleavage activity
To explore the ability of nanoparticles to cleave DNA, genomic DNA was used. When DNA cleavage was analyzed by electrophoresis, the smallest fragment was related to green-AgNPs treated DNA in comparison with chem-AgNPs treated DNA and untreated ones ().
Discussions
There are several methods for the synthesis of silver nanoparticles of various sizes, shapes, and morphologies. The physicochemical properties of the silver nanoparticles allow these materials to be the potential candidates for use in biomedical, pharmaceutical and healthcare related issues. In the present study, we studied the antibacterial activity of green and chemically synthesized AgNPs on both Gram-positive and Gram-negative bacteria and evaluated the antioxidant activity of NPs by DPPH radical scavenging activity, as well as the DNA cleavage mechanism by the agarose gel electrophoresis method. Analysis of Uv–vis graphs of silver nanoparticles demonstrates a nucleation process in the green synthesis method, while a strong Ostwald-ripening phenomenon (in which small particles dissolve, and redeposit onto larger particles) was observed in chemical synthesis method in addition to the nucleation process. Powerful reducing activity in green procedure was clearly shown () with an absorbance of 1.3 that is higher than 0.35 for the chemical procedure at the same time. Also a wider size distribution pattern of the resulted nanoparticles in chemical method confirms more intensity in Ostwald-ripening phenomenon that was obtained from spectroscopy data. The results of zeta potential analysis demonstrated that although stability of chem-AgNPs was more than green-AgNPs after synthesis reaction, the stability of chem-AgNPs reduces faster than green-AgNPs during the time (). In the current study, size distribution ranges of green and chemically synthesized silver nanoparticles were narrower than previously reported ones confirming the improvement of synthesizing methods [Citation45–47]. For demonstration of phytochemical coating of the green-AgNPs FTIR analysis test was performed. Due to the similarity of the FTIR peaks for green-AgNPs and the plant extract, the extract precipitation on the nanoparticles could be concluded. Slight differences between intensity and position of the peaks could result from the interaction of chemical bonds of the phytochemicals with the nanoparticles. Interestingly, more similarity and peak adaptation of the nanoparticles and the extract was observed compared to a similar study on a plant from the same genus (Datura metel) [Citation46]. It demonstrates that phytochemicals that were presented in the extract were stably precipitated on nanoparticles and did not separate after three times washing steps applied through centrifugal forces. Microscopic characterization demonstrated an isometric arrangement of the green-AgNPs confirming narrow size distribution range obtained from DLS data. These results could be compared with a wide size range of nanoparticles that have been synthesized previously by Datura metel leaf extract [Citation48]. XRD patterns of chem-AgNPs were similar with previously studied silver nanoparticles synthesized by Polyvinylpyrrolidone (PVP) as a reducing agent [Citation49]. XRD pattern of green-AgNPs demonstrated some additional peaks. They are apparent in many works in which the XRD pattern included the relevant 2θ range. These peaks were due to the organic compounds that were present in the extract. Based on the results phytochemical coating of green synthesized nanoparticles caused some additional peaks at the X-ray spectra in comparison with sharp and unique peaks for chemically synthesized nanoparticles [Citation50]. The antioxidant activity of D. stramonium aqueous extract has been previously reported, and it was very similar to the current study [Citation51]. Also, due to a minimum antioxidant effect at the higher concentration of the chem-AgNPs, it can be concluded that the nanoparticles have oxidative effects. Moreover, oxidative AgNPs caused a variety of ROS mediated stress responses, including apoptosis, DNA damage and autophagy [Citation52]. This result approves phytochemical coating of green- AgNPs in the presence of the plant extract similar with other plants (longum, Datura metel) delivering their own antioxidant phytochemicals on the nanoparticles [Citation47,Citation53]. The percentage of DPPH radical scavenging activity and colour trends from low to high concentrations of the extract, green-AgNPs and chem-AgNPs was shown in the related charts at different concentrations (). Three typical antibacterial tests were applied on both types of the nanoparticles. Silver nanoparticles had more antibacterial effects on E. coli than S. aureus in well diffusion and MIC assays that is possibly due to the difference in the structure of cell wall between Gram-positive and Gram-negative bacteria [Citation54]. However, in the present study, it was clearly expressed that green-AgNPs were more effective in the three antibacterial assays comparing to the extract, chem-AgNP and AgNO3. In MIC assay, AgNO3 had maximum antibacterial effect in the lowest concentration against both the types of bacteria. This result could be due to the agglutination potential of AgNO3 in high concentrations in presence of bacterial metabolites [Citation55]. Based on these assays confirming less antibacterial effect for chem-AgNPs and plant extract in comparison with green-AgNPs, the synergistic antibacterial effect of the plant extract metabolites and silver nanoparticles can be raised. This is possibly due to the inability of the plant extract to penetrate into bacterial cells, while when they are added on the nanoparticle surface, will be able to affect bacterial cells in addition to the antibacterial effect of the nanoparticles themselves. The results show enhanced effect of green synthesized nanoparticles through coating by phytochemicals [Citation56]. Previous studies indicated that the silver ion released from AgNPs was responsible for antibacterial activity [Citation57]. The high bactericidal activity of silver nanoparticles is due to their extremely large surface area, which provides better contact with microorganisms [Citation58]. Moreover, silver nanoparticles act as reservoirs for bactericidal agents. In the current study, a combination of silver nanoparticles coated with the plant phytochemicals revealed a synergistic effect on the antimicrobial activity against E. coli and S. aureus. However, advance studies are required to identify and characterize the bioactive compounds responsible for the activity. Possible antimicrobial substances contained in the extract are alkaloids, saponins, tannins, flavonoids and glycosides [Citation59]. The presence of secondary metabolites in single or in combination with others could be responsible for the antibacterial activity of the plant. Finally, DNA digestion could be considered as an antimicrobial mechanism for synthesized nanoparticles based on the results obtained from DNA cleavage activity test. In previous studies, it was found that DNA strand break formation is likely correlated to silver ion release that is strongly dependent on AgNP size, with smaller NPs inducing more damage than larger particles. Regarding the larger size of green-AgNPs than chem-AgNPs and approximate removal of the DNA band after green-AgNPs treatment, the hypothesis of alkaloid interaction presenting on the particles is strong because of its antibacterial effect through DNA digestion [Citation60,Citation61].
Conclusions
Various methods have been reported previously for synthesis of AgNPs using different materials as capping and reducing agents and their antibacterial effects have been studied. Here, a comparison was carried out between green and chemically synthesized silver nanoparticles differing in reducing and capping agents. The green-AgNPs were found to be of spherical shape, isotropic and stable. They also revealed significant antibacterial activity and because oxidative activity of chemically synthesized ones, green-AgNPs could be an effective substantial of chemical nanoparticles for medical purposes. In the current study, the DNA cleavage activity of green-AgNPs was confirmed. Hence, this mechanism could be responsible for the antibacterial effects. Consequently, green synthesized silver nanoparticles have the potential for external use as a biological agent on microorganisms and bacteria.
References
- Hassouna ME, ElBably MA, Mohammed AN, et al. Assessment of carbon nanotubes and silver nanoparticles loaded clays as adsorbents for removal of bacterial contaminants from water sources. J Water Health. 2017;15:133–144.
- Anandhakumar S, Sasidharan M, Tsao CW, et al. Tailor-made hollow silver nanoparticle cages assembled with silver nanoparticles: an efficient catalyst for epoxidation. ACS Appl Mater Interfaces. 2014;6:3275–3281.
- Pletsch H, Greiner A, Agarwal S. Preparing a pseudo-solid by the reinforcement of a polydentate thioether using silver nanoparticles. Nanoscale. 2015;7:1977–1983.
- Sarkar A, Fatima I, Jamal QM, et al. Nanoparticles as a carrier system for drug delivery across blood brain barrier. Curr Drug Metab. 2017;18:129–137.
- Liu R, Lal R. Synthetic apatite nanoparticles as a phosphorus fertilizer for soybean (Glycine max). Sci Rep. 2014;4:5686.
- Kelly KL, Coronado E, Zhao LL, et al. The optical properties of metal nanoparticles: the influence of size, shape, and dielectric environment. J Phys Chem B 2003;107:668–677.
- Marambio-Jones C, Hoek EMV. A review of the antibacterial effects of silver nanomaterials and potential implications for human health and the environment. J Nanopart Res. 2010;12:1531–1551.
- Kim KJ, Sung WS, Moon SK, et al. Antifungal effect of silver nanoparticles on dermatophytes. J Microbiol Biotechnol. 2008;18:1482–1484.
- Lara HH, Ayala-Nunez NV, Ixtepan-Turrent L, et al. Mode of antiviral action of silver nanoparticles against HIV-1. J Nanobiotechnol. 2010;8:1.
- Khan Z, Al-Thabaiti SA, Obaid AY, et al. Preparation and characterization of silver nanoparticles by chemical reduction method. Colloids Surf B Biointerfaces. 2011;82:513–517.
- Raffi M, Rumaiz AK, Hasan MM, et al. Studies of the growth parameters for silver nanoparticle synthesis by inert gas condensation. J Mater Res. 2007;22:3378–3384.
- Ashraf JM, Ansari MA, Khan HM, et al. Green synthesis of silver nanoparticles and characterization of their inhibitory effects on AGEs formation using biophysical techniques. Sci. Rep. 2016;6:20414.
- Saif S, Tahir A, Chen Y. Green synthesis of iron nanoparticles and their environmental applications and implications. Nanomaterials (Basel). 2016;6:209.
- Chen X, Schluesener HJ. Nanosilver: a nanoproduct in medical application. Toxicol Lett. 2008;176:1–12.
- Ahamed M, Alsalhi MS, Siddiqui MK. Silver nanoparticle applications and human health. Clin Chim Acta. 2010;411:1841–1848.
- Foldbjerg R, Olesen P, Hougaard M, et al. PVP-coated silver nanoparticles and silver ions induce reactive oxygen species, apoptosis and necrosis in THP-1 monocytes. Toxicol. Lett. 2009;190:156–162.
- Almofti MR, Ichikawa T, Yamashita K, et al. Silver ion induces a cyclosporine a-insensitive permeability transition in rat liver mitochondria and release of apoptogenic cytochrome C. J. Biochem. 2003;134:43–49.
- Sanyasi S, Majhi RK, Kumar S, et al. Polysaccharide-capped silver nanoparticles inhibit biofilm formation and eliminate multi-drug-resistant bacteria by disrupting bacterial cytoskeleton with reduced cytotoxicity towards mammalian cells. Sci. Rep. 2016;6:24929.
- Katti K, Chanda N, Shukla R, et al. Green nanotechnology from cumin phytochemicals: generation of biocompatible gold nanoparticles. Int J Green Nanotechnol Biomed. 2009;1:B39–B52.
- Slawson RM, Van Dyke MI, Lee H, et al. Germanium and silver resistance, accumulation, and toxicity in microorganisms. Plasmid. 1992;27:72–79.
- Meenal K, Shriwas A, Sharmin K, et al. Extracellular synthesis of silver nanoparticles by a silver-tolerant yeast strain MKY3. Nanotechnology. 2003;14:95.
- Bhainsa KC, D'Souza SF. Extracellular biosynthesis of silver nanoparticles using the fungus Aspergillus fumigatus. Colloids Surf B Biointerfaces. 2006;47:160–164.
- Ahmed S, Ahmad M, Swami BL, et al. A review on plants extract mediated synthesis of silver nanoparticles for antimicrobial applications: a green expertise. J Adv Res. 2016;7:17–28.
- Makarov VV, Love AJ, Sinitsyna OV, et al. “Green” nanotechnologies: synthesis of metal nanoparticles using plants. Acta Naturae. 2014;6:35–44.
- J, Lee; E, Park; J. Lee Non-toxic nanoparticles from phytochemicals: preparation and biomedical application. Bioprocess Biosyst Eng. 2014;37:983–989.
- Dwivedi AD, Gopal K. Biosynthesis of silver and gold nanoparticles using Chenopodium album leaf extract. Colloids Surf A Physicochem Eng Asp. 2010;369:27–33.
- Bhardwaj K, Kumar S, Ojha S. Antioxidant activity and FT-IR analysis of Datura innoxia and Datura metel leaf and seed methanolic extracts. Afr. J. Tradit. Complement. Altern. Med. 2016;13:7–16.
- Gajendran B, Chinnasamy A, Durai P, et al. Biosynthesis and characterization of silver nanoparticles from Datura inoxia and its apoptotic effect on human breast cancer cell line MCF7. Mater Lett. 2014;122:98–102.
- Murugan K, Dinesh D, Kumar PJ, et al. Datura metel-synthesized silver nanoparticles magnify predation of dragonfly nymphs against the malaria vector Anopheles stephensi. Parasitol Res. 2015;114:4645–4654.
- Soni P, Siddiqui AA, Dwivedi J, et al. Pharmacological properties of Datura stramonium L. as a potential medicinal tree: an overview. Asian Pac J Trop Biomed. 2012;2:1002–1008.
- Eftekhar F, Yousefzadi M, Tafakori V. Antimicrobial activity of Datura innoxia and Datura stramonium. Fitoterapia. 2005;76:118–120.
- Reddy U. Antimicrobial activity of Datura stramonium L. and Tylophora indica (Burm.f.) Merr. Pharmacologyonline. 2009;1: 1293–1300.
- Boojar MM, Goodarzi F. The copper tolerance strategies and the role of antioxidative enzymes in three plant species grown on copper mine. Chemosphere. 2007;67:2138–2147.
- B, Solomon N, Berhane S, Wagaw, et al. Antibacterial activity of Datura stramonium against standard and clinical isolate pathogenic microorganisms. J Med Plant Res. 2017;11:501–506.
- Alt V, Bechert T, Steinrucke P, et al. An in vitro assessment of the antibacterial properties and cytotoxicity of nanoparticulate silver bone cement. Biomaterials. 2004;25:4383–4391.
- Long YM, Hu LG, Yan XT, et al. Surface ligand controls silver ion release of nanosilver and its antibacterial activity against Escherichia coli. Int J Nanomedicine. 2017;12:3193–3206.
- Bastús NG, Merkoçi F, Piella J, et al. Synthesis of highly monodisperse citrate-stabilized silver nanoparticles of up to 200 nm: kinetic control and catalytic properties. Chem Mater. 2014;26:2836–2846.
- Krenzelok EP. Aspects of Datura poisoning and treatment. Clin Toxicol (Phila). 2010;48:104–110.
- Nanda A, Saravanan M. Biosynthesis of silver nanoparticles from Staphylococcus aureus and its antimicrobial activity against MRSA and MRSE. Nanomedicine. 2009;5:452–456.
- CLSI. Methods for dilution antimicrobial susceptibility tests for bacteria that grow aerobically, approved standard. 9th ed., Wayne, (PA): Clinical and Laboratory Standards Institute, 2012.
- Balouiri M, Sadiki M, Ibnsouda SK. Methods for in vitro evaluating antimicrobial activity: a review. J Pharm Anal. 2016;6:71–79.
- Jadhav M, Kulkarni S, Raikar P, et al. Green biosynthesis of CuO & Ag-CuO nanoparticles from Malus domestica leaf extract and evaluation of antibacterial, antioxidant and DNA cleavage activities. New J Chem 2018;42:204–213.
- Okafor F, Janen A., Kukhtareva T, et al. Green synthesis of silver nanoparticles, their characterization, application and antibacterial activity. Int J Environ Res Public Health. 2013;10:5221–5238.
- Berciaud S, Cognet L, Tamarat P, et al. Observation of intrinsic size effects in the optical response of individual gold nanoparticles. Nano Lett. 2005;5:515–518.
- Kiruba Daniel SCG, Nazeema Banu B, Harshiny M, et al. Ipomea carnea-based silver nanoparticle synthesis for antibacterial activity against selected human pathogens. J Exp Nanosci. 2014;9:197–209.
- Ranoszek-Soliwoda K, Tomaszewska E, Socha E, et al. The role of tannic acid and sodium citrate in the synthesis of silver nanoparticles. J Nanoparticle Res 2017;19:273.
- Reddy NJ, Nagoor Vali D, Rani M, et al. Evaluation of antioxidant, antibacterial and cytotoxic effects of green synthesized silver nanoparticles by Piper longum fruit. Mater Sci Eng C Mater Biol Appl 2014;34:115–122.
- Kesharwani J, Yoon K-Y , Hwang J, et al., Phytofabrication of Silver Nanoparticles by Leaf Extract of Datura metel: Hypothetical Mechanism Involved in Synthesis. J Bionanosci. 2009;3:39–44.
- Song Y, Liu G, Wang J, et al. Synthesis and luminescence resonance energy transfer based on noble metal nanoparticles and the NaYF(4):Tb(3)(+) shell. Phys. Chem. Chem. Phys. 2014;16:15139–15145.
- Halawani EM. Rapid biosynthesis method and characterization of silver nanoparticles using Zizyphus spina christi leaf extract and their antibacterial efficacy in therapeutic application. J Biomater Nanobiotechnol. 2017;8:22–35.
- Ananth A, Rajan S. In-vitro antioxidant activity of Datura stramonium L. leaves. Advances in Applied Science Research. 2015;6:147–151.
- Mao B-H, Chen Z-Y, Wang Y-J, et al. Silver nanoparticles have lethal and sublethal adverse effects on development and longevity by inducing ROS-mediated stress responses. Sci. Rep. 2018;8:2445.
- Dipankar C, Murugan S. The green synthesis, characterization and evaluation of the biological activities of silver nanoparticles synthesized from Iresine herbstii leaf aqueous extracts. Colloids Surf B: Biointerfaces. 2012;98:112–119.
- Jung WK, Koo HC, Kim KW, et al. Antibacterial activity and mechanism of action of the silver ion in Staphylococcus aureus and Escherichia coli. Appl Environ Microbiol. 2008;74:2171–2178.
- Lin IW-S, Lok C-N, Che C-M. Biosynthesis of silver nanoparticles from silver(i) reduction by the periplasmic nitrate reductase c-type cytochrome subunit NapC in a silver-resistant E.coli. Chem Sci. 2014;5:3144–3150.
- Jain S, Mehata MS. Medicinal plant leaf extract and pure flavonoid mediated green synthesis of silver nanoparticles and their enhanced antibacterial property. Sci. Rep. 2017;7:15867.
- Feng QL, Wu J, Chen GQ, et al. A mechanistic study of the antibacterial effect of silver ions on Escherichia coli and Staphylococcus aureus. J Biomed Mater Res. 2000;52:662–668.
- Losasso C, Belluco S, Cibin V, et al. Antibacterial activity of silver nanoparticles: sensitivity of different Salmonella serovars. Front Microbiol. 2014;5:227.
- Cowan MM. Plant products as antimicrobial agents. Clin Microbiol Rev. 1999;12:564–582.
- Poojary MM, Vishnumurthy KA, Adhikari AV. Extraction, characterization and biological studies of phytochemicals from Mammea suriga. J Pharm Anal. 2015;5:182–189.
- Butler KS, Peeler DJ, Casey BJ, et al. Silver nanoparticles: correlating nanoparticle size and cellular uptake with genotoxicity. Mutagenesis. 2015;30:577–591.