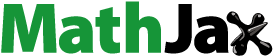
Abstract
Osteoporosis is a common disease among the ageing society. Oxidative stress caused by excessive accumulation of reactive oxygen species (ROS) is the aetiology of osteoporosis. α-Lipoic acid (ALA) is an antioxidant in the body, which can eliminate excess ROS in the body and inhibits levels of oxidative stress in cells. Herein, we designed PEGylated hollow gold nanoparticles (HGNPs) loaded with ALA (mPEG@HGNPs-ALA) to remove ROS in the treatment of osteoporosis. First, mPEG@HGNPs with a particle size of ∼63 nm has been successfully synthesized. By comparing the drug loading of mPEG@HGNPs, it was concluded that the optimal mass ratio of mPEG@HGNPs (calculated by the amount of gold) to ALA was ∼1:2. ABTS antioxidant assay showed that free radical removal ability. In vitro results revealed that the preparation had good biocompatibility. At the gold concentration of 1–150 μg/mL, the cell viability of mPEG@HGNPs was more than 100%, which indicated that it could promote the proliferation of osteoblasts. What’s more, mPEG@HGNPs-ALA could effectively remove the ROS caused by H2O2 injury and improve the cell viability. According to these results, it can be considered that mPEG@HGNPs-ALA has the potential to treat osteoporosis.
Introduction
Osteoporosis is a common disease among middle-aged and elderly people. With the ageing of the population, osteoporosis has become a common disease in the world [Citation1]. Osteoporosis is a bone disease characterized by low bone mass and deterioration of bone microstructure [Citation2], resulting in reduced bone strength and an increased risk of fracture. Reactive oxygen species (ROS) can regulate the differentiation of osteoclasts, and osteoclasts digest bone matrix by dissolving minerals in bone and secreting protease, which eventually leads to osteoporosis [Citation3]. Oxidative stress is caused by the accumulation of excessive ROS in the body, which means that the production of ROS exceeds the antioxidant capacity of the body [Citation4]. The methods for the treatment of osteoporosis can be divided into three categories according to their mechanism of action, namely inhibiting bone resorption, promoting bone formation and promoting bone mineralization [Citation5,Citation6]. Some in vitro and animal studies have reported that chondrocytes, osteoblasts, osteoclasts and bone marrow mesenchymal stem cells participate in bone remodelling, bone mineralization and bone injury repair to maintain bone density and bone mass [Citation7]. Oxidative stress can reduce the level of bone formation by regulating the differentiation and survival of osteoblasts [Citation8]. ROS in vivo can inhibit the differentiation and mineralization of osteoblasts and induce the apoptosis of osteoblasts [Citation9,Citation10]. What’s more, ROS can activate osteoclasts and promote osteoclast differentiation and bone resorption, both of which can disrupt the balance of bone remodelling and lead to bone resorption higher than bone formation, leading to osteoporosis [Citation10,Citation11].
α-Lipoic acid (ALA) is a natural dimercaptan active compound. ALA is known as a ‘universal antioxidant’ and plays a vital role in the function of different oxidation-metabolism enzymes [Citation12]. ALA and its reduced form dihydrolipoic acid both have antioxidant effects in vivo [Citation13]. Because ALA has a strong antioxidant activity, its effects on osteoblasts and osteoclasts are also of concern. ALA can inhibit the level of intracellular oxidative stress, promote the proliferation and differentiation of osteoblasts [Citation14], inhibit the apoptosis of osteoblasts, inhibit the differentiation and maturation of osteoclasts [Citation15] and promote the deposition of calcium salts in vivo [Citation11]. It has the effect of treating osteoporosis.
In osteoporosis treatment, various nanoparticles have been used as nanocarriers for conjugated drugs or peptides [Citation16]. Although some nanoparticles have been used in the orthopaedics field, suitable nanoparticles for the treatment of osteoporosis are highly limited. Among them, gold nanoparticles are promising agents in the field of tissue engineering and regenerative medicine [Citation17]. GNPs with low toxicity, simple synthesis and good biocompatibility have been widely used in drug detection, disease diagnosis and cell imaging in the field of medicine [Citation18]. The local surface plasmon resonance property of GNPs makes them have good photothermal conversion efficiency [Citation19]. Thus, GNPs are one of the most widely used photothermal therapeutic agents. GNPs can be linked to thiol compounds by forming a gold-sulfur (AU-S) coordination bond, which enables the drug to be loaded onto the surface of the gold nanoparticles [Citation20]. Due to the accumulation in different tissues and lack of biodegradability of gold nanoparticles and other metal nanoparticles, their use in drug delivery in vivo is limited, and there are certain safety problems. However, Hydrophilic modification can improve the distribution and elimination of hollow gold in vivo. Hainfeld et al. [Citation21] modified GNPs with anti-EGFR antibody for photothermal treatment of mice inoculated with human squamous cell carcinoma, and the results showed that the tumour was completely ablated with almost no normal tissue damage. In addition, GNPs can combine photothermal therapy with chemotherapy to achieve synergism. You et al. [Citation22] loaded doxorubicin onto hollow gold nanospheres coated with polyethylene glycol (PEG) and produced photothermal effect through NIR irradiation. The photothermal effect was combined with chemotherapy to enhance antitumor activity and reduce toxicity. In addition to being a drug carrier, gold nanoparticles also play a unique role in the field of orthopaedics. GNPs can stimulate the expression of osteoblasts and chondrocytes related growth factors [Citation23], inhibit the growth of osteoclasts and reduce the production of ROS [Citation24]. It also has a certain anti-inflammatory effect [Citation25]. Hollow gold nanoparticle (HGNPs) is a kind of spherical gold nanoparticle with hollow structure. With its unique cavity structure and large cross-sectional area, HGNPs have higher drug loading capacity, higher photothermal conversion ability, and have the characteristics of low toxicity, small size (30–60 nm in diameter), spherical shape, etc. [Citation26]. However, the application of HGNPs in the treatment of osteoporosis is rarely reported.
In this study, HGNPs were prepared by a cobalt template method [Citation27]. PEG modification was used to prolong the cycle time of nanoparticles, and ALA and mPEG-HGNPs was loaded by crosslinking through Au-S covalent bond (Scheme 1), simultaneous detection mPEG@HGNPs-ALA scavenges ABTS capability. Then we tested the ability of mPEG@HGNPs-ALA to scavenge ROS induced by H2O2. These results may be mPEG@HGNPs-ALA provides supportive preclinical evidence as a potential clinical application of osteoporosis related diseases caused by elevated ROS.
Materials and methods
Materials
Sodium citrate (>99%), cobalt chloride hexahydrate (99.99%), sodium borohydride (99%) and chloroauric acid trihydrate (American Chemical Society reagent grade) were from Sigma Chemical Co, Ltd. (Saint Louis, MO, USA) and were used as received. Polyvinylpyrrolidone K30 (PVP-K30) (molecular weight = ∼40,000) was purchased from Anhui Shanhe Pharmaceutical Auxiliary Co., Ltd. (Huainan, China). ALA was purchased from Aladdin (Shanghai, China). mPEG-SH (Mw = 5000) was purchased from Shanghai Peng Sheng Biotechnology Co., Ltd. (Shanghai, China).
Synthesis of HGNPs and mPEG@HGNPs
The synthesis of HGNPs was reported in the previous literature [Citation27]. In short, 6 mL 0.05 M sodium citrate solution, 300 μL 20% poly-N-vinylpyrrolidone (PVP K30) solution and 300 μL 0.4 M cobalt chloride (CoCl2) solution were added to 300 mL ultrapure water. After 15 min of vacuum stirring, add 6 mL 0.1 M NaBH4 solution and continue reaction for 15 min after discolouring. Add 1.2 mL of 25 mM HAuCl4 and shake vigorously until the solution turns blue-green. The above solution was centrifuged in a high-speed frozen centrifuge at 10,000 rpm for 15 min. The HGNPs solution was obtained by discarding the supernatant and redispersing the precipitation with ultrapure water. The modified mPEG@HGNPs was obtained by taking an appropriate amount of HGNPs with a concentration of 100 μg/mL, adding excessive mPEG-SH under stirring condition, stirring overnight at room temperature, centrifugation at 10,000 rpm for 20 min and collecting precipitation.
Synthesis of mPEG@HGNPs-ALA
Weigh an appropriate amount of lipoic acid, dissolve it in 0.5% sodium dodecyl sulphate (SDS) solution, prepare a concentrated solution of 500 μg/mL and then dilute it with ultrapure water to an appropriate concentration. According to the HGNPs and methoxy polyethylene glycol (mPEG-SH, Mw = 5000) mass ratio of 5:1 feeding, stirring for 8 h to obtain mPEG@HGNPs. Then, mPEG@HGNPs and ALA with mass ratios of 1:0.5, 1:1 and 1:2 was reacted and stirred for 8 h to get mPEG@HGNPs-ALA. The supernatant was collected by centrifugation at 10,000 rpm for 20 min, and the ALA content was determined by a high-performance liquid chromatography (UV3000 HPLC, Agilent, USA) with a UV detector at 215 nm. A C18 column (4.6 × 100 mm, 3.5 μm, Agilent) was used for separation at temperature of 35 °C. The mobile phase used was a mixture of 60% phosphoric acid and 40% acetonitrile at the flow rate of 1.0 mL/min. The reaction efficiency (%) was calculated according to EquationEquation (1)(1)
(1) :
(1)
(1)
where m0 is the weight of ALA added for reaction and mn is the weight of unreacted ALA.
Synthesis of mPEG@GNPs-ALA
The 50 mL of 250 μM HAuCl4 was heated to boiling with vigorous stirring. Next, 1 mL of 1 wt% sodium citrate was rapidly added into the mixture, which resulted in a colour change from blue to burgundy. After further stirring for 15 min, the resulting GNPs solution was cooled to room temperature. The prepared GNPs were uncentrifuged (KDC-140HR, AnHui ustc ZonKia Scientific Instruments Co., Ltd.) for 10 min at the 5000 rpm by ultrafiltration (10 kDa, Nanjing Wanqing). mPEG-SH (100 μg/mL) of 1.5 molar mass GNPs was added to the stirring condition and stirred overnight at room temperature. The obtained mPEG@GNPs were purified by ultrafiltration. Stir according to different GNPs to ALA mass ratio. The reaction efficiency (%) was calculated according to EquationEquation (1)(1)
(1) .
Characterization of HGNPs, mPEG@GNPs-ALA and mPEG@HGNPs-ALA
The hydrodynamic diameter of HGNPs, mPEG@HGNPs and mPEG@HGNPs-ALA were determined by a zeta sizer (Brookhaven Instruments Corporation, NY). The ultraviolet absorption spectrum at 200–1000 nm was scanned by UV–vis spectrophotometer (UV1800, Shimadzu, Japan). Finally, the particle size and best morphology of products were evaluated by transmission electron microscopy (TEM, Zeiss-EM10C-100 KV, Germany).
In vitro release of ALA
The experiment was divided into two groups, namely, free ALA group, mPEG@GNPs-ALA group and mPEG@HGNPs-ALA group (n = 3). 0.5 mL of each of the three solutions was placed in dialysis bags of 3.5 kDa, and the releasing medium was 10 mL PBS (pH 7.4) solution containing 0.5% SDS. The solution was placed at 37 °C and shaken for 24 h at 100 rpm. The 1 mL was sampled at 15 min, 30 min, 45 min, 1 h, 2 h, 4 h, 6 h, 8 h, 10 h, 12 h and 24 h, respectively. An isothermal release medium of 1 mL was added to collect samples at each time point. The content of lipoic acid was determined according to the chromatographic conditions. The cumulative release rate was calculated according to EquationEquation (2)(2)
(2) :
(2)
(2)
where Qn is the cumulative release amount of ALA, V is the volume of the releasing medium, Ci is the concentration of ALA at each time point, W is the total dose and Vx is the volume of the release medium taken out.
ALA antioxidant capacity assay
The 7.5 mL 7 mM ABTS solution and 132 μL 140 mM K2S2O8 solution were reacted against light for 16 h. Before use, dilute with water until the absorbance value at 732 nm is ∼0.7 [Citation28]. The reaction principle is shown in .
Add 100 μL ABTS and 50 μL samples into the 96-well plate, and place in darkness for 10 min. The absorbance value (Ai) was then measured at 734 nm (n = 3). Distilled water was used as the control (A0, H2O + ABTS), and distilled water was used as the negative control (Ai0, H2O + sample). The experiment was divided into three groups: (1) ALA, (2) mPEG@GNPs-ALA, and (3) mPEG@HGNPs-ALA. The concentration of ALA was 41.6 μg/mL. The clearance rate is calculated according to EquationEquation (3):(3)
(3)
(3)
(3)
Cytotoxicity of different preparations
MC3T3-E1 cells were obtained from American Type Culture Collection. MC3T3-E1 cells were cultivated in DMEM medium containing 10% foetal bovine serum, 1 mM l-glutamine, 1% penicillin–streptomycin at 37 °C and 5% CO2. The MC3T3-E1 cells were seeded in a 96-well plate at a density of 5000 cells per well. After cells adhesion, ALA, mPEG@GNPs-ALA and mPEG@HGNPs-ALA groups treated MC3T3-E1 cells with the concentrations of 1, 5, 10, 25, 50, 75, 100 and 150 μg/mL, respectively. After 24, 48 and 72 h incubation, MTT was added and incubated for 4 h. Then, the medium was removed and 150 μL of DMSO was added to each well and the optical density was measured at a wavelength of 570 nm using a Microplate Reader (POLARstar Omega, Germany). The relative cell viability (%) was calculated according to EquationEquation (4)(4)
(4) :
(4)
(4)
where the ODsample is the absorbance of the cells incubated with the nanoparticle sample; the ODblank is the absorbance of the blank medium without cells and the ODcontrol is the absorbance of the blank medium with cells.
Rt-qPCR detection
Total RNA was extracted from osteoblasts by the Trizol method and reverse transcribed into cDNA using the PrimeScript RT Reagent Kit with gDNA Eraser Kit. Quantitative PCR was performed with TBGreen® Premix Ex Taq™, GAPDH was used as an internal reference, and relative quantification was calculated using the δδCT method. Related primer sequences are shown in .
Table 1. Sequences of primers used in quantitative real-time PCR.
H2O2-induced cells oxidative injury
The MC3T3-E1 cells were seeded in a 96-well plate at a density of 5000 cells per well. After cells adhesion, different concentrations of H2O2 (0.1, 0.25, 0.5, 0.75, 1.0, 1.25 and 1.5 mM) were added for 4 h. Then, MTT was added and the relative cell viability (%) was calculated according to EquationEquation (4)(4)
(4) .
mEPG@HGNPs-ALA protected cells against oxidative injury
After reaching confluence, the MC3T3-E1 cells were treated with medium containing different concentrations of mPEG@GNPs-ALA and mEPG@HGNPs-ALA (5, 25, 75 and 150 μg/mL) for 24 h before treatment with H2O2 (a final concentration of 1.5 mM) for 4 h.
Statistical analysis
All results were derived from triplicate experiments. The results were presented as mean ± SD. One-way ANOVA was used to assess the statistical significance of the difference between group means. A value of p < 0.05 was considered statistically significant.
Results and discussion
Characterization of HGNPs and GNPs
The particle size distribution of HGNPs is shown in , with the particle size of 54.6 nm and PDI of 0.059. After ultraviolet spectrum scanning, the maximum absorption wavelength is shown in , and the maximum absorption wavelength was 777 nm. TEM images showed that the morphology of HGNPs is spherical and hollow (). The size of GNPs was 26.9 nm with the PDI of 0.151 (). The maximum absorption wavelength of GNPs was 545 nm (). TEM images showed that the morphology of GNPs is spherical and solid ().
Figure 2. (A) The size of HGNPs. (B) The absorption spectra of HGNPs. (C) The morphology of HGNPs. (D) The size of GNPs. (E) The absorption spectra of GNPs. (F) The morphology of GNPs. (G) Effect of different mass ratios on reaction efficiency. (H) The diameters of HGNPs, mPEG@HGNPs and mPEG@HGNPs-ALA. (I) The zeta potential of HGNPs, mPEG@HGNPs and mPEG@HGNPs-ALA (*p < 0.05, **p < 0.01, ***p < 0.001 and ****p < 0.0001).

The determination of drug loading and the particle size before and after loading
The content of ALA was determined according to the chromatographic conditions in 2.3, and the results are shown in . Compared with mEPG@GNPs-ALA, the reaction efficiency of mEPG@HGNPs-ALA was higher. When designing drug delivery systems, the size and porosity of nanoparticles could influence drug loading capacity [Citation29]. HGNPs had higher specific surface area, more active centres than solid gold nanoparticles [Citation30], so HGNPs had higher drug loading capacity than GNPs. When the mass ratio of gold and ALA was 1:2, the reaction efficiency reached the maximum. The reaction efficiency is stable at ∼4.7%.
Comparison of particle size before and after loading
As shown in , the particle size of mPEG@HGNPs and PDI were 63.8 nm and 0.099, respectively, which were 6.9 nm larger than HGNPs. The particle size of mPEG@HGNPs-ALA and PDI were 73 nm and 0.107, respectively, which were 16.1 nm larger than HGNPs. We also determined the zeta potential of HGNPs, mPEG@HGNPs and mPEG@HGNPs, which were −17.61, −20.62 and −21.87 mV, respectively.
In vitro release of ALA
Calculate the cumulative release rate according to EquationEquation (2)(2)
(2) . shows that free ALA released more than 90% at 37 °C for 24 h. ALA released from mPEG@HGNPs-ALA was 56.00%. Compared with free drugs, HGNPs and GNPs can enhance the sustained-release effect of drugs, which may improve the distribution and circulation time of drugs in the body and improve the therapeutic effect of osteoporosis. However, there was no significant difference in drug release between mPEG@GNPs-ALA and mPEG@HGNPs-ALA.
Figure 3. (A) In vitro release of ALA from free ALA, mPEG@GNPs-ALA and mPEG@HGNPs-ALA. (B) ABTS clearance rate of ALA, GNPs, HGNPs, mPEG@GNPs-ALA and mPEG@HGNPs-ALA. (C) The viability of cells treated with different concentrations of H2O2. (D) Cell viability of MC3T3-E1 cells treated with nanoparticles for 24 h. (E) Cell viability of MC3T3-E1 cells treated with nanoparticles for 48 h. (F) Cell viability of MC3T3-E1 cells treated with nanoparticles for 72 h. (G) Cell viability of macrophage treated with nanoparticles for 24 h. (H) Cell viability of macrophage treated with nanoparticles for 48 h. (I) Cell viability of macrophage treated with nanoparticles for 72 h. (*p < 0.05, **p < 0.01, ***p < 0.001 and ****p < 0.0001).

Antioxidant capacity evaluation
The results shown in state that mPEG@HGNPs-ALA had antioxidant capacity. When the concentration of ALA was 41.6 μg/mL, the clearance rate of ALA, mPEG@GNPs-ALA and mPEG@HGNPs-ALA was 27.45%, 33.55% and 42.56%, respectively, indicating that mPEG@HGNPs-ALA had antioxidant capacity. Therefore, mPEG@HGNPs-ALA had a good application prospect in the treatment of osteoporosis.
Cytotoxicity evaluation
MC3T3-E1 cells and macrophages were used to investigate the cytotoxicity. The results are shown in . At ALA concentration of 1–150 μg/mL, the viability of cells in mPEG@GNPs-ALA and mPEG@HGNPs-ALA groups were greater than 100%. With the increase in ALA concentration, cell viability increased. It indicated that mPEG@GNPs-ALA and mPEG@HGNPs-ALA exhibited great biocompatibility with MC3T3-E1 and could promote the proliferation of osteoblasts. It may be because gold nanoparticles are absorbed by osteoblasts and accumulated in the perinuclear compartment and vascular structure. At the same time, gold nanoparticles can up regulate the expression of BMP-2, Runx-2, OCN and COL-1 and promote the proliferation of osteoblasts [Citation23]. What’s more, free ALA, mPEG@HGNPs-ALA and mPEG@GNPs-ALA had little cytotoxicity at concentration of 1–150 μg/mL, indicating that they were highly biocompatible.
H2O2-induced cells oxidative injury
MTT results showed that the cell viability of 1.25 mmol/L H2O2 for 4 h was significantly reduced (). The cell viability was 46.88%, and it was less than 50%, which could be used as the damage condition for subsequent experiments.
mEPG@HGNPs-ALA upregulated Runx-2, BMP-2 and OCN expression
BMP-2, Runx-2 and OCN are important transcriptional regulators in bone development and play an important role in bone formation and remodelling. BMP-2 is one of the most effective promoters of MSC differentiation into osteoblasts in vitro and upregulates the expression of Runx-2 during osteoblast differentiation [Citation23]. As a key regulator of bone formation, Runx2 can promote the proliferation and differentiation of early osteoblasts and affect the secretion of other bone formation related cytokines. OCN is a marker of osteoblast differentiation and maturation. As shown in , ALA, mPEG@HGNPs-ALA and mPEG@GNPs-ALA all upregulated the expression of BMP-2, Runx2 and OCN, indicating that mPEG@HGNPs-ALA is beneficial for osteoblasts at the gene level.
Figure 4. (A) Expression levels of Runx-2, BMP-2 and OCN at 24 h. (B) Expression levels of Runx-2, BMP-2 and OCN at 48 h. (C) Expression levels of Runx-2, BMP-2 and OCN at 72 h. (D) mPEG@HGNPs-ALA enhanced MC3T3-E1 cell viability after treatment with H2O2 (*p < 0.05, **p < 0.01, ***p < 0.001 and ****p < 0.0001).

mEPG@HGNPs-ALA protected cells against oxidative injury
As shown in , mPEG@HGNPs-ALA could increase the survival MC3T3-E1 cells treated with H2O2. With the increase concentration of mPEG@HGNPs-ALA, the cell viability was significantly improved (p < 0.05). When the mPEG@HGNPs-ALA concentration was 150 μg/mL, the cell viability was 75.31%. Compared with the cells treated with H2O2 alone, cell viability increased by 28.03% (p < 0.0001). It indicated that ALA can effectively remove ROS produced by H2O2. These results suggest that mPEG@HGNPs-ALA can effectively eliminate ROS for the treatment of osteoporosis.
Conclusion
In this study, we successfully synthesized mPEG@HGNPs-ALA as a new nanomaterial to treat osteoporosis. First, a cobalt template method was used to prepare HGNPs with controllable particle size. The particle size was 54.6 nm, and the maximum ultraviolet absorption wavelength was ∼777 nm. ALA was self-assembled on the surface of HGNPs by Au-S bond and the preparation method was optimized. At the same time, HGNPs were modified with mPEG to improve their stability. mPEG@HGNPs-ALA had antioxidant ability and biocompatibility. mPEG@HGNPs-ALA increased the viability of MC3T3-E1 cells after H2O2 injury, which has the prospect of treating osteoporosis. Finally, we verified that gold nanoparticles can also upregulate the expression levels of BMP-2, Runx-2 and OCN to regulate osteoblast differentiation at the gene level. In conclusion, mPEG@HGNPs-ALA may be used as a drug carrier in bone and tissue engineering.
Author contributions
Xiaojian Ye contributed to conceptualization, data curation, formal analysis, investigation, visualization and writing—original draft. Wenming Pan contributed to visualization and writing—original draft. Yanyan Liu, Yanguo Su, Ji Liu and Guohua Xu contributed to visualization and data curation. Daquan Chen contributed to conceptualization, data curation and methodology. Yanhai Xi contributed to project administration, resources, supervision, validation and writing—review and editing. All authors approved the final version of the manuscript.
Disclosure statement
The authors declare that the research was conducted in the absence of any commercial or financial relationships that could be construed as a potential conflict of interest.
Data availability statement
All data could be obtained by contacting the authors.
Additional information
Funding
References
- Pietschmann P, Mechtcheriakova D, Meshcheryakova A, et al. Immunology of osteoporosis: a mini-review. Gerontology. 2016;62(2):128–137.
- Lee D, Heo DN, Kim H-J, et al. Inhibition of osteoclast differentiation and bone resorption by bisphosphonate-conjugated gold nanoparticles. Sci Rep. 2016;6(1):27336–27311.
- Wauquier F, Leotoing L, Coxam V, et al. Oxidative stress in bone remodelling and disease. Trends Mol Med. 2009;15(10):468–477.
- Zhou Q, Zhu L, Zhang D, et al. Oxidative stress-related biomarkers in postmenopausal osteoporosis: a systematic review and meta-analyses. Dis Markers. 2016;2016:7067984.
- Holroyd C, Cooper C, Dennison E. Epidemiology of osteoporosis. Best Pract Res Clin Endocrinol Metab. 2008;22(5):671–685.
- Pinna A, Baghbaderani MT, Hernandez VV, et al. Nanoceria provides antioxidant and osteogenic properties to mesoporous silica nanoparticles for osteoporosis treatment. Acta Biomater. 2021;122:365–376.
- Bai X, Gao Y, Zhang M, et al. Carboxylated gold nanoparticles inhibit bone erosion by disturbing the acidification of an osteoclast absorption microenvironment. Nanoscale. 2020;12(6):3871–3878.
- Baek KH, Oh KW, Lee WY, et al. Association of oxidative stress with postmenopausal osteoporosis and the effects of hydrogen peroxide on osteoclast formation in human bone marrow cell cultures. Calcif Tissue Int. 2010;87(3):226–235.
- Lagouge M, Larsson NG. The role of mitochondrial DNA mutations and free radicals in disease and ageing. J Intern Med. 2013;273(6):529–543.
- Lin C-H, Li N-T, Cheng H-S, et al. Oxidative stress induces imbalance of adipogenic/osteoblastic lineage commitment in mesenchymal stem cells through decreasing SIRT1 functions. J Cell Mol Med. 2018;22(2):786–796.
- Fu C, Xu D, Wang CY, et al. Alpha‐lipoic acid promotes osteoblastic formation in H2O2‐treated MC3T3‐E1 cells and prevents bone loss in ovariectomized rats. J Cell Physiol. 2015;230(9):2184–2201.
- Golbidi S, Badran M, Laher I. Diabetes and alpha lipoic acid. Front Pharmacol. 2011;2:69.
- Rochette L, Ghibu S, Richard C, et al. Direct and indirect antioxidant properties of α-lipoic acid and therapeutic potential. Mol Nutr Food Res. 2013;57(1):114–125.
- Lin Z, Guichun Z, Lifeng L, et al. Protective effect of α-lipoic acid against antimycin a cytotoxicity in MC3T3-E1 osteoblastic cells. Cell Stress Chaperones. 2017;22(1):5–13.
- Kondo H, Takeuchi S, Togari A. β-Adrenergic signaling stimulates osteoclastogenesis via reactive oxygen species. Am J Physiol Endocrinol Metab. 2013;304(5):E507–E515.
- Nah H, Lee D, Lee JS, et al. Strategy to inhibit effective differentiation of RANKL-induced osteoclasts using vitamin D-conjugated gold nanoparticles. Appl Surf Sci. 2020;527:146765.
- Daraee H, Eatemadi A, Abbasi E, et al. Application of gold nanoparticles in biomedical and drug delivery. Artif Cells Nanomed Biotechnol. 2016;44(1):410–422.
- You J, Zhang G, Li C. Exceptionally high payload of doxorubicin in hollow gold nanospheres for near-infrared light-triggered drug release. ACS Nano. 2010;4(2):1033–1041.
- Fairbairn N, Christofidou A, Kanaras AG, et al. Hyperspectral darkfield microscopy of single hollow gold nanoparticles for biomedical applications. Phys Chem Chem Phys. 2013;15(12):4163–4168.
- Wang F, Wang Y-C, Dou S, et al. Doxorubicin-tethered responsive gold nanoparticles facilitate intracellular drug delivery for overcoming multidrug resistance in cancer cells. ACS Nano. 2011;5(5):3679–3692.
- Hainfeld JF, O’Connor MJ, Lin P, et al. Infrared-transparent gold nanoparticles converted by tumors to infrared absorbers cure tumors in mice by photothermal therapy. PLoS One. 2014;9(2):e88414.
- You J, Zhang R, Zhang GD, et al. Photothermal-chemotherapy with doxorubicin-loaded hollow gold nanospheres: a platform for near-infrared light-trigged drug release. J Control Release. 2012;158(2):319–328.
- Zhang D, Liu D, Zhang J, et al. Gold nanoparticles stimulate differentiation and mineralization of primary osteoblasts through the ERK/MAPK signaling pathway. Mater Sci Eng C Mater Biol Appl. 2014;42:70–77.
- Sul O-J, Kim J-C, Kyung T-W, et al. Gold nanoparticles inhibited the receptor activator of nuclear factor-κb ligand (RANKL)-induced osteoclast formation by acting as an antioxidant. Biosci Biotechnol Biochem. 2010;74(11):2209–2213.
- Dohnert MB, Venâncio M, Possato JC, et al. Gold nanoparticles and diclofenac diethylammonium administered by iontophoresis reduce inflammatory cytokines expression in Achilles tendinitis. Int J Nanomed. 2012;7:1651–1657.
- Wang R, Deng J, He D, et al. PEGylated hollow gold nanoparticles for combined X-ray radiation and photothermal therapy in vitro and enhanced CT imaging in vivo. Nanomedicine. 2019;16:195–205.
- Li Y, He D, Tu J, et al. The comparative effect of wrapping solid gold nanoparticles and hollow gold nanoparticles with doxorubicin-loaded thermosensitive liposomes for cancer thermo-chemotherapy. Nanoscale. 2018;10(18):8628–8641.
- Ilyasov IR, Beloborodov VL, Selivanova IA, et al. ABTS/PP decolorization assay of antioxidant capacity reaction pathways. Int J Mol Sci. 2020;21(3):1131.
- Xu P, Wang R, Yang W, et al. A DM1-doped porous gold nanoshell system for NIR accelerated redox-responsive release and triple modal imaging guided photothermal synergistic chemotherapy. J Nanobiotechnol. 2021;19(1):77.
- Ihsan A, Katsiev H, Alyami N, et al. From porous gold nanocups to porous nanospheres and solid particles–a new synthetic approach. J Colloid Interface Sci. 2015;446:59–66.