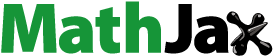
Abstract
The present study encompasses green synthesis of silver nanoparticles (AgNPs) using aqueous leaf extract of Arabian Primrose within 6 min of reaction at 60 °C, pH 7 and their characterisation using physico-chemical analytical techniques. UV-Visible spectroscopy elucidated the surface plasmon resonance around 420 nm. FESEM and TEM images revealed that AgNPs were spherical with average diameter 10–60 nm. XRD pattern confirmed their crystalline nature. The leaf extract rich in phenolics and flavonoids was subjected to GC-MS analysis that identified bioactive compounds helping in reduction and stabilisation of AgNPs. The synthesised AgNPs possessed high anti-oxidant potential against DPPH and H2O2 radicals. Incidentally, the AgNPs acted as excellent nanocatalyst towards borohydride reduction and degradation of structurally different organic dyes. The AgNPs also exhibited selective colorimetric sensing of hazardous mercuric, ferric ions and ammonia. Such AgNPs were cytotoxic against HeLa cells (IC50 7.18 µg/mL) and compatible towards normal L20B cells. These AgNPs showed effective anti-microbial activity against different human pathogens tested (bacterial and fungal). This is probably the first report of AgNPs synthesis using Arabian Primrose leaf extract showing strong anti-oxidant, catalytic, biosensing, anti-cancer and anti-microbial activities and find remarkable applications in medical, industrial and ecological sectors.
Introduction
Nanotechnology has emerged as one of the lucrative areas of research enticing wider applications in biology, optics, mechanics, catalysis, microelectronics, medical, space, energy and material science. In the last decade, metal nanoparticles (Au, Cu, Ag, Mg, Al, Zn, Ti, etc.) have been used tremendously in the area of science and technology. The extremely small size of these nanoparticles presents a very high reactive surface area thereby enhancing their properties compared to their metal compatriots [Citation1]. Among metal nanoparticles, silver nanoparticles (AgNPs) have gained maximum interest as silver is a soft metal having specific thermal, electrical and reflectance properties.
Generally metal nanoparticles are synthesised using physical (ultrasonication, microwave assisted, irradiation, electrochemical techniques) and chemical methods (chemical reduction, inert condensation, photochemical reduction, sol gel method) [Citation2]. In order to reduce the usage of hazardous chemicals, global, concerted and sustained efforts are being made following the principles of green chemistry. In green synthesis, metal salt is allowed to react with biological extracts of bacteria, algae, fungi, or plants. Plant extracts are favoured over microbial extracts because microbes mediated synthesis require highly aseptic conditions and elaborate process of maintaining cell cultures whereas, plant extract mediated synthesis is non-biohazardous. Plant extracts contain primary and secondary metabolites (alkaloids, terpenoids, polysachharides, proteins, etc.) which reduce Ag+, forms a coating over the nanoparticles and helps in their stabilisation. Different parameters such as pH, temperature, time required for reaction, plant extract and metal salt concentration, significantly influence size, shape, morphology, yield and agglomeration state of AgNPs [Citation3].
Synthetic dyes are used extensively in various industries like textile, ceramics, food, glass, paints, cosmetics, polymers, pulp and paper etc. Discharge of dye residues and toxic effluents are a major source of environmental contamination as they impart colour to the water, affecting photosynthesis in plants due to reduced light penetration. Also, they deplete oxygen in the surface waters and streams affecting the aquatic flora and fauna [Citation4]. These dyes in wastewaters are difficult to treat, because they have complex aromatic structure (e.g. anthraquinone, azo, etc.) which are resistant to aerobic digestion, and are stable to oxidising agents. The anaerobic degradation product of azo dyes are amines which are toxic, carcinogenic and mutagenic in nature. A range of conventional treatment technologies for dye removal have been investigated extensively but are not cost-effective and eco-friendly. So, an attempt is made here for rapid degradation of these dyes using AgNPs as nanocatalysts which can be employed in the treatment of effluents.
Nanoparticles can also be employed in sensor studies as the detection and quantification of heavy metal ions play a significant role in environmental monitoring, ecological waste management and clinical toxicological studies. Heavy metals (Hg, Ba, Ni, Cu, Fe, Cr, Zn, Co, Cd, Pb, etc.) have been reported to be pollutants as they are toxic at very low (ppm level) concentrations [Citation5]. The major objective of sensors is the detection of pollutants in the effluents. For sensitivity analysis, usage of metallic nanoparticles is a simple, cost-effective, highly selective and sensitive approach for rapid tracking of toxic metal ions and liquid ammonia in the environment [Citation6,Citation7].
Nanobiotechnological applications of AgNPs also involves their anti-cancer and anti-microbial potency. Silver nanoparticles synthesised using plant extract have been found to effectively inhibit various pathogenic bacteria such as Escherichia coli, E. hermanii, Bacillus subtilis, B. cereus, Klebsiella pneumonia, Pseudomonas aeruginosa, Salmonella typhi, Proteus vulgaris, multidrug resistant bacteria and fungi like Candida albicans, C. glabrata, C. krusei, C. tropicalis and Trichophyton mentagrophytes. In vitro toxicity of AgNPs against various cancer cell lines like HaCat, HeLa, U937, HepG2, HT-29, etc. has been reported [Citation8]. In this modern era of advancement in science and technology, nanomedicine, nanobiotechnology, and nanosensors come under the potential applications of artificial cells that have nano dimension configurations [Citation9].
Arnebia hispidissima, commonly known as Arabian Primrose, is widely distributed in dry sandy areas of Saudi Arabia, Bahrain, Qatar, UAE, Africa, India and Pakistan. Whole plant extract is used as a stimulant, tonic, diuretic, expectorant and in Indian traditional medicine system to treat fevers including malaria. Aerial parts including flowers are known to possess soothing effect in the treatment of heart ailments, tongue and throat infections, cardiac disorder, microbial infections etc. [Citation10,Citation11]. The study aims at synthesis of Arnebia hispidissima funtionalized silver nanoparticles (Ah-AgNPs) with an objective to alleviate the detrimental effects of toxic dyes polluting the environment posing serious ecological concern, to detect toxic heavy metal ions and ammonia in different samples and to study their biomedical potential.
Material and methods
Synthesis and characterization of Ah-AgNPs
The aerial plant parts of Arabian Primrose were collected from arid and semi-arid regions of Rajasthan, India. The leaves were separated, gently washed to remove surface adhered contamination, air dried in shade and ground to fine powder. To prepare aqueous leaf extract, 10 g leaf powder was mixed in 100 ml double distilled water and incubated in water bath at 60 °C for 120 min. The suspension was then filtered through Whatman filter paper no. 1 and stored at 4 °C for further experiments. The synthesis of nanoparticles was carried out by adding 10 ml aqueous leaf extract to 90 ml AgNO3 solution wherein effect of AgNO3 concentration (0.5–5 mM), temperature (40–80 °C), pH (5–11), and time duration (0–6 min) of reaction was studied to optimise the reaction conditions. Characterisation of the synthesised Ah-AgNPs was done following diffferent analytical techniques namely UV-Vis spectroscopy, field emission scanning electron microscopy (FE-SEM), energy dispersive X-ray analysis (EDAX), transmission electron microscopy (TEM), dynamic light scattering (DLS), X-ray diffraction analysis (XRD), Fourier-transform infra-red spectroscopy (FT-IR).
Gas chromatography-mass spectrometry (GC-MS)
The GC-MS analysis of leaf extract (1 mg/mL) was carried out on Shimadzu QP-2010 Plus unit with thermal desorption system (TD 20) including an auto sampler and a gas chromatograph connected to mass spectrometer. Column dimensions were 30 m × 0.25 mm × 0.26 µm with a thin film of 0.26 µm thickness and composed of 5 MS detector (5% diphenyl/95% diphenyl poly siloxane). Running conditions were: carrier gas – Helium (99.999%), injector temperature – 260 °C, flow rate – 1.21 ml/min, run time – 45 min. Phytochemical characterisation of bioactive compounds was done by comparing the obtained mass spectra with National Institute of Standards and Technology (NIST) database.
Catalytic potential of Ah-AgNPs in the dye degradation
The dyes namely methylene blue (MB), crystal violet (CV), methyl red (MR), methyl orange (MO), eosin yellow (EY), trypan blue (TB) and safranin O (SO) were subjected to sodium borohydride (NaBH4) reduction in the presence of Ah-AgNPs in order to assess the catalytic potential of nanoparticles. It was done according to the modified protocol of Nakkala et al. [Citation12]. Briefly, 0.150 M NaBH4 solution was prepared and fresh solution was mixed with 1 mM dye (1 ml). To this, AgNPs suspension (50 µg/mL) was added and agitated well. UV-Vis spectrophotometer was used to scan the wavelength in the range 200–800 nm. For kinetic analysis, the change in absorbance at 662 nm, 585 nm, 394 nm, 460 nm, 515 nm, 594 nm and 516 nm for MB, CV, MR, MO, EY, TB and SO respectively, was recorded. The rate of reaction was determined by the equation given by Sengan et al. [Citation13]:
(1)
(1)
where k is first-order rate constant, t is time duration of reaction, At is absorbance at time t and A0 is absorbance at time 0. The value of k (rate constant) was calculated from the slope of linear part of kinetics data.
Sensing of metal ions and ammonia
The metal ion detection ability of Ah-AgNPs was tested following the modified protocols of Kirubaharan et al. [Citation5] and Rastogi et al. [Citation14]. The test solutions (100 nM) of metal ion (Na+, K+, Mg2+, Ca2+, Ba2+, As3+, Sn2+, Cu2+, Fe2+, Zn2+, Fe3+, Co2+, Cd2+, Ni2+, Pb2+, Cr3+, Mn2+, and Hg2+) were prepared by dissolving their corresponding chloride and nitrate salts in double-distilled water. Ammonia recognition ability was tested according to Pandey et al. [Citation7]. For the preparation of ammonia solution (1–120 ppm) 25% ammonia solution was used. For the detection analysis, 50 µL test solution was added to four times diluted Ah-AgNPs suspension. The change in colour of solution and UV-Vis spectra was monitored.
Quantification of phenolic, flavonoid content of plant extract and in vitro anti-oxidant activity of Ah-AgNPs
Total flavonoid content was quantified according to Eddine et al. [Citation15]. To 250 µL leaf extract (1 mg/mL), 75 µL NaNO2 (5% w/v) was added and incubated for 6 min. Then 150 µL AlCl3 (10% w/v) and 500 µL NaOH (1 N) were added and volume was made up to 2.5 ml using distilled water. Absorbance was recorded at 510 nm. Quercetin (40–200 µg/mL) was taken as positive control. Total flavonoid content in the leaf extract is expressed as mg quercetin equivalents/g of extract (QE mg/g). Similarly, total phenolic content was determined following the protocol of Kamtekar et al. [Citation16] and was calculated as gallic acid equivalents (GAE mg/g of extract). The antioxidant potential of Ah-AgNPs was analysed by DPPH and hydrogen peroxide (H2O2) free radical scavenging assays as per the protocols illustrated previously in Nindawat and Agrawal [Citation17].
Evaluation of anti-cancerous potential
The cytotoxic potential of Ah-AgNPs and aqueous leaf extract was assessed on HeLa cell line (human cervical cancer) and L20 B cell line (non-tumorous mouse cells) based on our earlier study [Citation17]. Methotrexate was used as positive control and percent cell viability was calculated by the following equation and IC50 value was also determined.
(2)
(2)
Evaluation of anti-microbial activity
The anti-microbial potential of Ah-AgNPs and leaf extract was analysed using agar well diffusion method described in detail in Nindawat and Agrawal [Citation17] against the following bacteria – Escherichia coli (ATCC 25922), Klebsiella pneumoniae (ATCC 13883), Enterococcus faecalis (ATCC 29212), Staphylococcus aureus (ATCC 25923) and fungi – Candida tropicalis (ATCC 750), C. albicans (ATCC 24433), Geotrichum candidum (ATCC 7115). Further, the dissolved silver content in Ah-AgNPs suspension was quantified using atomic absorption spectrophotometer (AAS Flame, SensAA, GBC Scientific equipment, Australia).
Statistical analysis
Each experiment was done in triplicates and the data was processed using ANOVA (Analysis of Variance) by SPSS (Statistical Package for Social Science) version 21. Results are presented as average value ± standard deviation for all the experiments performed in triplicates. Significant differences between means were based on Duncan’s multiple range test (DMRT, p = .05).
Results and discussion
Green synthesis of Ah-AgNPs
UV-Vis spectroscopy
On addition of leaf extract to AgNO3 solution, a rapid change in colour of the reaction mixture from light yellow to brown was observed which indicated the production of Ah-AgNPs. This was due to the reduction of Ag+ ions to Ag0 by the bioactive compounds present in leaf extract and the surface plasmon resonance (SPR, λspr) vibrations of the Ah-AgNPs which resulted from coherent oscillation of their conduction electrons with oscillating electromagnetic field of incident light. These oscillations cause unusually strong scattering and absorption properties that depend on shape and size of AgNPs. The synthesised AgNPs displayed maximum absorption peak (λmax) around 420 nm. Different physico-chemical factors play an essential role in the synthesis of appropriate shape and size of AgNPs thereby preventing agglomeration and maintaining their yield. A bathochromic shift in the absorption maxima indicates larger size of nanoparticles and the peak becomes narrow with higher absorption intensity. Increase in the height of absorption peak also indicates higher AgNPs concentration, but at higher concentrations, aggregation occurs due to enhanced collision frequency of nanoparticles [Citation3].
Effect of different physico-chemical parameters on Ah-AgNPs synthesis
AgNO3 concentrations
With increasing AgNO3 concentration, the absorbance was found to increase and bathochromic shift was observed (). Higher AgNO3 concentration boost the yield of nanoparticles, however it causes AgNO3 deposition on the nanoparticles surface making it unclear. A study reported that increase in AgNO3 concentration and reaction time enhanced the yield of AgNPs shifting the λspr to a higher range, pointing towards their agglomeration [Citation18]. So, 0.5 mM AgNO3 was found to be optimum for synthesis of Ah-AgNPs.
Figure 1. (A) Arnebia hispidissima (Lehm.) A. DC (B) Vials showing chromatic variation of the synthesised AgNPs after adding different concentrations of AgNO3 to A. hispidissima leaf extract, effect of (C) AgNO3 concentrations, (D) time, (E) temperature and (F) pH of reaction mixture on AgNPs synthesis using aqueous leaf extract of A. hispidissima.

Time duration of reaction
The maximum absorption peak of Ah-AgNPs increased on increasing the duration of reaction. It was observed that the absorbance maxima (λspr, 420 nm) increased from 0.182 a.u. (0 min) to 0.926 a.u. after 6 min and became stable thereafter depicting the end-point of reduction reaction (). This rapid bioreduction of AgNO3 to Ah-AgNPs is attributed to the presence of phytochemicals in leaf extract which helped in reduction and capping of Ah-AgNPs. Also, the duration of reaction determines the yield of nanoparticles. Further, it was observed that Ah-AgNPs suspension remained well dispersed and unwavered for over 3 months of synthesis.
Temperature of reaction
It is yet another factor crucial for the AgNPs synthesis as it amends the rate of reaction affecting shape and size of AgNPs. With an increase in temperature, increase in absorbance value was observed as it was 0.743 a.u., 1.099 a.u. and 1.677 a.u. at 40, 60 and 80 °C, respectively (). It was observed that 60 °C was optimum for faster reduction of Ag+ ions changing the reaction colour rapidly. It is reported that synthesis of AgNPs at lower temperature (<25 °C) generate narrower peaks with formation of uniform-sized AgNPs while at higher temperatures (>40 °C), broad peaks are observed [Citation19]. Sometimes at higher temperatures, sharper and higher peaks are observed as it depends on the plant or plant parts used and the quantity of extract added [Citation20].
pH of reaction
The characteristic absorbance maxima at pH 7, 9 and 11 were observed around 420 nm whereas at pH 5, it was absent (). The spectra revealed that acidic pH does not favour AgNPs synthesis and alkaline pH is found to be suitable. The pH affects ionisation state of phytochemical compounds present in plant extract responsible for reduction, capping and stabilisation of AgNPs. In our study, pH 7 was found to be optimum. Previous studies also showed that neutral pH favours AgNPs synthesis with slight or no aggregation and AgNPs are synthesised with apt size and shape [Citation21]. A study also reported that high pH leads to the formation of large anisotropic nanoparticles with λspr at higher wavelength [Citation22].
Characterisation of Ah-AgNPs
Electron microscopy and elemental analyses
The surface morphology and topography of Ah-AgNPs was examined by FE-SEM analysis which showed that they were spherical in shape with varying size, 10–60 nm present in different aggregations (). The EDAX spectrum exhibited silver as the major element confirming the synthesis of silver nanoparticles as the characteristic signal peak was observed at 0.310 keV (). TEM micrographs predominantly showed spherical shape of Ah-AgNPs (). They occurred in the size range of 10–60 nm thus corroborating with the FE-SEM results. Polydispersity might be due to the presence of various bioactive compounds having different reduction properties which affect AgNPs nucleation and growth.
XRD analysis
The crystalline nature of Ah-AgNPs was determined by XRD analysis. The spectrum showed intense Bragg diffraction peaks at 38.10°, 44.32°, 64.46° and 77.40°, corresponding to hkl values of ˂111>, ˂200>, ˂220> and ˂311> lattice planes, respectively of face centred cubic (FCC) crystal structure of the biosynthesized Ah-AgNPs (). These results are in agreement with the universal Joint Committee on 250 Powder Diffraction Standards file no. 04-0783 verifying crystal shape of AgNPs [Citation23]. The mean crystallite size of Ah-AgNPs was 14.16 nm as determined by Debye-Scherrer's equation considering the width of most intense plane ˂111>:
where K, Scherrer's constant (K = 0.9); λ, wavelength of X-ray radiation (0.15406 nm); β, full-width-at-half-maximum (FWHM) of the corresponding plane (in radians) and θ, diffraction angle].
DLS analysis
DLS analysis determines the hydrodynamic diameter which includes the size of inorganic core along with coating/capping material and the solvent layer interacting with the nanoparticle. It was observed that Ah-AgNPs existed in the size range of 20–50 nm while a few moderately sized nanoparticles (200–500 nm) were also found (). The zeta potential value (surface charge) of nanoparticles was −21.3 mV (Supplementary Figure 1(A,B)) indicating high electrostatic repulsion among the nanoparticles present in suspension. The uniformity in capping could be ascribed to these repulsive forces existing between AgNPs. Surface charge of nanoparticles is an important factor that affects cytotoxicity because of the potential electrostatic interactions between surface of nanoparticles and the cell surface. The high negative potential enhances their stability (preventing agglomeration) and good colloidal nature.
FTIR analysis
FTIR spectrum of A. hispidissima leaf extract and synthesised Ah-AgNPs is depicted in . The absorption peaks observed at 3315.40, 2946.24, 2833.64 cm−1 correspond to –OH stretching of phenols/polyphenols or proteins/enzymes and C–H stretching band whereas the peak at 1630 cm−1 may be due to the stretching vibration of (NH)=O group [Citation24]. The peak formed at 1648.15 and 1449.25 cm−1 correspond to aldehydic stretching vibrations of methyl acetate or conjugated carboxyl and C = C stretching. The peaks at 1119.31 and 1071.90 cm−1 are due to sugar absorption related to alcoholic group of the C–O–C and CH2OH deformation of –OH in phytoconstituents [Citation25]. The bands at 3315.40 and 3151 cm−1 are assigned to –OH stretching of phenols and 1449.25 cm−1 is due to aromatic stretch of –C–N whereas 1017.90 cm−1 corresponds to aromatic stretch of –C–O–C– or –C–O bonds [Citation26]. From the FTIR spectral analysis, it can be anticipated that phenols, proteins, hydroxyl, carboxyl and secondary –OH groups participated in the AgNPs synthesis.
GC-MS analysis
The compounds identified through GC-MS analysis in the leaf extract are listed in Supplementary Table 1, Supplementary Figure 2. Some of the compounds have been reported to have high medicinal potential such as 2-methoxy-4-vinylphenol which is a phenolic compound reported to have anti-oxidant, anti-microbial and anti-inflammatory activities [Citation27]. Similarly, 2-Hydroxyisocaproic acid is reported to be fungicidal against several pathogenic sps. (Candida sp. and Aspergillus sp.) [Citation28] and its anti-inflammatory and anti-microbial activity have also been reported by Nieminen et al. [Citation29]. Also, cis-Vaccenic acid is reported to have anti-inflammatory effects [Citation30]. Guanosine is an intercellular messenger in the central nervous system and it has neuroprotective and neurotrophic effects [Citation31]. Dehydroabietic acid is a naturally occurring diterpene resin acid mainly found in conifers and has anti-microbial, anti-ulcer, cardiovascular activities along with anti-aging effects [Citation32]. Also, n-Hexadecanoic acid is reported to have anti-oxidant, hypocholestrolemic and anti-bacterial activities [Citation33]. Ravi and Krishnan [Citation34] explored the anti-cancer cytotoxic potential of hexadecanoic acid. Thus, the results revealed presence of high medicinal potential of the bioactive compounds found in A. hispidissima leaf extract.
Evaluation of catalytic potential of Ah-AgNPs
To investigate the catalytic activity of biosynthesized AgNPs, the reduction reaction of different dyes was invigorated in the presence of Ah-AgNPs and monitored. Sodium borohydride acts as hydrogen donor to the nanocatalysts, Ah-AgNPs which in turn act as hydrogen carrier for reduction reaction. The process of catalytic dye degradation is thermodynamically favourable but existence of large potential difference between BH4− (donor) and dyes (acceptor) acts as a kinetic barrier decreasing the feasibility of reaction [Citation35]. The reaction in the absence of catalyst was monitored for a week but the absorption maxima did not alter because of the presence of high kinetic barrier between the reciprocally deterring negative ions (BH4−) and organic dyes. Thus, reduction of dyes is absent in the absence of catalyst. The reaction kinetics followed pseudo first order equation and the rate constant 'k' was calculated from the slope of linear part of trace (). Here, Ah-AgNPs acted as catalysts alongwith NaBH4 overcoming the kinetic barrier and led to dye degradation. The MB, MO, TB, SO, EY, CV, MR organic dyes absorption intensity decreased respectively within 30, 20, 80, 50, 240, 120 and 90 s after the addition of Ah-AgNPs. The spectra showing time-dependent reduction/degradation of these dyes in the presence and absence of Ah-AgNPs are shown in Supplementary Figure 3.
Table 1. Rate constant (k) for catalytic degradation of different organic dyes.
The smaller size of biosynthesized Ah-AgNPs having greater surface area and negative potential offered more catalytic sites for the reduction of dyes as it aids the adsorption of dye and BH4−. The nanocatalyst helps in relay of electrons in the redox reaction and transfer surface hydride ions (donor) to the dye (acceptor) [Citation36]. Thus, a similar mechanism might have been followed by the Ah-AgNPs acting as potential catalysts as it was observed that the absorption peak and colour of the dyes vanished completely (). The probable mechanism for catalytic degradation (decolourization) of dyes could be: (i) release of hydrogen by NaBH4 solution which gets adsorbed on catalytic surfaces (ii) adsorption of dyes on the surface of AgNPs activating the nanocatalytic surface (iii) simultaneously, the BH4− ions transfer electrons to AgNPs (iv) a negative charged layer develops around nanoparticles which are then transferred to dye molecules (v) finally the dyes get reduced and are released [Citation13]. These dyes were selected due to their chromophoric nature making it easier to screen their degradation as they become colourless after reduction.
In case of Azo dyes (MR, MO, MB and TB), the azo group (R–N = N–R) reduce to colourless amines (–NH–NH–) by NaBH4 in the presence of Ah-AgNPs. A significant decrease in the absorbance profile and dye colour intensity was observed on the addition of nanoparticles to the reaction mixture. MR is one of the major pollutants found in waste water so its degradation is highly desirable and we observed its degradation within 90 s of reaction in the presence of Ah-AgNPs. Previously, Mohapatra et al. [Citation37] analysed the reductive cleavage of azo bond of MR using Pt nanowires.
Similar observation has been recorded in the case of MO which is an organic sulphosalt dye reduced by reductants like NaBH4 producing non toxic compounds. But the rate of reduction is very slow and the dye is toxic to the environment. Metal nanoparticles with high specific surface area and reactivity accelerate the reduction reaction of such dyes. Also, the layer of bioactive compounds surrounding the surface of AgNPs promote effective adsorption between AgNPs and dye molecules [Citation38]. The spectral band of MO is noted at 460 nm and in the absence of Ah-AgNPs there was no change in absorbance of MO solution. But in their presence, absorbance of MO at 460 nm disappears and a new band was observed at 247 nm which is assigned to hydrazine derivatives. Complete degradation occurred within 20 s of reaction. Few studies reported complete degradation of MO dye leading to production of N, N-dimethyl-benzene-1, 4-diamine and sulfanilic acid [Citation39].
Alongwith these mutagenic dyes, the extent of reduction/degradation of MB was also monitored. It is a heterocyclic aromatic dye widely used in textile industries. The absorption peak of MB monomer in water is centred at 664 nm corresponding to n-π* transition of MB [Citation38]. On addition of colloidal AgNPs, degradation of MB was observed with declining absorbance value approaching baseline which ultimately vanished indicating the participation of AgNPs in electron relay. Similarly, TB is a heterocyclic dye complex which degraded instantly within 80 s of reaction in the presence of Ah-AgNPs.
Further, the degradation of basic dyes such as SO and CV was examined in the presence of synthesised nanocatalysts. Safranine O is a heterocyclic azine group of dyes and is a derivative of phenazine. The absorption band of SO appears at 519 nm and the rate of degradation was visualised by decreasing peak intensity. The photo catalytic degradation of CV was carried out with Ah-AgNPs and was observed in the absorption spectra region at 580–590 nm. It showed complete degradation within 120 s. Also, degradation of an acidic dye, EY was studied. It is a tetrabromofluorescein dye soluble in water and is largely used in textile and paper industry. In the presence of Ah-AgNPs, it took 4 min for complete degradation of EY.
We further extended our study to analyse the decolourization of the mixture of all the dyes tested. The seven dyes were mixed together and treated with NaBH4 and Ah-AgNPs. Interestingly, the mixture of dyes turned colourless within 2 min of reaction (). So it is interpreted that Ah-AgNPs helps in rapid degradation of even the mixture of dyes present in aquatic systems.
From the results obtained, it is clear that the rate of Ah-AgNPs catalysed reaction is very fast. This might be due to the smaller size, spherical shape and crystal structure of nanoparticles which are chemically active and prone to dissolve easily producing catalytically active sites. This results in the availability of large number of binding sites for the chemisorption of reactants thereby enhancing the reaction rate [Citation40].
Sensing of metal ions and ammonia
The Ah-AgNPs were found to be highly sensitive and selective for the detection of Hg2+, Fe3+ ions and NH3. On addition of different metal ions (Na+, K+, Mg2+, Ca2+, Ba2+, As3+, Sn2+, Cu2+, Fe2+, Zn2+, Co2+, Cd2+, Ni2+, Pb2+, Cr3+ and Mn2+) to the AgNPs solution, slight change in absorption spectra was observed but the colour of the solution remained constant. Whereas in the presence of Hg2+, Fe3+ ions and NH3, it was noted that the reaction mixture turned colourless from yellow solution. Alongwith this, quantitative assessment of the selective detection of Hg2+, Fe3+ and NH3 was done using different concentrations of each (Fe3+and Hg2+, 25 − 300 nM; NH3, 0–120 ppm). Fresh colloidal AgNPs solution was taken and the SPR absorbance spectra was observed for all the reactions and recorded (). Due to difference in electrochemical reduction potential, the AgNPs get oxidised to form Ag+ and reduce the Hg2+ ions to Hg0 [Citation6]. With increasing concentration of Hg2+, hypsochromic shift in the SPR spectra was observed which might have occurred due to redox reaction between Ag and Hg2+ ions (Supplementary Figure 4(A)). Similarly, in case of reaction with Fe3+ blue shift in absorbance spectra was observed with increasing ionic concentration (Supplementary Figure 4(B)). Also, ammonia sensing was performed by UV-Vis spectroscopy and it was observed that with increasing concentration from 0 to 30 ppm, the absorbance peak shifted from 416 nm to 394 nm and the absorbance ratio (Abs416/Abs394) increased but at higher concentrations (40–120 ppm), the absorbance ratio Abs416/Abs394 became constant showing saturation at 30 ppm. This indicates that Ah-AgNPs based optical biosensors can actively detect such low concentrations of ammonia in a solution (Supplementary Figure 4(C,D)). Our results are in agreement with earlier reports by other researchers [Citation7,Citation41]. Ammonia sensors have wide application in detecting NH3 in biological, clinical and environmental samples and these silver metal based nanoparticles have proved to be highly sensitive, simple, rapid and cost effective [Citation7]. The Ah-AgNPs sensor can also be used to screen large number of samples contaminated with ferric or mercuric ions.
In vitro anti-oxidant activity of Ah-AgNPs
Total phenolic and flavonoid content of the leaf extract was estimated to be 114.553 ± 10.384 GAE mg g−1 and 203.907 ± 5.884 QE mg g−1. The bioprepared Ah-AgNPs exhibited strong antioxidant potential as 77% DPPH radical scavenging activity was observed at 20 µg/mL which increased approximately upto 94% at all the higher concentrations (). The IC50 values for leaf extract, Ah-AgNPs and quercetin were 58.07, 7.41 and 28.02 µg/mL, respectively. Our results are in agreement with another study where IC50 values for Elephantopus scaber leaf extract and its AgNPs were 73.47 and 6.629 µg/mL, respectively [Citation42]. Further, H2O2 radical scavenging activity of Ah-AgNPs was found to increase with increasing concentration (). The IC50 values for leaf extract, Ah-AgNPs and gallic acid against H2O2 radicals were 54.86, 49.73, 66.93 µg/mL, respectively.
Figure 10. (A) DPPH radical scavenging activity (B) H2O2 radical scavenging activity. The letters a, b, c, d, e on bars indicate significant differences in percent inhibition at different concentrations, as determined by Duncan's multiple range test at p = .05.

Oxidation is a natural process occurring in living cells and leads to the generation of reactive oxygen species (ROS) that can interfere with the normal cell physiological functioning. This imbalance leads to cell damage and can cause oxidative stress, damage to lipids, protein and DNA causing various diseases like arthritis, diabetes, cardiovascular disorder, Alzheimer's disease, cancer, etc. [Citation43]. Antioxidants quench free radicals and it is interpreted that anti-cancer drugs trigger apoptotic cell death in cancer cells by altering oxidant/antioxidant balance. Perhaps the similar mechanism might be occurring with the synthesised Ah-AgNPs having strong anti-oxidant potential which could be used to treat many diseases caused by oxidative stress offering a ray of hope towards the use of AgNPs by medical and pharmaceutical industries.
Evaluation of anti-cancerous activity
MTT assay carried out with Ah-AgNPs and leaf extract have shown differential activity, their IC50 values against HeLa cells being 7.18 µg/mL and 99.07 µg/mL, respectively. A concentration-dependent decrease in percent cell viability of HeLa cells is gleaned from the obtained results (). Incidentally, these Ah-AgNPs being negatively charged with smaller size and shape showed least cytotoxicity with non-malignant L20 B cells. The Ah-AgNPs were found to be much more effective against cervical cancer cell line thereby evoking a new interest in their application as novel biotherapeutics. According to WHO, cervical cancer is the fourth most frequent cancer in women and WHO has prioritised its elimination across the world as it still remains one of the gravest threats to the lives of women. So our study is related to anti-cancer activity of Ah-AgNPs against cervical (HeLa) cancer cells. Bilal et al. [Citation44] also reported that AgNPs loaded chitosan-alginate constructs were completely bicompatible towards L929 (normal cell line) and were cytotoxic against HeLa cells.
Figure 11. Graph depicting effect of (A) AgNPs (2–12 µg/mL) and (B) aqueous leaf extract (20–120 µg/mL) on HeLa cells through MTT assay. The letters a, b, c, d, e on nodes indicate significant differences in percent cell viability in different treatments, as determined by Duncan's multiple range test at p = .05.

Evaluation of anti-microbial activity
The anti-microbial activity of Ah-AgNPs and leaf extract was tested and the diameter of inhibition zones produced by them were compared (; Supplementary Tables 2 and 3). It was found that Ah-AgNPs inhibited all the fungal and bacterial strains more effectively than the leaf extract. This is probably due to the combined action of silver and phytochemical compounds like phenols, flavonoids, proteins etc. constituting the Ah-AgNPs. It is also noted that due to the presence of thicker peptidoglycan layers in cell wall of gram-positive bacteria, they are less prone to AgNPs and Ag+ attack than gram-negative bacteria. The AgNPs disrupt cell membrane, permeabalize the cell wall and enter inside the cells. As the nanoparticles show high surface area to volume ratio, it enhances their area of contact with microbes. The bacterial cell death might have occurred due to accumulation of AgNPs inside the cell membrane and release of cellular compounds. The nanoparticles adhere to cell surface and interact with phosphorus and sulphur moieties present in cell membrane causing metabolism failure, blocks cellular respiration and lead to apoptosis or lysis of bacteria [Citation45]. Release of silver ions increase membrane permeability and subsequently result in loss of proton motive force. It induces de-energisation of cells, causes phosphate efflux, leakage of intracellular ions and reducing sugars and disrupts DNA replication causing cell demise [Citation46]. The use of silver in nanoparticle form is less toxic to cells and has better antibacterial efficiency rather than its ionic form due to the formation of free radicals. Silver nanoparticles have anti-microbial and anti-inflammatory effects and therefore finds tremendous usage in medical industry for mitigating infection in burns and open wounds.
Figure 12. Antimicrobial activity of the Ah-AgNPs against bacteria (a) E. coli (b) K. pneumoniae (c) S. aureus, (d) E. faecalis and fungi (e) Geotrichum candidum (f) C. tropicalis (g) C. albicans. Inhibition zones at different concentrations of Ah-AgNPs, (i) 0.125 mg/mL (ii) 0.25 mg/mL (iii) 0.5 mg/mL (iv) 1 mg/mL, (C) Inhibition zones by standard drug.

The total Ag dissolved in AgNPs suspension was found to be 26.95 ± 0.58 ppm. Though the exact mechanism of anti-microbial activity and toxicity of AgNPs is still not clear, it is assumed that Ag+ ions penetrate in the cell and disturbs H-bonding as it binds with purine and pyrimidine base pairs. It denatures the DNA molecule and causes bacterial cell lysis [Citation47]. The high bactericidal activity might be due to silver cations released from AgNPs which interact with ionic charges of the cell components resulting in enzyme deactivation, impaired cell physiology thereby killing the microbes.
Conclusion
The present study for the first time reports rapid, economically efficient, facile and eco-friendly method for the synthesis of Ah-AgNPs using aqueous leaf extract of Arnebia hispidissima. Such nanoparticles have shown strong anti-oxidant activity, catalytic degradation potential for the tested anthropogenic dye pollutants, excellent selective and sensitive detection of metal ions (Fe3+, Hg2+), ammonia; anti-cancerous and anti-microbial activities. Also, the biogenic Ah-AgNPs developed herein for the first time exhibited significantly high anti-fungal activity against Geotrichum candidum, a fungus known to infect immunocompromised patients. Consequently, such green synthesis of silver metal-based nanoparticles using medicinal plants is pharmacologically and industrially significant and will set a new standard for their large scale synthesis and application in plethora of fields.
Supplementary_Figures-ppt.pptx
Download MS Power Point (3.4 MB)Supplementary_Tables.docx
Download MS Word (23.1 KB)Acknowledgements
Authors acknowledge USIC, DU and SAIF, AIIMS, Delhi for providing instrumentation facilities. Authors are thankful to Dr. Smita Shekhawat, DU; Dr. Sumitra Choudhary and Dr. Ashok Patel, JNVU, Jodhpur for helping in the collection of plant material. SN is grateful to UGC, India for the award of RGNF SRF.
Disclosure statement
No potential conflict of interest was reported by the author(s).
Additional information
Funding
References
- Indana MK, Gangapuram BR, Dadigala R, et al. A novel green synthesis and characterization of silver nanoparticles using gum tragacanth and evaluation of their potential catalytic reduction activities with methylene blue and Congo red dyes. J Anal Sci Technol. 2016;7(1):19.
- Mathur P, Jha S, Ramteke S, et al. Pharmaceutical aspects of silver nanoparticles. Artif Cells Nanomed Biotechnol. 2018;46(sup1):115–126.
- Arya G, Kumari RM, Gupta N, et al. Green synthesis of silver nanoparticles using Prosopis juliflora bark extract: reaction optimization, antimicrobial and catalytic activities. Artif Cells Nanomed Biotechnol. 2018;46(5):985–993.
- Jyoti K, Singh A. Green synthesis of nanostructured silver particles and their catalytic application in dye degradation. J Genet Eng Biotechnol. 2016;14(2):311–317.
- Kirubaharan CJ, Kalpana D, Lee YS, et al. Biomediated silver nanoparticles for the highly selective copper(II) ion sensor applications. Ind Eng Chem Res. 2012;51(21):7441–7446.
- Annadhasan M, Muthukumarasamyvel T, Sankar Babu VR, et al. Green synthesized silver and gold nanoparticles for colorimetric detection of Hg2+, Pb2+, and Mn2+ in aqueous medium. ACS Sustainable Chem Eng. 2014;2(4):887–896.
- Pandey S, Goswami GK, Nanda KK. Green synthesis of biopolymer–silver nanoparticle nanocomposite: an optical sensor for ammonia detection. Int J Biol Macromol. 2012;51(4):583–589.
- Rafique M, Sadaf I, Rafique MS, et al. A review on green synthesis of silver nanoparticles and their applications. Artif Cells Nanomed Biotechnol. 2017;45(7):1272–1291.
- Chang TMS. ARTIFICIAL CELL evolves into nanomedicine, biotherapeutics, blood substitutes, drug delivery, enzyme/gene therapy, cancer therapy, cell/stem cell therapy, nanoparticles, liposomes, bioencapsulation, replicating synthetic cells, cell encapsulation/scaffold, biosorbent/immunosorbent haemoperfusion/plasmapheresis, regenerative medicine, encapsulated microbe, nanobiotechnology, nanotechnology. Artif Cells Nanomed Biotechnol. 2019;47(1):997–1013.
- Shameem N, Kamili AN, Parray JA, et al. Antimicrobial and antioxidant activity of methanol extracts of Arnebia benthamii (Wall ex. G. Don) Johnston—a critically endangered medicinal plant of North western Himalaya. JAST. 2015;6:36–43.
- Jain SC, Jain R, Singh B. Antimicrobial principles from Arnebia hispidissima. Pharm Biol. 2003;41(4):231–233.
- Nakkala JR, Mata R, Raja K, et al. Green synthesized silver nanoparticles: catalytic dye degradation, in vitro anticancer activity and in vivo toxicity in rats. Mater Sci Eng C Mater Biol Appl. 2018;91:372–381.
- Sengan M, Veeramuthu D, Veerappan A. Photosynthesis of silver nanoparticles using Durio zibethinus aqueous extract and its application in catalytic reduction of nitroaromatics, degradation of hazardous dyes and selective colorimetric sensing of mercury ions. Mater Res Bull. 2018;100:386–393.
- Rastogi L, Sashidhar RB, Karunasagar D, et al. Gum kondagogu reduced/stabilized silver nanoparticles as direct colorimetric sensor for the sensitive detection of Hg² in aqueous system. Talanta. 2014;118:111–117.
- Eddine TN, Eddine GN, Eddine L, et al. Antioxidant and antimicrobial activity of flavonoids fraction extract from Arnebia decumbens (Vent) growing in south east Algeria. IJCPR. 2016;7:110–116.
- Kamtekar S, Keer V, Patil V. Estimation of phenolic content, flavonoid content, antioxidant and alpha amylase inhibitory activity of marketed polyherbal formulation. J Appl Pharm Sci. 4(2014):61–65.
- Nindawat S, Agrawal V. Fabrication of silver nanoparticles using Arnebia hispidissima (Lehm.) A. DC. root extract and unravelling their potential biomedical applications. Artif Cells Nanomed Biotechnol. 2019;47(1):166–180.
- Park Y, Noh HJ, Han L, et al. Artemisia capillaris extracts as a green factory for the synthesis of silver nanoparticles with antibacterial activities. J Nanosci Nanotechnol. 2012;12(9):7087–7095.
- Zhang Y, Cheng X, Zhang Y, et al. Biosynthesis of silver nanoparticles at room temperature using aqueous aloe leaf extract and antibacterial properties. Colloids Surf A. 2013;423:63–68.
- Kumar R, Roopan SM, Prabhakarn A, et al. Agricultural waste Annona squamosa peel extract: biosynthesis of silver nanoparticles. Spectrochim Acta A Mol Biomol Spectrosc. 2012b;90:173–176.
- Iravani S, Zolfaghari B. Green synthesis of silver nanoparticles using Pinus eldarica bark extract. Biomed Res Int. 2013;2013:639725.
- Krishnaraj C, Ramachandran R, Mohan K, et al. Optimization for rapid synthesis of silver nanoparticles and its effect on phytopathogenic fungi. Spectrochim Acta A Mol Biomol Spectrosc. 2012;93:95–99.
- He Y, Wei F, Ma Z, et al. Green synthesis of silver nanoparticles using seed extract of Alpinia katsumadai, and their antioxidant, cytotoxicity, and antibacterial activities. RSC Adv. 2017;7(63):39842–39851.
- Shanmugam N, Rajkamal P, Cholan S, et al. Biosynthesis of silver nanoparticles from the marine seaweed Sargassum wightii and their antibacterial activity against some human pathogens. Appl Nanosci. 2014;4(7):881–888.
- Kumar B, Smita K, Cumbal L, et al. One pot phytosynthesis of gold nanoparticles using Genipa americana fruit extract and its biological applications. Mater Sci Eng C Mater Biol Appl. 2016;62:725–731.
- Rasheed T, Bilal M, Iqbal HM, et al. Green biosynthesis of silver nanoparticles using leaves extract of Artemisia vulgaris and their potential biomedical applications. Colloids Surf B Biointerfaces. 2017;158:408–415.
- Ravikumar VR, Gopal V, Sudha T. Analysis of phytochemical constituents of stem bark extracts of Zanthoxylum tetraspermum Wight & Arn. Res J Pharm Biol Chem Sci. 2012;3:391–402.
- Sakko M, Moore C, Novak L, ‐et al. 2-hydroxyisocaproic acid is fungicidal for Candida and Aspergillus species. Mycoses. 2014;57(4):214–221.
- Nieminen MT, Hernandez M, Novak-Frazer L, et al. DL-2-hydroxyisocaproic acid attenuates inflammatory responses in a murine Candida albicans biofilm model. Clin Vaccine Immunol. 2014;21(9):1240–1245.
- Hussein HM, Hameed IH, Ibraheem OA. Antimicrobial activity and spectral chemical analysis of methanolic leaves extract of Adiantum capillus-veneris using GC-MS and FT-IR spectroscopy. IJPPR. 2016;8:369–385.
- Lanznaster D, Dal-Cim T, Piermartiri TC, et al. Guanosine: a neuromodulator with therapeutic potential in brain disorders. Aging Dis. 2016;7(5):657–679.
- Kim J, Kang YG, Lee JY, et al. The natural phytochemical dehydroabietic acid is an anti-aging reagent that mediates the direct activation of SIRT1. Mol Cell Endocrinol. 2015;412:216–225.
- Abubakar M, Majinda R. GC-MS analysis and preliminary antimicrobial activity of Albizia adianthifolia (Schumach) and Pterocarpus angolensis (DC). Medicines. 2016;3(1):3.
- Ravi L, Krishnan K. Cytotoxic potential of N-hexadecanoic acid extracted from Kigelia pinnata leaves. Asian J Cell Biol. 2016;12(1):20–27.
- Laoufi I, Saint-Lager MC, Lazzari R, et al. Size and catalytic activity of supported gold nanoparticles: an in operando study during CO oxidation. J Phys Chem C. 2011;115(11):4673–4679.
- Nadaf NY, Kanase SS. Biosynthesis of gold nanoparticles by Bacillus marisflavi and its potential in catalytic dye degradation. Arab J Chem. 2016;12(8):4806–4814.
- Mohapatra SK, Sonavane SU, Jayaram RV, et al. Regio- and chemoselective catalytic transfer hydrogenation of aromatic nitro and carbonyl as well as reductive cleavage of azo compounds over novel mesoporous NiMCM-41 molecular sieves. Org Lett. 2002;4(24):4297–4300.
- Vidhu VK, Philip D. Catalytic degradation of organic dyes using biosynthesized silver nanoparticles. Micron. 2014;56:54–62.
- Rasheed T, Bilal M, Li C, et al. Catalytic potential of bio-synthesized silver nanoparticles using Convolvulus arvensis extract for the degradation of environmental pollutants. J Photochem Photobiol B Biol. 2018;181:44–52.
- Vijayan R, Joseph S, Mathew B. Indigofera tinctoria leaf extract mediated green synthesis of silver and gold nanoparticles and assessment of their anticancer, antimicrobial, antioxidant and catalytic properties. Artif Cells Nanomed Biotechnol. 2018;46(4):861–871.
- Dubas ST, Pimpan V. Green synthesis of silver nanoparticles for ammonia sensing. Talanta. 2008;76(1):29–33.
- Francis S, Joseph S, Koshy EP, et al. Microwave assisted green synthesis of silver nanoparticles using leaf extract of elephantopus scaber and its environmental and biological applications. Artif Cells Nanomed Biotechnol. 2018;46(4):795–804.
- Khalil AT, Ovais M, Ullah I, et al. Sageretia thea (Osbeck.) modulated biosynthesis of NiO nanoparticles and their in vitro pharmacognostic, antioxidant and cytotoxic potential. Artif Cells Nanomed Biotechnol. 2018;46(4):838–852.
- Bilal M, Rasheed T, Iqbal HM, et al. Development of silver nanoparticles loaded chitosan-alginate constructs with biomedical potentialities. Int J Biol Macromol. 2017;105(Pt 1):393–400.
- He Y, Li X, Zheng Y, et al. A green approach for synthesizing silver nanoparticles, and their antibacterial and cytotoxic activities. New J Chem. 2018;42(4):2882–2888.
- Marambio-Jones C, Hoek EM. A review of the antibacterial effects of silver nanomaterials and potential implications for human health and the environment. J Nanopart Res. 2010;12(5):1531–1551.
- Bilal M, Rasheed T, Iqbal HMN, et al. Silver nanoparticles: biosynthesis and antimicrobial potentialities. International J of Pharmacology. 2017;13(7):832–845.