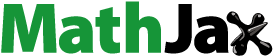
Abstract
Cancer is a global epidemic disease responsible for over ten millions death worldwide. The early diagnosis and the precise treatment with reduced adverse reactions are the main goal worldwide. In this study, we produced, characterized and evaluated (in vitro) in three different cancer cell lines (protaste, breast and melanoma) a radioactive gold nanocluster (R-AuNC) (198Au25(Capt)18). The pharmacokinetics as the influence in the ABC transporter (MRP1 Efflux Transporter Protein) was also evaluated. The results showed that R-AuNC (198Au25(Capt)18) are capable to kill the cancer cells lines of protaste, breast and melanoma. The pharmacokinetics showed a fast clearance and great volume of distribution, confirming the use of R-AuNC as nanomedicine for cancer treatment. Finally, the ABC transporter assay corroborated that the R-AuNC (198Au25(Capt)18) has no risk of being pumped out of cells by this efflux transporter. The results validate the use of gold nanoparticles as therapeutic nanomedicine for cancer treatment.
Graphical Abstract

Introduction
Several nanoparticles such as quantum dots, liposomes, polymeric nanoparticles, carbon nanotubes, metal nanoparticles, dendrimers and inorganic nanoparticles have been investigated in preclinical and clinical studies for therapy, imaging and combination of both as theranostic agent [Citation1–5]. Among them, gold-based nanoparticles, including gold nanocluster (AuNC) have gained relevance in recent years [Citation6–9]. AuNCs consist of a hybrid compound with inorganic metal and organic binders. They are about 2–3 nm in size, representing the core diameter of the metal cluster.
The AuNCs are organized in a unique structure ranging from 10 to 100 atoms. In addition to these structural characteristics, the physico-chemical characteristics of these AuNCs represent HOMO-LUMO electron transition, luminescence, large Stokes shift, catalytic reactivity, chirality, magnetism and photovoltaic properties [Citation8]. Moreover, the AuNCs have several advantages for its use in biomedical applications including high thermal conductivity, great optical stability, visible fluorescence (700 nm), high stability, low toxicity and low immune response [Citation10,Citation11]. All these characteristics of AuNCs signify its application in imaging [Citation12,Citation13] and therapy [Citation12,Citation14,Citation15].
The AuNC optical properties are directly related to its size, structure, presence of binders, surface charge, temperature and pH [Citation8,Citation11,Citation16]. The literature describes that its size is compatible with the wavelength of the Fermi electron (0.5 nm for gold), which demonstrates a strong quantum confinement of the free electrons with discrete electronic states; revealing molecular and non-atomic characteristics [Citation11,Citation17,Citation18]. As these electrons behave with molecular type transitions, a characteristic of metals surface plasmon resonance is not observed [Citation8,Citation11]. The fact that AuNCs are well-defined nanostructures allows surface modification, with the insertion of many functional groups including drugs, proteins and molecules [Citation8,Citation9,Citation11,Citation19]. This capacity promotes the use of AuCNs as efficient targeting system. In addition, the exhibition of fluorescence in a broad region (visible to near-infrared) makes AuNCs strong candidates to be used as imaging probes [Citation7,Citation12,Citation15,Citation20,Citation21].
Recently, an increasing interest in the use of radioactive material as nanocompounds in medicine is rising. In this regard, the use of radioactive AuNCs (R-AuNCs) emerges as promising alternative.
The R-AuNCs may be produced by direct irradiation of non-radioactive (metallic AuNCs). In this type of reaction, metallic gold ) is irradiated with thermal neutrons in order to produce the well-known radioisotope of gold (
). The production of radioactive gold from the metallic gold is defined by the equation:
The radioisotope of gold () has several interesting physical, chemical-physical and biological characteristics [Citation22–24] as shown in , which allows it use for therapeutic purposes.
Table 1. Properties of the gold radioisotope ().
The most abundant gamma ray (Eβ =0.412 MeV; Iγ = 95.6%) emitted by the is suitable to be used for radionuclide therapy. It is important to notice that for diagnostic purposes the gamma ray emitted by the therapeutic radionuclide must be composed of photons and comprised from 75 to 250 KeV and should have short half-life which is not the case in
Therefore, this radionuclide is not suitable for SPECT or PET imaging but a potential radiopharmaceutical for radionuclide therapy.
The has great therapeutic properties due to the emission of Auger and Coster–Kronig electrons because these electrons are capable to produce multiple ionizations (LET: 4–26 keV/µm) in the immediate vicinity (few nanometers) of the decay site. This multiple ionization can lead radiosensitive cells’ death and increased effect on localized radiotherapy. Also
(T1/2=2.7 d) emits mean beta energy (β−=312.5 keV; Iβ =100%) which is somehow similar to the 90Y (T1/2=2.67 d) a daily routine therapeutic beta (β−=933.6 keV; Iβ =100%) radiopharmaceuticals for the treatment of cancer. However, it emits higher energy than 177Lu (T1/2=6.6 d) with mean beta energy (β−=133.6 keV; Iβ =100%) and lower than 166Ho (T1/2=1.12 d) with mean beta energy (β−=665 keV; Iβ =100%) that are also used in nuclear medicine as routine radiopharmaceuticals [Citation25–27].
The R-AuNCs have the ability to spread, and re-emit the radiation, providing applicability in cancer therapy, for example by locally accumulating the dose of radiation into cancerous tissues [Citation7,Citation12,Citation15], due to the enhanced permeation and retention effect (EPR) of AuNCs at the tumour site [Citation20,Citation21]. Is important to notice however that the radiation dose delivered by R-AuNCs is estimated to be 100% higher compared with regular radioactive gold; considering tumour/healthy tissues correlation [Citation28], as consequence a 100% higher transference of energy from the R-AuNC to the tumour tissue is expected; promoting a higher damage with higher formation of free radical and ionization of molecules such as DNA [Citation12,Citation14], promoting cell death.
In this study, we produced and evaluated the 198Au25(Capt)18 nanoclusters as nanoradiopharmaceutical for therapeutic application due to its high water-soluble property, thermal stability, innovative one-step synthesis’s route, high fluorescence centred at ∼700 nm and chiroptical features given by the captopril ligand.
Materials and methods
Reactor irradiation
The samples of 197Au nanoclusters (197Au25(Capt)18) were irradiated at the Argonauta (Potency of 340 W) reactor installed in Nuclear Engineering Institute (Brazil). The sample was irradiated for 4 h using a thermal neutron flux of 3.2 × 109 n.cm−2.s−1 with average thermal neutrons energy of 0.0025 eV. The thermal activation microscopic cross-section was 98.5 ± 0.4 barns. It resulted the production of 4.04 × 107 atoms.s−1.g−1 of radioactive atoms.
Measure of the radioactivity
The induced activity of the was determined by gamma spectrometry system having 6.2 cm diameter, 4 cm height, 41.1 cm3 active volume and 30% detection efficiency; coupled to Canberra multi-channel analyser (MCA) with 8192 channels. This system presented a resolution of 1.8 keV in 1332 keV for 60Co. To reduce the background effect, the detector was shielded with ∼10 cm lead. The measurement time for each sample was standardized at 3600 s (1 h).
The detection efficiency
The detection efficiency for each energy was determined using a Laboratory SOurceless Calibration Software (LabSOCS) from Canberra. To this end, it was necessary to design the geometry used in computational environment entering the physical, chemical and geometric characteristics of both pot and sample. After geometrical modelling, the software divides the entire source region (pot and sample) into 1024 voxels, and randomly chooses a point in each voxel and calculates detection efficiency from the values found. Then, the software doubles the number of voxels and repeats the entire process, and obeying convergence criteria compares the values. If the simulated efficiency values do not converge, the number of voxels is doubled again until a satisfactory convergence is obtained.
Synthesis Au25 nanoclusters Captopril
Briefly, HAuCl43H2O (0.20 mmol, 78.7 mg) and TOABr (0.23 mmol, 126.8 mg) were dissolved in 10 ml methanol and vigorously stirred as described by Kumar and Jin [Citation29]. After 20 min, captopril (1 mmol, 217.2 mg, dissolved in 5 ml methanol) was introduced into the reaction mixture under stirring. Then after 30 min, NaBH4 (2 mmol, 75.6 mg dissolved in 5 ml of ice cold water) was added to the reaction mixture under stirring. The reaction was allowed to proceed for 8 h and then the reaction mixture was centrifuged to remove unreacted insoluble Au(I):SR polymers. The supernatant was collected and concentrated by rotary evaporation. The clusters were precipitated by adding ethanol to the solution. Then precipitates were extracted with minimum amounts of methanol. The process was repeated several times. The cluster solution was precipitated again by ethanol and finally dried under vacuum.
Characterization
The analysis was done in order to characterize the AuNCs (198Au25(Capt)18). The analysis of thermal stability (HP8453 spectrometer equipped with an Agilent 89,090A temperature-programmed module), fluorescence spectra (Fluorolog-3 spectrofluorometer, HORIBA Jobin Yvon), electrospray ionization (ESI) mass spectra (Waters Q-TOF mass spectrometer equipped with a Z spray source), thermogravimetric analysis (TGA) (TG/DTA6300 analyser Seiko Instruments, Inc., Tokyo, Japan), nuclear magnetic resonance (NMR) spectra (Bruker Avance_ 300 spectrometer, Billerica, MA, USA) and circular dichroism (CD) spectra of Au25 clusters (JASCO J-810 CD spectrometer) was carried out. All these data are included as supplemental file since this synthesis has been reported (published) previously.
Photoluminescence analysis
The photoluminescence spectra were obtained using a Horiba-Jobin-Yvon spectrometer (model T64000). The instrument was set up in single-mode with a 300 grooves/mm holographic diffraction grating and a liquid-N2-cooled charge-coupled device (CCD) detector. For excitation, a NUV 405.0 nm line was employed. The light was focussed on the sample using a microscope BX41 with an SLMPLN 20x objective lens (WD = 26.5 mm) of both Olympus. The obtained spectra resulted from 3 accumulations of 1 s each.
Human cells cultures
Human cell lines from melanoma (MV3), breast cancer (MDA-MB-231) and prostate cancer (PC-3) were obtained from Cell Bank of Rio de Janeiro (Rio de Janeiro, Brazil). These cells were routinely maintained (separately) in DMEM (MV3, MDA-MB-231 and HEK293T) or in RPMI medium (PC-3) supplemented with 10% FBS, NaHCO3 (3.7 g/l), HEPES (5.2 g/l), penicillin (0.5 U/ml) and streptomycin (0.5 mg/ml) [Citation30].
Proliferation assay
Human tumour cells (MV3, MDA-MB-231 and PC-3) at concentration 5 × 103 cells/well, were seeded in 96-well plates and allowed to attach for 24 h. Then, cells were treated in presence or absence of 198Au nanoclusters (2 and 4 Gy) and paclitaxel was used as positive control (10, 50 and 100 nm) for another 24 h. After washing, the number of attached cells was calculated based on a standard curve made by seeding 1 × 103 to 5 × 103 by the MTT assay as previously described by Nunes et al. [Citation31]
Statistical analysis
Statistical analysis of data was performed using the Graph-Pad Prism 5.0 software (GraphPad Software, San Diego, CA, USA). Differences between means from two groups were compared by the one-way ANOVA test and confirmed by Bonferroni post-test. The values of *p<.05, **p<.01, ***p<.005 and *****p<.0001 were considered statistically significant.
Pharmacokinetic
The pharmacokinetics parameters: concentration at zero time, elimination constant (K), volume of distribution, elimination half-life and clearance were calculated [Citation32]. To perform the PK analysis, 2 µg of 198Au nanoclusters (Au25(Capt)1) with an activity of 50 µCi were injected by retro-orbital via into healthy Wistar rats (n = 3). Then, at time of 1, 3, 6 and 24 h, 10 µl of blood from tail vein were collected. The conversion of radioactive counting to mass of 198Au nanocluster was calculated considering the initial mass (2 µg) administered as the initial activity of 50 µCi using the radioactive decay formula:
MRP1 efflux transporter protein activity
Efflux transport activity of MRP1 was measured using doxorubicin accumulation assay. This assay was performed using confocal microscopy. Sterilized cover glasses were placed in 6-well plate and covered with 0.1 mg/ml of poly-D-lysine for 10 min prior to washing with phosphate buffered saline (PBS). HEK293T cells were plated on poly-D-lysine-coated cover glass at a density of 5 × 105 cells/well in 2 ml culture medium. Cells were transiently transfected with 2 µg of an MRP1-GFP expression vector after 24 h using 4 µg of jet PRIME Transfection Reagent with 200 µl of Transfection buffer (Polyplus-transfection SA, Illkirch, France). After 48 h, cells were pre-treated with 0.1, 0.25, 0.5, and 1% of Au nanoclusters for 30 min before incubation with doxorubicin (10 µM) for 1 h. Cells were maintained in buffer (4.5% glucose, 10 mM HEPES, PBS containing Ca2+ and Mg2+) and intracellular fluorescence was visualized using a iMIC digital microscope (TILL Photonics GmbH, Gräfelfing, Germany) equipped with a 1.35 numerical aperture 60× oil-immersion objective. Excitation was done at 488 nm for GFP and doxorubicin with emission bands of 475/42 and 605/64 nm respectively. Images were processed using ImageJ (NIH, Bethesda, MD).
Results and discussion
Reactor irradiation
The irradiation was carried out at the established conditions to produce 198Au nanoclusters. The gamma spectrum taken with HPGe shows the specific gamma (412 KeV) from the 198Au as given in .
Measure of the radioactivity
The radioactivity in the final solution of 198Au25(Capt)18 AuNC was 5 × 106 Bq or 1.35 × 10−4 Ci (135 µCi) as measured with HPGe that affirms the transformation of AuNCs to R-AuNCs. This radioactive activity was sufficient to perform the MTT assay.
Photoluminescence analysis
Photoluminescence analysis was performed to observe whether the neutron irradiation to produce the R-AuNCs (198Au25(Capt)18) had affected the stability and/or the properties of the AuNCs or not. The visual inspection () shows no changes in colour or any visual aspect whereas photoluminescence analysis () shows a slightly dislocation of the luminescence counts as the wavelength shifts towards right. There is no explanation in literature to justify the influence of neutron on atomic states of AuNCs and the difference between the irradiated and non-irradiated sample. We believe that the anionic Au25 would slowly be changed to neutral Au25 [Citation43].
Cytotoxicity
The cytotoxicity assay showed that in both concentrations (2 and 4 Gy), the 198Au-Nanocluster (198Au25(Capt)18) was capable to show a considerable cell death behaviour in three different cancer cell lines (). The law governing the effect of radiation on cell proliferation/death is the Bergonié and Tribondeau Law. According to this law, cells that are under intense division rate are more radiosensitive. Thus it is concluded that the most radiosensitive cell line is breast cancer (MDA-MB-231) followed by melanoma cancer (MV3) and prostate cancer (PC3). This can be explained by the fact that an immature tumour is more aggressive than the more differentiated counterpart [Citation33]. In all the cases, the three cell lines used are very aggressive cell lines [Citation34–36]. Thus the difference in behaviour, when exposed to beta radiation, is possibly due an intrinsic factor of each cell line including resistance and internalization. The results show significant response due to radiation dose and reduction of cell viability in vitro for all three human cancer cell lines. Is also possible to observe that the AuNCs showed better results in all cell lines when compared with low (10 nm) and middle dose of paclitaxel (50 nm). In the case of higher doses of paclitaxel (100 nm) the results were very similar in all cancer cell lines, corroborating the efficacy of R- AuNCs.
Pharmacokinetic
Pharmacokinetic analysis was performed using non-compartmental analysis of 198Au-Nanocluster (198Au25(Capt)18). The analysis was performed collecting blood samples of three healthy rats (Wistar rat) at four different times (1, 3, 8 and 24 h) after single retro-orbital bolus administration (20 µg) with a total activity of 50 µCi. The volume of distribution (Vd) which estimates the distribution into extravascular tissues (tissue deposition) [Citation37] was 0.00442 mg/l. This indicates that for the analysed period, high amount of 198AuNC remains in plasma while is poorly distributed into the body. The clearance (CL) that is an essential value to establish the dose regime, shows that the elimination rate 0.32 mg/l/h [Citation38] which means the fast elimination of 198AuNC (198Au25(Capt)18). Finally, the elimination half-life (T1/2) which represents the time to eliminate 50% of the 198Au-Nanocluster from plasma was measured that is 0.906 h ratifying the fast clearance as reported by Zhang et al. [Citation7]
Interaction of Au nanoparticles with MRP1 efflux transporter protein
MRP1, an ATP-binding cassette (ABC) integral membrane transporter protein, mediates the ATP-dependent efflux of a wide variety of drugs, drug-conjugates, toxic molecules and various types of endogenous and exogenous chemical compounds including nanoparticles [Citation39–41]. Therefore, MRP1 can significantly reduce the efficacy of many different types of drugs and therapeutic agents. As MRP1 is known to have moderate to high expression in lung, breast and prostate tissues and plays an important role in tissue defence [Citation42], we investigated the interaction of AuNCs (Au25(Capt)18) with this efflux transporter protein. MRP1 transport activity was evaluated by detecting the accumulation of the fluorescent anticancer drug, doxorubicin and a well-known substrate of MRP1. HEK293T cells were transiently transfected with MRP1-GFP vector and confocal microscopy was used to visualize the effect of various concentrations (0.1, 0.25, 0.5, and 1%) of the Au nanoclusters (Au25(Capt)18) on MRP1-mediated doxorubicin efflux in live cells. As shown in , cells treated with DMSO (vehicle) showed high doxorubicin accumulation in the nuclei of non-transfected cells while doxorubicin fluorescence was very low or undetectable in cells expressing GFP-tagged MRP1 (MRP1-GFP). MRP1-mediated efflux of doxorubicin was blocked by MK571 treatment (50 µM), a commonly used inhibitor of MRP1 transport activity. If Au nanoclusters were substrates of MRP1 then they will be expected to decrease the efflux of doxorubicin by MRP1 due to competitive inhibition. As shown in , none of the tested concentrations of Au nanoclusters demonstrated MRP1 inhibition. These results indicate that Au nanoclusters did not interfere with the doxorubicin efflux by MRP1. We also tested the effects of various concentrations (0.1, 0.25, 0.5, and 1%) of the Au nanoclusters on MRP1-mediated efflux of calcein dye (another commonly used MRP1 substrate) in live cells. As observed in case of doxorubicin, Au nanoclusters did not interfere with the efflux activity of calcein dye by MRP1 (results not shown). Overall the results indicate that Au nanoclusters are likely not substrates of MRP1 and therefore are not at risk of being pumped out of cells by this efflux transporter. The ABC transporter protein such as MRP1 is frequently upregulated in various types of cancers leading to the failure of chemotherapy. For successful cancer treatment, it is desirable to use drugs and nanomaterials that are not substrates of this efflux transporter protein. Is important to notice however that more studies, using more advanced techniques as inductively coupled plasma mass spectrometry (ICP-MS) must be conducted in order to undoubtedly confirm the interaction between AuNC and MPR1 efflux.
Figure 4. Effect of Au nanoclusters (Au25(Capt)18) on MRP1 efflux activity. Doxorubicin accumulation assay was used to measure MRP1 efflux activity. HEK293T cells transiently transfected with MRP1-GFP (green) were pre-treated with 0.1, 0.25, 0.5, 1% Au nanoclusters or 50 μM of MK571 (known MRP1 inhibitor), before incubation with doxorubicin (red) at 37 °C for 1 h. Images were acquired using confocal microscopy. GFP and doxorubicin were excited at 488 nm, and emission detected at 475/42 and 605/64 nm, respectively.

Conclusion
The results represent the efficacy of 198Au25(Capt)18 as therapeutic nanodrug. Also, the pharmacokinetics and the ABC transporter results confirmed the quality of gold nanoparticles. Is important to notice that a high renal clearance has been observed however, more studies should be performed in order to increase this parameter.
Acknowledgments
Dr Rongchao Jin from the Carnegie Mellon University, Chemistry, 4400 Fifth Ave, 15213, Pittsburgh, USA, who gently donates the Gold Nanocluster. The authors thank to CNPq and CAPES. All the raw data is under consideration in a patent. No support was received from any pharmaceutical or biopharmaceutical company for the production of this manuscript. The manuscript is a sole research study.
Disclosure statement
No potential conflict of interest was reported by the author(s).
References
- Daraee H, Eatemadi A, Abbasi E, et al. Application of gold nanoparticles in biomedical and drug delivery. Artif Cells Nanomed Biotechnol. 2016;44(1):410–422.
- Wolfram J, Zhu M, Yang Y, et al. Safety of nanoparticles in medicine. Curr Drug Targets. 2015;16(14):1671–1681.
- Andreou C, Pal S, Rotter L, et al. Molecular imaging in nanotechnology and theranostics. Mol Imaging Biol. 2017;19(3):363–372.
- Jahangirian H, Lemraski EG, Webster TJ, et al. A review of drug delivery systems based on nanotechnology and green chemistry: green nanomedicine. Int J Nanomedicine. 2017;12:2957–2978.
- Karaman DŞ, Sarparanta MP, Rosenholm JM, et al. Multimodality imaging of silica and silicon materials in vivo. Adv Mater. 2018;30(24):1703651.
- Zheng K, Setyawati MI, Leong DT, et al. Antimicrobial gold nanoclusters. ACS Nano. 2017;11(7):6904–6910.
- Zhang XD, Luo Z, Chen J, et al. Ultrasmall glutathione-protected gold nanoclusters as next generation radiotherapy sensitizers with high tumor uptake and high renal clearance. Sci Rep. 2015;5:1–7.
- Jin R. Atomically precise metal nanoclusters: stable sizes and optical properties. Nanoscale. 2015;7(5):1549–1565.
- Negishi Y, Chaki NK, Shichibu Y, et al. Origin of magic stability of thiolated gold clusters: a case study on Au25(SC6H13)18. J Am Chem Soc. 2007;129(37):11322–11323.
- Purohit R, Singh S. Fluorescent gold nanoclusters for efficient cancer cell targeting. Int J Nanomed. 2018;13:15–17.
- Chen LY, Wang CW, Yuan Z, et al. Fluorescent gold nanoclusters: recent advances in sensing and imaging. Anal Chem. 2015;87(1):216–229.
- Zhou F, Feng B, Yu H, et al. Cisplatin prodrug-conjugated gold nanocluster for fluorescence imaging and targeted therapy of the breast cancer. Theranostics. 2016;6(5):679–687.
- Liang G, Xiao L. Gd3+-Functionalized gold nanoclusters for fluorescence-magnetic resonance bimodal imaging. Biomater Sci. 2017;5(10):2122–2130.
- Liang G, Jin X, Zhang S, et al. RGD peptide-modified fluorescent gold nanoclusters as highly efficient tumor-targeted radiotherapy sensitizers. Biomaterials. 2017;144:95–104.
- Zhang XD, Luo Z, Chen J, et al. Ultrasmall Au(10-12)(SG)(10-12) nanomolecules for high tumor specificity and cancer radiotherapy. Adv Mater Weinheim. 2014;26(26):4565–4568.
- Riaz N, Wolden SL, Gelblum DY, et al. HHS public access. 2016;118(24):6072–6078.
- Chen S, Ingram RS, Hostetler MJ, et al. Gold nanoelectrodes of varied size: transition to molecule-like charging. Science. 1998;280(5372):2098–2101.
- Zheng J, Nicovich PR, Dickson RM. Highly fluorescent noble-metal quantum dots. Annu Rev Phys Chem. 2007;58(1):409–431.
- Matulionyte M, Dapkute D, Budenaite L, et al. Photoluminescent gold nanoclusters in cancer cells: cellular uptake, toxicity, and generation of reactive oxygen species. Int J Mol Sci. 2017;18(2):1–17.
- Drude N, Tienken L, Mottaghy FM. Theranostic and nanotheranostic probes in nuclear medicine. Methods. 2017;130:14–22.
- Cooper DL, Conder CM, Harirforoosh S. Nanoparticles in drug delivery: mechanism of action, formulation and clinical application towards reduction in drug-associated nephrotoxicity. Expert Opin Drug Deliv. 2014;11(10):1661–1680.
- Kato H. Production of gold-198 grains. Gold Bull. 1978;11(3):86–87.
- Hosseini SF, Sadeghi M, Aboudzadeh MR, et al. Production and modeling of radioactive gold nanoparticles in Tehran research reactor. Appl Radiat Isot. 2016;118:361–365.
- Volkert WA, Goeckeler WF, Ehrhardt GJ, et al. Therapeutic radionuclides: production and decay property considerations. J Nuclear Med. 1991;32:147–185.
- Müller C, van der Meulen NP, Benešová M, et al. Therapeutic radiometals beyond 177Lu and 90Y: production and application of promising α-particle, β-particle, and auger electron emitters. J Nucl Med. 2017; 58( 2):91S–96S.
- Kassis AI. Therapeutic radionuclides: biophysical and radiobiologic principles. Semin Nucl Med. 2008;38(5):358–366.
- Sgouros G. Radiopharmaceutical therapy. Health Phys. 2019;116(2):175–178.
- Sainz V, Conniot J, Matos AI, et al. Regulatory aspects on nanomedicines. Biochem Biophys Res Commun. 2015;468(3):504–510.
- Kumar S, Jin R. Water-soluble Au25(Capt)18 nanoclusters: synthesis, thermal stability, and optical properties. Nanoscale. 2012;4(14):4222–4227.
- Helal-Neto E, de Barros AOS, Saldanha-Gama R, et al. Molecular and cellular risk assessment of healthy human cells and cancer human cells exposed to nanoparticles. Int J Mol Sci. 2020;21:230.
- Nunes SS, Outeiro-Bernstein MAFD, Juliano L, et al. Syndecan-4 contributes to endothelial tubulogenesis through interactions with two motifs inside the pro-angiogenic N-terminal domain of thrombospondin-1. J Cell Physiol. 2008;214(3):828–837.
- Moia VM, Leal Portilho F, Almeida Pádua T, et al. Lycopene used as anti-inflammatory nanodrug for the treatment of rheumathoid arthritis: animal assay, pharmacokinetics, ABC transporter and tissue deposition. Colloids Surf B Biointerfaces. 2020;188:110814.
- Jögi A, Vaapil M, Johansson M, et al. Cancer cell differentiation heterogeneity and aggressive behavior in solid tumors. Ups J Med Sci. 2012;117(2):217–224.
- Friedl P, Maaser K, Klein CE, et al. Migration of highly aggressive MV3 melanoma cells in 3-dimensional collagen lattices results in local matrix reorganization and shedding of alpha2 and beta1 integrins and CD44. Cancer Res. 1997;57(10):2061–2070.
- Tai S, Sun Y, Squires JM, et al. PC3 is a cell line characteristic of prostatic small cell carcinoma. Prostate. 2011;71(15):1668–1679.
- Neve RM, Chin K, Fridlyand J, et al. A collection of breast cancer cell lines for the study of functionally distinct cancer subtypes. Cancer Cell. 2006;10(6):515–527.
- Verrotti A, Iapadre G, Di Donato G, et al. Pharmacokinetic considerations for anti-epileptic drugs in children. Expert Opin Drug Metab Toxicol. 2019;15:199–211.
- Gaudinski MR, Coates EE, Novik L, et al. Safety, tolerability, pharmacokinetics, and immunogenicity of the therapeutic monoclonal antibody mAb114 targeting Ebola virus glycoprotein (VRC 608): an open-label phase 1 study. Lancet. 2019;393(1074): 889–898.
- Iram SH, Gruber SJ, Raguimova ON, et al. ATP-binding cassette transporter structure changes detected by intramolecular fluorescence energy transfer for high-throughput screening. Mol Pharmacol. 2015;(1):84–94.
- Iram SH, Cole SP. Differential functional rescue of Lys(513) and Lys(516) processing mutants of MRP1 (ABCC1) by chemical chaperones reveals different domain-domain interactions of the transporter. Biochim Biophys Acta. 2014;1838(3):756–765.
- Peterson BG, Tan KW, Osa-Andrews B, et al. High-content screening of clinically tested anticancer drugs identifies novel inhibitors of human MRP1 (ABCC1). Pharmacol Res. 2017;119:313–326.
- Tan KW, Osa-Andrews B, Sampson A, et al. Calcitriol and calcipotriol modulate transport activity of ABC transporters and exhibit selective cytotoxicity in MRP1-overexpressing cells. Drug Metab Disposit. 2018;(12):1856–1866.
- Wu Z, Jin R. On the ligand’s role in the fluorescence of gold nanoclusters. Nano Lett. 2010;10(7):2568–2573.