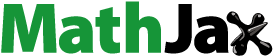
Abstract
Nano-erythrocyte coating has been developed as an interesting biomimetic platform to provide hybrid nano-carriers with innate functions to target liver cancer. This goal was achieved by coating nano-erythrocyte membranes (NEMs) onto 5-fluorouracil (5-FU)-loaded liposomes (LPs) to produce NEM-5-FU-LPs. This framework is used to promote the escape of 5-FU-LPs from degradation during systemic circulation. NEMs were obtained by hypotonic lysis of erythrocytes to produce ghost erythrocytes (GEs) followed by extrusion through polycarbonate membranes. Chimeric NEM-5-FU-LPs were fabricated via the fusion of NEMs and artificial LPs. The resultant chaperoned LPs were characterized based on particle size, morphology, entrapment efficiency (EE %), stability, protein content and phosphatidylserine exposure and their in vitro release profiles and cytotoxic efficacy were also determined. The present results revealed that 5-FU-LPs, NEM-5-FU and NEM-5-FU-LPs exhibited nanosize, spherical shapes and unimodal size distributions <0.3. In addition, the vesicles presented a zeta potential with EE% of 24.6–30.7% and an appropriate stability for 3 weeks. NEM-5-FU-LPs retained the erythrocyte membrane proteins as confirmed by PAGE and displayed a sustained release profile up to 48 h when compared to NEM-5-FU and the 5-FU solution. Moreover, hybrid NEM-5-FU-LPs induced a late cytotoxic effect after 48 h compared to the other formulations. Thus, mantling of 5-FU-LPs by NEMs could enhance vesicle controllability and their targetability to liver cancer cells.
Introduction
Cancer is one of the leading causes of mortality worldwide. Cancer results from exposure to carcinogens, disruption of the DNA repair machinery, failure in the apoptotic process and abnormal cell cycle control, which lead to uncontrolled proliferation [Citation1]. Hepatocellular carcinoma (HCC) is the third leading cause of cancer-related deaths worldwide [Citation2] and is associated with poor diagnosis and death [Citation3]. Although chemotherapy is used as an essential strategy to treat liver cancer, HCC is insensitive to chemotherapy [Citation4].
The anti-metabolite drug, 5-Fluorouracil (5-FU), has been extensively used as an anticancer drug. Its cytotoxicity results from the deleterious effect exerted on DNA and RNA, which mediates many events that lead to cell cycle arrest. The recommended dose of 5-FU is 12 mg/kg/day for 4 days via IV infusion or 12 mg/kg/4 days via IV bolus [Citation5]. From a clinical perspective, the use of 5-FU is limited owing to its short half-life and a lack of selectivity. Moreover, 5-FU elicits life-threating effects such as cardiotoxicity, myelosuppression, and neurotoxicity. These issues are related to a high dose of 5-FU and its associated non-specific biodistribution [Citation5]. Therefore, further research regarding 5-FU in the field of drug delivery is still warranted.
Several strategies have been developed to improve the targeting and selective accumulation of chemotherapeutic drugs in tumour cells. Nanomedicine is one of the promising tactics that have been developed to achieve this goal [Citation6]. Chandran et al. [Citation7] reported several implications of a nano-delivery system for improved therapeutic outcomes of 5-FU [Citation7]. Nanocarriers abundantly accumulate in tumour tissues owing to enhanced permeability and retention (EPR) effect. Several synthetic and natural polymers have been used to design nano-shuttles as drug delivery systems. The nanocarriers might target tumour cells with acceptable biodistribution, cellular uptake and localization patterns [Citation5]. Although nanocarriers represent smart drug delivery systems, they are associated with opsonization and clearance by the immune system [Citation8]. Further studies are therefore required to resolve these issues.
In early studies, polyethylene glycol coating was employed to decrease the immunological issues associated with nanocarriers; however, this method is linked to the decreased interaction between target cells and drug cargoes [Citation9,Citation10]. More recently, hybrid nano-delivery systems have been employed as a novel platform to decrease the elimination of artificial nanocarriers [Citation11]. In this regard, Hu et al. [Citation11] demonstrated that bio-functionalized nanocarriers are non-immunogenic [Citation11]. Furthermore, the biomimetic nanocarriers could deliver drugs to the liver, spleen, lungs, bone marrow and other tissues in a selective manner [Citation12]. Advanced nanobiology has yielded many cell-membrane mimicking nano-cargoes, including liposomes (LPs) and cell membrane-derived nanovesicles [Citation13], through the use of biomembrane derived from blood cells, stem cells, hepatocytes and cancer cells [Citation5]. The incorporation of a functional membrane character allows the transfer of biological properties to LPs, improving their stability during circulation and increasing the targeting capability with efficient intracellular delivery [Citation14].
Erythrocytes, ghost erythrocytes (GEs) and nanovesicles from erythrocyte membranes (NEMs) are mainly used as drug delivery systems and biological coats [Citation15,Citation16]. Moreover, these carriers passively and actively target liver cells and possess many desirable properties for anticancer drug delivery [Citation17]. It has been demonstrated that nanocarrier-loaded erythrocytes have higher cytotoxicity against the HepG2 cell lines than the free drug [Citation18]. Moreover, NEMs represent a promising delivery system with high targeting efficiency. They possess a large surface and prolonged circulation time in the blood. NEMs could be mantled as artificial nano-systems that form hybrid nanocarriers [Citation9]. Such delivery system provides a novel approach to the coating of 5-FU-LPs to overcome clearance by the mononuclear phagocytic system [Citation19].
In the current study, a top-down biomimetic approach was exploited to combine both artificial (LPs) and biological vesicles (NEMs) to develop a chimeric drug delivery system. The 5-FU-LPs were prepared and coated by NEMs, with NEMs prepared by hypotonic lysis of erythrocytes followed by extrusion through polycarbonate membranes. NEM particle size, morphology and entrapment efficiency (EE) were determined, and protein content and phosphatidylserine exposure were measured to confirm the native properties of the biomembranes. The hybrid nanocarriers (NEM-5-FU-LPs) were synthesized by the fusion of NEMs with LPs and were characterized by particle size, morphology, EE, stability and in vitro release profiles. A cytotoxicity study for all formulations was also performed using HepG2 cell lines.
Material and methods
Materials
MTT (3–(4,5-dimethyl-thiazol-2-yl)- 2,5-diphenyltetrazolium bromide) and 5-FU were purchased from Sigma-Aldrich (St. Louis, MO, USA). Dipalmitoyl phosphatidylcholine (DPPC) and 1-oleoyl-2-[12-biotinyl (aminododecanoyl)]-sn-glycero-3-phosphocholine were purchased from Lipoid KG (Germany). The HepG2 cell line was obtained from American type cell culture (ATCC, Rockville, MD, USA). All other reagents and chemicals were of analytical grade.
HPLC analysis
The concentration of 5-FU was analyzed using reverse phase-High Performance Liquid Chromatography (HPLC). The HPLC system (Waters™ 600 controller, USA) was comprised of a detector (Waters™ 2487, a Dual Absorbance detector, USA), pump (Waters™ 1252, a Binary pump, USA) and an autosampler system (WatersTM 717 Plus Autosampler, USA). Results from the HPLC system were analyzed using Empower software (Waters, USA). Analysis was performed under isocratic elution using 40 mM phosphate buffer (pH 7.0) as the mobile phase and a flow rate of 1 ml/min at room temperature. The mobile phase was run on a reversed-phase C18 column (Bondapak™, 4.6 × 150 mm, 10 mm particle size). The volume of injection was 30 µl and detection was performed using a UV detector at 260 nm [Citation20].
Preparation of LP-5-FU
The 5-FU-LPs were prepared using the thin film hydration method as described previously [Citation21]. Briefly, 100 mg of lipid consisting of 2 M DPPC and 1 M cholesterol was dissolved in a mixture of chloroform and methanol (2:1, v/v) in a round bottom flask. The organic solvents were then evaporated to obtain a dry lipid film using a rotary evaporator (Buchi Rotavapor R-200, Switzerland) under vacuum at 55 C for 2 h. Flushing was performed with nitrogen gas to ensure complete removal of organic solvents from the round bottom flask. The dry lipid film was then rehydrated with phosphate buffer (PBS, pH 7.4) containing 5-FU (2 mg/ml) using the rotary evaporator for 1 h at 55
C to obtain multi-lamellar vesicles (MLVs). The MLVs were down-sized using probe-sonication at 60% power for 1 min and extruded through a polycarbonate membrane to achieve the desired size of LPs (<200 nm). The formed LPs were ultra-centrifuged at 30,000 rpm for 30 min at 4 °C to remove free 5-FU. The obtained pellets were re-suspended in 2 ml PBS and stored at 4 °C for further investigation [Citation22].
Preparation of NEM-5-FU-LPs
NEMs were produced from GE according to a previous report by Done et al. [Citation23]. In brief, fresh whole-blood samples were collected in heparinized tubes from 10 albino rats (age 2 months, weight 200–220 g). The protocol was conducted according to the guidelines of the Research Centre Ethics of King Saud University, College of Pharmacy, Riyadh, Saudi Arabia (Ref. No.: KSU-SE-18–27). The blood samples were centrifuged at 4000 rpm for 15 min at 25 C. The obtained packed erythrocytes were washed three times with ice-cold 1 × PBS (pH 7.4) to remove plasma and buffy coat. The washed erythrocytes were incubated with 3 volumes of hypotonic PBS at 4
C for 20 min to remove intracellular content; centrifugation was then performed at 14,000 rpm at 4 °C for 5 min. The supernatant was removed, and the light-pink pellets (GEs) were collected and washed thrice with 1 × PBS until a colourless supernatant was obtained.
To prepare loaded NEMs, the 5-FU solution and 5-FU-LPs were suspended in 0.2 × PBS of GE at a 1:2 ratio. The resultant mixture was incubated at 4 °C for 1 h with continuous shaking, followed by the addition of 10 × PBS to reseal the membrane nanovesicles at 37 °C for 1 h. The resultant NEMs were washed twice and re-suspended in 1 × PBS.
Subsequently, NEMs were extruded (11 cycles) through 400, 200 and 100 nm polycarbonate membranes using the Avanti Mini Extruder (Avanti Polar Lipids, Inc. USA). The resultants from the extrusion were washed out with 1 × PBS and centrifuged at 13,000 rpm for 15 min 4 °C to obtain NEMs [Citation24].
Characterization of NEM
Particle size and zeta potential
Hydrodynamic diameter, polydispersity index (PDI) and zeta potential were monitored using a Zetasizer Nano ZS (Malvern Instruments, UK). The diameters of the prepared NEM were calculated in dynamic light scattering (DLS) mode at 25 C after dilution. Zeta potential of each formula was measured in laser Doppler velocimetry mode using the Nano ZS at 25
C. All experiments were performed in triplicates. Each value recorded represents the average of three measurements.
Loading efficiency assay
The non-encapsulated 5-FU was separated from the drug-loaded NEM vesicles by centrifugation at 14,000 rpm for 30 min at 4 C. Moreover, drug loading was evaluated by a direct method after lysing the drug-loaded NEM pellets with methanol. For the indirect method, the amount of 5-FU in the supernatant was measured using HPLC.
Drug loading was calculated using the following equation:
Protein content by SDS-PAGE
GE and NEM vesicles were investigated for the protein markers that are commonly associated with normal erythrocyte membranes. These proteins may be conserved through the production of GEs and reserved on the surface of NEM vesicles. The content of the proteins was measured after erythrocyte membrane lysis using a hypertonic buffering system. SDS-PAGE was then used to confirm the presence of erythrocyte membrane proteins. Briefly, GEs, NEM vesicles and NEM-LPs were treated with SDS in buffer solution. The samples were then applied and ran on a polyacrylamide gel electrophoresis apparatus (BioRad, USA) at 100 V for 2 h. Finally, the gel was stained with Coomassie blue for 1 h and was visualized using an electrophoretic imaging system (Aplegen Omega Lum G, USA).
Phosphatidylserine exposure
The presence of the phosphatidylserine (PS) on the external surface of NEM vesicle membrane was quantified by Annexin V binding test according to the manufacturer’s procedures (BD Pharmingen PE Annexin V Apoptosis Detection Kit). Briefly, plain GEs, NEMs, 5-FU-NEMs, and NEM-5-FU-LPs were resuspended in ice-cold Annexin V Binding Buffer (10 mM HEPES (pH 7.4), 140 mM NaCl and 2.5 mM CaCl2) and kept on ice before the addition of Annexin V-FITC solution. The tubes were then incubated for 15 min in the dark. Samples were analyzed within 30 min using flow cytometry, and cells with an FITC fluorescence higher than the cutoff value of the non-specific fluorescence were declared Annexin V positive. The percentage of positive cells was expressed as the ratio of labeled cell to total cell number [Citation25].
The surface morphology
A transmission electron microscope (TEM) was used to visualize the surface morphology of the obtained LPs, GEs, NEM vesicles and NEM-5-FU-LP chimera. In brief, a drop of the sample suspension was deposited onto a glow-discharged carbon-coated grid for 5 min, followed by rinsing of the sample with 15 drops of distilled water; 1% uranyl acetate stain was then added to the grid. The grid was subsequently dried and visualized using TEM (JEM-1011, JEOL, Tokyo, Japan) at 60 kV.
Stability studies
The stability of the obtained formulations was assessed by storing the compounds for 21 days at 4 C. Moreover, the samples were subjected to macroscopical observation and characterized by vesicle size, PDI, zeta potential and protein content, as described previously [Citation23].
In vitro release studies
The in vitro release of 5-FU from LPs, NEM vesicles and NEM-LPs formulations was evaluated using the dialysis membrane method [Citation23]. The dialysis bag (MWCO 5 kDa, Livingstone, NSW, Australia) was soaked in distilled water for 12 h before use. Specific amounts of 5-FU formulations were dispersed in 1 ml of PBS (pH 7.4) and placed in a dialysis bag. The sealed bag was then incubated with 20 ml of preheated PSB (37 C) in beakers as the release medium. The sink conditions for the in vitro release studies were met since the aqueous solubility of 5-FU was reported as approximately 10–12 mg/ml [Citation26]. The beakers were placed in a thermostatic shaker water bath at 37
C and 100 rpm. Two ml of the aliquots was collected at predetermined time points and replenished immediately with an equal volume of fresh PBS. Release of free 5-FU (5-FU suspended in PBS) was performed to serve as a control. The amount of 5-FU in the aliquots was analyzed by HPLC, and the experiments were carried out in triplicates.
Cytotoxicity study
HepG2 cell viability was analyzed using the MTT assay. This method was dependent on a colourimetric reaction based on the conversion of the MTT (yellow) colour to purple (formazan production). This mechanism is attributed to the effect of the tested materials on the activity of mitochondrial succinate dehydrogenase in cells. The cells were maintained in Dulbecco's Modified Eagle Medium (Gibco, Grand Island, NY) supplemented with 10% fetal bovine serum (Gibco) at 37 C in a 5% CO2 humidified incubator. Cells were plated at 1 × 104 cells per well in a 96-well plate. After overnight incubation, the cells were treated with the 5-FU solution (1 mM), 5-FU-LPs, 5-FU-NEMs, and NEM-5-FU-LPs for 3 days. Cell viability was calculated using the following equation:
Statistical analysis
All data analyses were performed using GraphPad InStat software, Version 4 (GraphPad, ISI Software Inc., La Jolla, CA, USA). The results were compared using one-way ANOVA (analysis of variance). A p value <0.05 indicated significance.
Results and discussion
Preparation of NEM-5-FU-LPs
The nano-sized properties of the erythrocyte-derived vesicles demonstrated that an excellent drug delivery system with non-immunogenic characters was synthesized. The delivery system extended the residence time of the drug in circulation and displayed liver targetability. Therefore, nano-sized erythrocytes could be utilized to deliver 5-FU and enhance its therapeutic index. Moreover, NEMs are exploited as chaperones for LPs to govern their surface characters with large loading capacity [Citation13]. The resultant hybrid vesicles represent a promising delivery system to target cancer cells. It has been reported that coating LPs using cells displays an outstanding therapeutic effect [Citation14]. When this is performed, LPs may conserve their ability to deliver the drug to the intracellular milieu to elicit potential effects.
In the present study, TEM revealed the formation of GEs and NEM vesicles as opposed to intact erythrocytes. The 5-FU-LPs were added to GEs followed by an extrusion process to form NEM-5-FU-LPs. The obtained hybrid vesicles contained 5-FU with a nano-size 200 nm after successive extrusion through 400, 200 and 100 nm polycarbonate membranes. The results aligned with those of several studies where erythrocyte-derived nano-vesicles were used for nano-system cloaking [Citation27].
Particle size and zeta potential of vesicles
In the current study, diameter and PDI were measured using DLS mode at 25 C when the proper dilutions were made. NEM-5-FU showed a hydrodynamic diameter of 155.69 ± 8.9 nm, with a unimodal size distribution of 0.325 ± 0.018. When NEMs encapsulated the 5-FU-LPs, the size of the formed vesicles increased to 191.4 ± 14.76 nm with a unimodal size distribution of 0.245 ± 0.012. Therefore, encapsulation of 5-FU-LPs into NEM vesicles had a significant effect on particle size. This may be attributable to the double bilayers from the phospholipid of LPs and NEMs. The current results agree with those of a previous investigation published by Zhang et al. [Citation28]. In addition, it was observed that the PDI for NEM-5-FU-LPs was lower than that of other formulations. This might be explained by the presence of lipids led to the dilution of protein corona at the surface of 5-FU-LPs, inducing less aggregation, and hence a lower PDI.
Zeta potential is a significant property that is responsible for stabilized NEM suspension to prevent cell aggregation. In addition, it is essential to prevent interactions between nano-erythrocytes and other cells in the vascular system [Citation29]. The negative charge also prevents clumping of the formulation. 5-FU-LPs, NEM-5-FU and NEM-5-FU-LPs exhibit negative ZP. These results suggest that biocompatible drug delivery shuttles were created to prevent the interaction between NEM-LPs and vascular cells. Likewise, a previous study reported that preservation of the negative charge from the erythrocyte membrane could stabilize the erythrocyte suspension [Citation29].
Entrapment efficiency
In the present study, different concentrations of 5-FU were loaded onto LPs and then coated with NEMs of varying concentrations (1, 5 and 10 mg/ml; ). The present results showed that the EE % of NEMs was 3.549 ± 0.162, 6.066 ± 0.137 and 24.62 ± 6.99% after the addition of 5-FU (1, 5 and 10 mg/ml, respectively). On the other hand, the EE% of NEM-5-FU-LPs was 5.051 ± 0.508, 8.019 ± 0.591 and 30.71 ± 2.58% when 1, 5 and 10 mg/ml of 5-FU was loaded, respectively. shows that the amount of drug entrapped increased when the concentration increased from 1 to 10 mg/ml. This result aligns with a previous study that proved a higher loading efficiency could be obtained by increasing drug concentration [Citation23].
SDS-PAGE
The erythrocyte membrane proteins offer the main intriguing functional advantages for the use of erythrocytes as a drug delivery system [Citation25]. Therefore, SDS-PAGE was performed to confirm the presence of erythrocyte membrane proteins on GEs, NEM-5-FU and NEM-5-FU-LPs. As displayed in , GEs and NEMs retained the membrane proteins inherited from the erythrocyte membrane, and NEM-5-FU-LPs showed a similar protein profile. SDS-PAGE results, therefore, confirmed the coating of erythrocyte membranes with their surface proteins on LPs. The detection of these protein markers on the surface of chaperoned liposomes suggests that fusion indeed occurred. The similarities in the protein marker profiles of NEM and NEM-LPs were also noticed, therefore, the protein markers on the NEM are preserved in the production of NEM-LPs.
Figure 2. SDS-PAGE and TEM of ghost erythrocytes, nanoerythrocyte membrane and hybrid nanoerythrocyte-membrane-coated liposomes. (Scale bar for TEM = 100 nm).
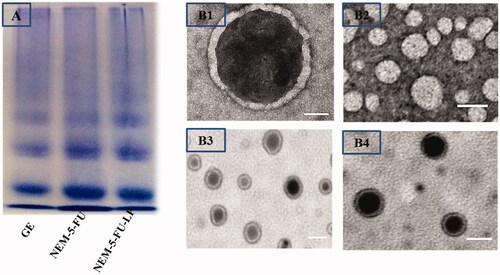
In addition, the presence of NEMs on the surface of 5-FU-LPs was visually proven using TEM imaging, as shown in . The TEM images displayed a spherical shape with nano-sized 5-F-LPs and NEM-5-FU vesicles, with smooth surface and no aggregation. Moreover, NEM-5-FU-LPs exhibited an inner core region bounded by a thin shell due to the presence of the NEM layer (); these results agree with those presented by Zhang et al. [Citation28].
Phosphatidylserine exposure
Phosphatidylserine is considered a potential marker for rapid clearance of erythrocyte by macrophages to liver and spleen cells [Citation18]. Phosphatidylserine on the outer layer of the membrane is the premier key in cellular targeting. Thus, phosphatidylserine exposure on the surface of NEM-5-FU-LPs was measured using flow cytometry via the binding of Annexin V-FITC to phosphatidylserine (). The data revealed that plain GEs and NEMs showed less amounts of phosphatidylserine exposure on their surface. In contrast, NEM-5-FU-LPs displayed an overbearing fluorescence compared to that of NEM-5-FU due to the exposure of more phosphatidylserine on the NEM surface. These data confirmed the successful fusion of NEMs to 5-FU-LPs, with a reorganization of phosphatidylserine in the membrane bilayer [Citation18,Citation30]. Therefore, this structural membrane change could lead to high recognition ability by macrophages with the prospects of liver targeting [Citation18,Citation25,Citation30].
Stability studies
It is highly recommended to investigate the physical stability of the obtained nanocarriers for prospective applications. In the present study, particle size, PDI, zeta potential and protein content were evaluated after 21 days (). The 5-FU-LPs, NEM-5-FU and NEM-5-FU-LPs exhibited good stability as indicated by slight changes in particle size and zeta potential over the 21-day period (). Furthermore, the formulations maintained their negative surface charge over the 21 days (), and PDI remained relatively within a moderate range (0.5) (). This minor change may be attributed to slight particle aggregation caused by the fusion of small amounts of vesicles in the particle solution [Citation23]. Surface proteins were also examined by SDS-PAGE over a span of 21 days as an indicative tool of stability (). These data show the presence of protein bands of GEs, NEM-5-FU and NEM-5-FU-LPs, indicating good stability of NE-5-FU-LPs due to the shielding influence of cell membrane coating [Citation23,Citation31].
In vitro release
The in vitro release profile of the 5-FU solution, 5-FU-LPs, NEM-5-FU and NEM-5-FU-LPs was examined in PBS buffer at 37 °C. The release of the 5-FU solution was evaluated using a dialysis bag; ∼100% of the drug was available in the receptor site within the first 4 h. Moreover, within the first 4 h, the burst release of 5-FU from NEM-5-FU and NEM-5-FU-LPs was approximately 72% and 66%, respectively, compared to 84% from the 5-FU-LPs (). Within 48 h, 92.5 ± 3.23%, 76.9 ± 1.09%, and 70.3 ± 1.51% () of 5-FU were released from 5-FU-LPs, NEM-5-FU and NEM-5-FU-LPs, respectively. Therefore, NEM-5-FU-LPs displayed good sustained-release behaviour, which can be attributed to the double layers of the NEM vesicles and artificial membrane of LPs. These data suggest that NEM-5-FU LPs could be used to improve the targeting of 5-FU to cancer cells [Citation24].
Cytotoxicity assay
To evaluate the in vitro cytotoxicity of 5-FU LPs, NEM-5-FU and NEM-5-FU-LPs, MTT assays were performed using HepG2 cells as the characteristic cancer cells (). Treatment of cells with LPs, NEMs and NEM-LPs resulted in negligible cell toxicity (>91% survival rate); this depicts the safety of the carriers. Moreover, cells treated with the 5-FU solution showed a higher cytotoxic effect, resulting from free 5-FU from both LPs and NEMs. Owing to treatment for up to 24, 48 and 72 h with 5-FU-LPs, NEM-5-FU and NEM-5-FU-LPs, cell viability decreased from 57.33% to 19.41%, 78.71% to 39.06% and 87.11% to 44.30%, respectively. This might be due to the differences in 5-FU concentration within cells at a given time, based on the carrier used [Citation23]. The delaying cytotoxic effect of NEM-5-FU-LPs might be the sustained release of 5-FU up to 48 h when compared to that by other formulations, as indicated in the in vitro release study. The fusion of the protein from NEM onto the LPs might be contributed to improved cellular entry and therefore the inhibition of HepG2 cell growth activity was increased.
Figure 6. Cytotoxicity studies of 5-FU liposome, 5-FU solution, 5-FU loaded nanoerythrocytes and 5-FU liposome loaded nanoerythrocytes. Data expressed as M ± SD, N = 3.
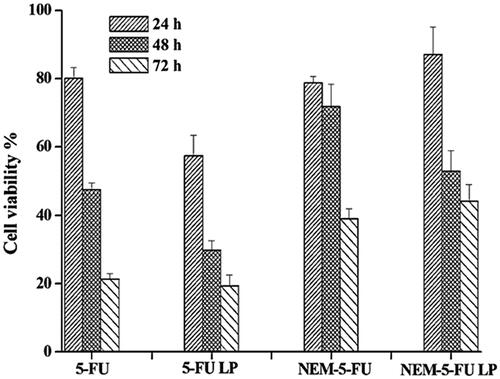
The data obtained from this study indicate that the use of chimeric nanoparticles can be pursued as an interesting aspect of personal medicine. In this regard, erythrocytes can be extracted from a patient and their membranes used to mantle the artificial nanoparticle before re-injection into the same patient. This could provide biofunctionalized nano-carriers containing therapeutic cores and minimize the immune clearance of the encapsulated drug [Citation32]. The NEM-coated LPs reported in this study are structurally similar to the commonly named hybrid LPs, emerging as a promising multifunctional drug delivery shuttle that contains the desirable characteristics of both erythrocyte and LPs. Additional studies are necessary to optimize biofunctionalized nano-carriers for application to various types of diseases, including cancers.
Conclusion
The data obtained from this study suggest that 5-FU-LPs, NEMs and NEM-LPs were successfully prepared as drug delivery shuttles for 5-FU target delivery; this was also achieved with desirable cytotoxicity. The tailored compounds displayed spherical shapes with nanosized diameter and small size distribution (PDI < 0.3). Particularly, NEM-5-FU-LP vesicles presented a negative charge with reasonable EE (∼31%) and suitable stability for 3 weeks. NEM-5-FU-LPs demonstrated erythrocyte membrane mimicking characteristics as confirmed by SDS-PAGE, phosphatidylserine exposure and TEM. Additionally, NEM-5-FU-LPs displayed a sustained release profile compared to that of NEM-5-FU, LPs and the 5-FU solution. The sustained release patterns of NEM-5-FU-LPs delayed cell viability over 72 h, presenting a good opportunity to enhance in vivo liver cancer targeting. The future progression of natural nano-coating technology promises high efficiency in drug delivery.
Supplement.docx
Download ()Disclosure statement
No potential conflict of interest was reported by the authors.
Additional information
Funding
References
- Naldi I, Taranta M, Gherardin L, et al. Novel epigenetic target therapy for prostate cancer: a preclinical study. PLoS One. 2014;9:98–101.
- Cole AJ, Yang VC, David AE. Cancer theranostics: the rise of targeted magnetic nanoparticles. Trends Biotechnol. 2011;29:323–332.
- Poustchi H, Sepanlou SG, Esmaili S, et al. Hepatocellular carcinoma in the world and the Middle East. Middle East J Dig Dis. 2010;2:31–41.
- Jinsil S. Challenge and hope in radiotherapy of hepatocellular carcinoma. Yonsei Med J. 2009;50:601–612.
- Yanan S, Jing S, Geyi L, et al. Advances of blood cell-based drug delivery systems. Eur J Pharm Sci. 2017;96:115–128.
- Mohanraj VJ, Chen Y. Nanoparticles – a review. Trop J Pharm Res. 2006;5:561–573.
- Chandran SP, Natarajan SB, Chandraseharan S, et al. Nano drug delivery strategy of 5-fluorouracil for the treatment of colorectal cancer. JCRPR. 2017;4:45–48.
- Li W, Zhang L, Zhang G, et al. The finely regulating well-defined functional polymeric nanocarriers for anti-tumor immunotherapy. Mini Rev Med Chem. 2013;13:643–652.
- Brian T, Luk LZ. Cell membrane-camouflaged nanoparticles for drug delivery. J Control Release. 2015;220:600–607.
- Ha D, Yang N, Nadithe V. Exosomes as therapeutic drug carriers and delivery vehicles across biological membranes: current perspectives and future challenges. Acta Pharm Sin B. 2016;6:287–296.
- Hu C-MJ, Zhang L, Aryal S, et al. Erythrocyte membrane-camouflaged polymeric nanoparticles as a biomimetic delivery platform. Proc Natl Acad Sci USA. 2011;108:10980–10985.
- Noble GT, Stefanick JF, Ashley JD, et al. Ligand-targeted liposome design: challenges and fundamental considerations. Trends Biotechnol. 2014;32:32–45.
- Fang RH, Hu CMJ, Luk BT, et al. Cancer cell membrane-coated nanoparticles for anticancer vaccination and drug delivery. Nano Lett. 2014;14:2181–2188.
- Lu M, Zhao X, Xing H, et al. Liposome-chaperoned cell-free synthesis for the design of proteoliposomes: implications for therapeutic delivery. Acta Biomater. 2018;76:1–20.
- Gopal VS, Ranjith KA, Usha AN, et al. Effective drug targeting by erythrocytes as carrier systems. Curr Trends Biotechnol Pharm. 2007;1:18–33.
- Harisa GI, Ibrahim MF, Alanazi F, et al. Engineering erythrocytes as a novel carrier for the targeted delivery of the anticancer drug paclitaxel. Saudi Pharm J. 2014;22:223–230.
- Rossi L, Serafini S, Pierige F, et al. 2005. Erythrocyte-based delivery. Exp Opin Deliv. 2:311–322.
- Harisa GI, Badran MM, AlQahtani SA, et al. Pravastatin chitosan nanogels-loaded erythrocytes as a new delivery strategy for targeting liver cancer. Saudi Pharm J. 2016;24:74–81.
- Gustafson HH, Casper DH, Grainger DW, et al. Nanoparticle uptake: the phagocyte problem. Nano Today. 2015;10:487–510.
- Alanazi FK, Yassin AE, El-Badry M, et al. Validated high-performance liquid chromatographic technique for determination of 5-fluorouracil: applications to stability studies and simulated colonic media. J Chromatogr Sci. 2009;47:558–563.
- Liko F, Suna E, Özer YA, et al. In vitro studies on 5-florouracil-loaded DTPA-PE containing nanosized pegylated liposomes for diagnosis and treatment of tumor. J Liposome Res. 2013;23:61–69.
- Singh V, Krishan P, Shri R. Amelioration of ischaemia reperfusion-induced cerebral injury in mice by liposomes containing Allium cepa fraction administered intranasally. Artif Cells Nanomed Biotechnol. 2018;19:1-11..
- Dong X, Niu Y, Ding Y, et al. Formulation and drug loading features of nano-erythrocytes. Nanoscale Res Lett. 2017;12:202.
- Gupta N, Patel B, Ahsan F. Nano-engineered erythrocyte ghosts as inhalational carriers for delivery of fasudil: preparation and characterization. Pharm Res. 2014;31:1553–1565.
- Kostić IT, Ilić VLJ, Đorđević VB, et al. Erythrocyte membranes from slaughterhouse blood as potential drug vehicles: isolation by gradual hypotonic hemolysis and biochemical and morphological characterization. Colloids Surf B Biointerfaces. 2014;122:250–259.
- Badran MM, Mohsen MM, Magdy MG, et al. Preparation and characterization of polymeric nanoparticles surface modified with chitosan for target treatment of colorectal cancer. Inter J Biol Macromol. 2017;95:643–649.
- Jiang X, Wang K, Zhou Z, et al. Erythrocyte membrane nanoparticles improve the intestinal absorption of paclitaxel. Biochem Biophys Res Commun. 2017;488:322–328.
- Zhang Y, Zhang J, Chen W, et al. Erythrocyte membrane-coated nanogel for combinatorial antivirulence and responsive antimicrobial delivery against Staphylococcus aureus infection. J Control Release. 2017;263:185–191.
- Silva DC, Jovino CN, Silva CA, et al. Optical tweezers as a new biomedical tool to measure zeta potential of stored red blood cells. PLoS One. 2012;7:e31778
- Alanazi FK, Harisa G-D, Maqboul A, et al. Biochemically altered human erythrocytes as a carrier for targeted delivery of primaquine: an in vitro study. Arch Pharm Res. 2011;34:563–571.
- Luk BT, Hu CMJ, Fang RH, et al. Interfacial interactions between natural RBC membranes and synthetic polymeric nanoparticles. Nanoscale 2013;6:2730–2737.
- Rao L, Bu LL, Xu JH, et al. Red blood cell membrane as a biomimetic nanocoating for prolonged circulation time and reduced accelerated blood clearance. Small. 2015;11:6225–6236.