Abstract
Acute respiratory distress syndrome (ARDS) is a complication of pulmonary disease that produces life-threatening hypoxaemia. Despite ventilation and hyperoxic therapies, undetected hypoxia can manifest in capillary beds leading to multi-organ failure. Ox66™ is an ingestible, solid-state form of oxygen designed to supplement oxygen deficits. Twenty-four anaesthetized rats underwent a two-hit model of respiratory distress (ARDS), where a single dose (5 mg/kg) of lipopolysaccharide (LPS) was given intratracheally, and then the respiratory tidal volume was reduced by 40%. After 60 min, animals were randomized to receive Ox66™, or normal saline (NS; vehicle control) via gavage or supplemental inspired oxygen (40% FiO2). A second gavage was administered at 120 min. Cardiovascular function and blood oximetry/chemistry were measured alongside the peripheral spinotrapezius muscle’s interstitial oxygenation (PISFO2). ARDS reduced mean arterial pressure (MAP) and PISFO2 compared to baseline (BL) for all treatment groups. Treatment with Ox66 or NS did not improve MAP, but 40% FiO2 caused a rapid return to BL. PISFO2 improved after treatment with Ox66™ and 40% FiO2 and remained elevated for both groups against NS until study conclusion. Both oxygen treatments also suppressed the inflammatory response to LPS, suggesting that Ox66™ can deliver therapeutically-impactful levels of oxygen in situations of pulmonary dysfunction.
Introduction
Acute respiratory distress syndrome (ARDS) is a critical failure of lung function. Onset can be rapid (hours) or progressive (days) and is clinically recognized as dyspnoea, moderate to severe hypoxaemia, and diffuse damage to alveoli or pulmonary microvasculature. ARDS annually kills over 155,000 people in the United States (average for years 1999–2013), disproportionately impacting elderly populations [Citation1]. With the emergence of COVID-19, ARDS has taken centre stage as novel SARS-CoV-2 infections resulting in ARDS carry up to a 75% mortality rate [Citation2].
Pathophysiologically, ARDS arises from inflammatory injury to the alveoli capillary barrier [Citation3]. Varying magnitudes of corresponding pulmonary vasoconstriction, alveolar vascular collapse and oedema further reduce the number of active pulmonary gas exchange units [Citation4]. The result is an alveolar ventilation-perfusion mismatch where well-ventilated alveoli receive insufficient or no perfusion, while well-perfused alveoli receive little or no ventilation [Citation5]. This hallmark increase in the fraction of dead space ventilation is a proportionate indicator of mortality [Citation6–9].
Supportive therapies such as lung-protective (low tidal volume) ventilation [Citation10], supplemental oxygen [Citation11], prone positioning [Citation12] and extracorporeal membrane oxygenation [Citation13,Citation14] prevail as clinical treatments. However, existing pulmonary damage can limit the efficacy of interventions reliant on the respiratory pathway and carry the risk of complications like ventilator-induced lung injury and hyperoxic acute lung injury. Since ventilatory and oxygenation parameters must be carefully titrated to individual needs, over-oxygenation is common in the clinic. A 2012 retrospective found that 74% of patients with acute lung injury and invasive mechanical ventilation received excessive FiO2 per their needs, which resulted in a worse oxygen index at 48 h [Citation15]. Still, patients who do not receive any form of oxygen fare far worse, with hypoxia-driven sepsis and multi-organ failure being the chief cause of death in ARDS patients [Citation16].
Ox66™ (Hemotek, Plano, TX) is a powdered (solid-state) form of diatomic oxygen (O2). Its molecular structure is an aluminium hydroxide clathrate enclosing 66.2% O2 by weight. Ingested Ox66™ has previously been evaluated for safety in healthy rats at Charles River Laboratories and mesenteric oxygen-transfer efficacy at Song Biotechnologies with encouraging results [Citation17]. We recently demonstrated significant outcomes in tissue oxygenation, lactate, PaO2, SO2 and base deficit compared to vehicle control in a hypoventilatory model of ARDS [Citation18].
The purpose of this study was to evaluate the efficacy of oral oxygen supplementation (Ox66™) in a murine model of ARDS combining a previous model of hypoventilation with inflammation. Lipopolysaccharide (LPS) was administered to the lungs prior to hypoventilation, and treatment with Ox66™ was compared to vehicle control (normal saline [NS]) and conventional, respiratory-based oxygen supplementation (40% FiO2). Study parameters were direct measurement of tissue oxygenation in peripheral microvascular regions, blood chemistry, cardiovascular outputs, oximetry and inflammatory biomarker multiplexing.
Methods
Animals
The following protocol and experimental procedures – performed by Song Biotechnologies, LLC – were approved by the Thomas D. Morris, Inc. Institutional Animal Care and Use Committee (Protocol # 20-007) and are consistent with the National Institutes of Health guidelines for the humane treatment of laboratory animals. Study subjects were male Sprague Dawley rats (N = 24; 250–300 g; 8–10 weeks old; Charles River Laboratories, Inc., Wilmington, MA) housed in pairs with a 12/12 day-night cycle and provided ad libitum access to feed and water. The study was unblinded and animals were enrolled into treatment groups through random lottery at the time of treatment. Two experiments (AM and PM) typically occurred in a day to minimize variability due to animal weight gain and ageing.
Surgical preparation and intravital microscopy
Surgical preparation and microscopy proceeded as previously described [Citation18]. Briefly, animals were anaesthetized with 1–5% isoflurane, then switched to IV alfaxalone acetate (Alfaxan, Schering-Plough Animal Health, Welwyn Garden City, UK) for maintenance. Cardiovascular parameters were monitored by a pressure transducer connected to a femoral artery cannula (BIOPAC MP-150, BIOPAC Systems, Goleta, CA). Blood samples were collected through the same femoral artery cannula to measure blood gases and chemistry (ABL90 Flex, Radiometer, Denmark). Cannulas were kept free of clots with heparinized phosphate-buffered NS (20 IU Heparin Sodium per ml; Pfizer, New York, NY), which was not infused. Animals continued to inspire room air without artificial ventilation until BL metrics were collected. Mechanical ventilation (RoVent® Jr, Kent Scientific, Torrington, CT) occurred with medical air (21% oxygen, 78% nitrogen: Airgas, Radnor Township, PA) for all groups except the 40% FiO2 group, which was switched to 40% oxygen, 59% nitrogen (Airgas) at the 60-min mark.
The rat spinotrapezius muscle was utilized for the investigation of microcirculatory parameters. This thin skeletal muscle was exteriorized as described by Gray [Citation19] and secured in situ onto a thermostable animal platform adapted for microcirculatory studies [Citation20–22] and measurement of the partial pressure of oxygen in the interstitium (PISFO2) as previously described [Citation23–27]. Measurement of arterial diameters occurred on an upright microscope Axio Imager M2m, Carl Zeiss AG, Oberkochen, Germany) with a 5X/0.25 objective (N-Achroplan; Zeiss), which was focused in the diametral plane. Images were captured using a colour CMOS camera (Axiocam 305 colour, Zeiss, Germany) and stored digitally. Internal vessel diameters were measured with a digital ruler (ZEN 3.3 Blue Edition, Zeiss, Germany) calibrated using a standard microscope micrometer (Stage Micrometer, Zeiss). Only arterioles between 50 and 100 microns at baseline (BL) were included.
Anaesthesia was maintained through the end of experimentation when rats were euthanized with an IV infusion of Euthasol (150 mg/kg, pentobarbital component; Delmarva, Midlothian, VA).
Multiplex
The MILLIPLEX® MAP Rat Cytokine/Chemokine Magnetic Bead Panel (Cat# RECYMAG65K27PMX; Millipore Sigma, Burlington, MA) ran on Luminex® MAGPIX® hardware with xPONENT® software (Luminex, Austin, TX) to measure plasma expression of cytokines and chemokines. Two separate plasma samples were drawn per time point (BL and 240 min) per animal. Samples were frozen and multiplexed by the Bruce Lab at Baylor University (Waco, TX). Output data in ng/mL were converted to fold change at 240 min compared to BL.
Treatment groups
Rats were randomly assigned to receive either a gavage of 2.5 mL NS (BD PosiFlush™, Becton, Dickinson, and Company, Franklin Lakes, NJ) as a vehicle control; Ox66™ (Ox66; Hemotek) at 1 g/kg suspended in 2.5 mL NS, or inspired oxygen supplementation (40% FiO2; Airgas, Radnor Township, PA) delivered through a ventilator from a pre-mixed 40% FiO2 tank.
Experimental protocol
Following surgical preparation, animals were allowed to reach a steady state (15–30 min) on the 3D-printed, thermoregulated platform used for intravital microscopy. BL was recorded while the animal was still spontaneously breathing room air. Systemic variables consisted of mean arterial pressure (MAP), pulse pressure (PP), heart rate (HR), hindlimb oximetry and temperature. Microcirculatory PISFO2 data were obtained from three to five capillary-dominant interstitial sites – conserved throughout the experiment – located 200 µm from arterioles and venules. Additionally, 65 µL of arterial blood was collected for oximetry and chemical analysis via ABL90 Flex (Radiometer; Copenhagen, Denmark). Subsequent systemic and microcirculatory metrics were recorded every 15 min and blood gases at BL and 60, 120, 180 and 240 min.
After BL, a state of inflammatory and mechanical pulmonary dysfunction (ARDS) was induced by intratracheally administering 5 mg/kg E. coli (O26:B6, Millipore Sigma) LPS and then immediately connecting the intubated animals to a mechanical ventilator (RoVent® Jr). The RoVent® Jr came with presets of both breath rate and tidal volume based on a rodent’s weight and was calibrated to maintain a 40% tidal volume reduction relative to the preset volume. For example, the preset tidal volume of a 300 g rat was 2.19 mL, and at the onset of the experiment, the tidal volume was reduced to 1.31 mL. The reduced tidal volume remained for the entirety of the experiment.
After 60 min of ARDS, animals were randomly treated with a gavage of either 2.5 mL NS (vehicle control; N = 8) or 1 g/kg Ox66™ suspended in 2.5 mL NS (N = 8) or switched to ventilation with 40% FiO2 (N = 8). The 40% respiratory tidal volume reduction remained in effect for all groups until study end. Animals receiving a gavage were treated with a second dose after two hours of ARDS (120 min post-BL), while the 40% FiO2 group received supplemental oxygen for the full duration of the experiment following initiation of treatment (post-60 min). The observation period lasted until 240 min post-BL when the study was terminated.
Statistics
Data are mean ± standard error of the mean (SEM). Statistical comparisons within and between experimental groups were made using two-way ANOVA (repeated measures or multiple comparisons as appropriate; Prism 9, GraphPad Software Inc., San Diego, CA). Significance was defined as p < 0.05. Intragroup comparisons were to BL, while inter-group comparisons were at each appropriate time point. In cases where a significant difference (p < 0.05) was detected between groups, a high stringency multiple comparison test (Tukey’s or Sidak’s) was performed. For differences within groups, Dunnett’s was used. An unpaired T-test was used for single metrics (weight, age, etc.).
Results
Animal characteristics and ARDS profile
Animal weights (NS: 302 ± 12; Ox66: 300 ± 12; 40% FiO2: 294 ± 8) and all physiological metrics and biomarkers were equivalent between groups at BL. The induction of ARDS by LPS lavage and subsequent tidal volume reduction rapidly reduced PISFO2 and MAP pressure in all groups (p ≤ 0.05). MAP stabilized but remained hypotensive after 15 min, while PISFO2 continued to decline for all groups (). At 60 min of ARDS, all groups had decreased pH, PAO2, PAO2/FiO2 and sO2 and elevated pCO2 compared to their BLs ().
Figure 1. Interstitial oxygen tension (PISFO2) of skeletal muscle capillary beds. The microvasculature of the spinotrapezius muscle was monitored at specific sites for interstitial fluid oxygenation throughout the experimental time course. Two-Way ANOVA detected no differences up to 60 min but saw post-treatment separation between NS and oxygen-containing treatments. About 40% FiO2 was higher than Ox66 for time points 60 and 75 (not illustrated). Data are mean SEM. Statistical annotation colour-coded to indicate significance vs. NS. *p < 0.05 vs. NS.
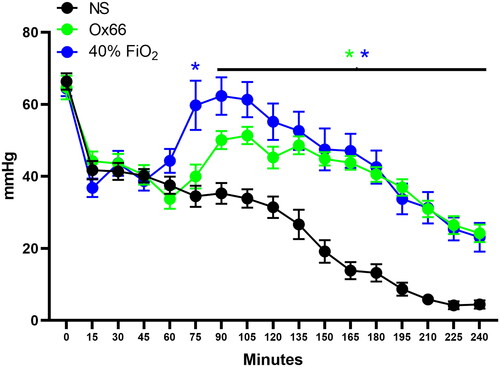
Table 1. Arterial blood gasses and metabolites.
Response to gavage and supplemental oxygen
Gavage with Ox66™ at 60 min of ARDS caused a steady increase in PISFO2 that became significantly higher than the NS group at 90 min (p ≤ 0.05). Treatment with 40% FiO2 caused a faster rise in PISFO2 but was on par with Ox66 after 90 min. The second gavage of Ox66™ at 120 min caused a slight rise in PISFO2; subsequent measurements were virtually identical to the 40% FiO2 group (). While PISFO2 responded to both Ox66™ gavage and 40% FiO2, PAO2 only rose for 40% FiO2 (p < 0.0001 vs. NS and Ox66), reaching and sustaining supraphysiologic levels (124 mmHg ± 5; p < 0.001 vs. BL). Ox66 remained identical to the NS profile (). This was also reflected in the calculated PAO2/FiO2 index () and SO2.
MAP rose in response to 40% FiO2 but not gavage with NS or Ox66™ (). HRs (BL: 545 ± 8, 428 ± 12 and 456 ± 9 mmHg, for NS, Ox66 and 40% FiO2, respectively) were generally highest for NS and lowest for 40% FiO2, but the only detected difference was at 180 min between NS (454 ± 10 BPM) and 40% FiO2 (390 ± 21 BPM; p = 0.046). PP (NS: 29 ± 2; Ox66: 25 ± 1; and 40% FiO2: 28 ± 1 mmHg at BL) did not vary between groups but was elevated by 10–12 mmHg for all between 60 and 120 min (p < 0.05 vs. BL) before returning to BL for the remainder of the observation period.
Figure 2. The PAO2/FiO2 index of pulmonary function. Arterial blood samples were used to measure PAO2, which was then divided by the fraction of inspired oxygen (FiO2) – 21% for NS and Ox66. 40% FiO2 returned the index to BL, while NS and Ox66™ had similar profiles. N = 8 per group. Data are mean ± SEM. βp < 0.05 vs. 0 min.
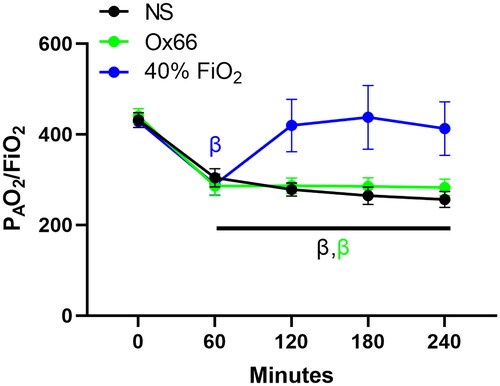
Figure 3. Mean arterial pressure. Arterial pressure data were collected continuously through an indwelling catheter and plotted at discrete time points to align with PISFO2 collection. Measurements before treatment did not differ between groups. NS and Ox66™ were virtually identical and remained suppressed from their BL. About 40% FiO2 was significantly elevated against most NS and Ox66 time points after treatment. It was also suppressed versus its BL but at a lower magnitude. Statistical annotations are colour-coded to correspond to their specific group. N = 8 per group. Data are mean ± SEM. *p < 0.05 vs. NS. αp < 0.05 vs. Ox66.
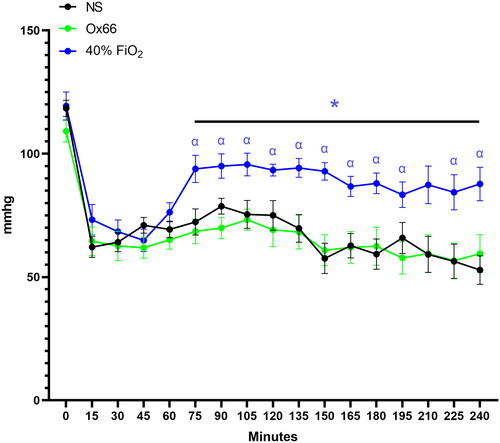
Arteriolar lumenal diameters did not show any significant change for any group. BL measurements were 78 ± 4, 81 ± 3 and 75 ± 4 µm for NS, Ox66 and 40% FiO2, respectively. The corresponding measurements at 240 min were 71 ± 5, 71 ± 5 and 75 ± 10 µm.
Inflammatory markers
contains the comprehensive panel of multiplexed plasma proteins expressed as fold change from BL. Overall, it shows a graded inflammatory response that was highest with NS and reduced in proportion to the amount of oxygen in each treatment (i.e. Ox66 had lower and 40% FiO2 lowest cytokine signalling vs. NS). Since NS was the vehicle control group, it showed the cleanest response to LPS and 40% tidal volume reduction with almost all markers elevated above BL. Ox66™’s impact (in terms of significant suppression) was primarily on general inflammatory cytokines and those responsible for inducing a T-cell response. This effect was further extended using 40% FiO2. The magnitude of inflammatory suppression was best associated with the PISFO2 response and the amount of oxygen injected by the treatment groups.
Table 2. Inflammatory biomarkers.
Discussion
A two-hit rat model of ARDS compared a novel, ingested, solid-state oxygen-containing compound to vehicle (NS) and a clinically standard, 40% FiO2 supplemental oxygen treatment. Ox66™ was previously shown to improve tissue oxygenation versus vehicle (NS) in a single-hit, mechanical simulation of ARDS [Citation18]. However, efficacy was not examined in the context of inflammation, which both compounds pulmonary insufficiency by burdening the circulatory system and functions as a disease marker. This study added an intratracheal LPS insult to the mechanically restricted breathing simulation of ARDS to determine the feasibility of Ox66™ gavage in improving oxygen delivery to hypoxic tissues.
ARDS in humans is a clinically challenging, biologically heterogenous syndrome that does not translate well from preclinical research models due to additional variation from species-specific physiologies [Citation28]. Since ARDS severity ultimately hinges on gas exchange inefficiency and oxygen is a primary form and goal of treatment, our approach focused on the oxygen transport chain. Tidal volume restriction limited gas diffusion to simulate the alveolar perfusion deficits in ARDS. Acute inflammation through LPS lavage then sought to add the inflammatory and cardiovascular burden that often manifests as decreased oxygenation to peripheral capillary beds like those in the spinotrapezius muscle.
For an equitable comparison, we kept this study’s injury and experimental protocol identical to the ‘single hit’ protocol in our previous gavage study [Citation18] except for the intratracheal LPS dose. In the first study, the 40% reduction in tidal volume alone caused a new steady state for PISFO2, MAP and blood gases, which were then responsive to Ox66™ gavage. The addition of LPS to this study induced a pathogen-mediated inflammatory response that was associated with a more pronounced suppression of MAP and a deteriorating oxygen transport chain. Surprisingly, Ox66™ had similar efficacy between the two models in delivering oxygen to the peripheral spinotrapezius muscle, which was almost identical to raising FiO2 from 21% to 40%.
All groups’ pO2/FiO2 ratios met the criteria for mild ARDS [300–200] 60 min after LPS dosage and the onset of 40% tidal volume reduction, whereas breathing restriction alone in the single hit study produced ratios in the ∼350s [Citation18]. From experience, and as we argued previously (see discussion in [Citation18]), human pO2/FiO2 ratios may underestimate severity for smaller animals due to higher metabolisms (much like pharmacological dosing). Additionally, centrally-drawn PAO2 may be influenced by blood shunting to critical organs and therefore not be reflective of effective oxygen delivery to peripheral tissues. This insensitivity is supported by study findings where PAO2 and SO2 only registered with the 40% FiO2 treatment. PISFO2 in the peripheral capitally beds responded to both Ox66™ and 40% FiO2. Since PISFO2 evaluated end-stage gas exchange with the interstitium – the proximal site of oxygen metabolism by respiring cells – it provided a better read on effective oxygen delivery.
The insensitivity of PAO2 to Ox66™-driven changes to PISFO2 is interesting. On the surface, it suggests that two gavages of Ox66™ delivered a similar amount of oxygen to peripheral capillary beds as 40% FiO2. Further, it supports a theorized mechanism of Ox66™ having selective oxygen release in hypoxic regions, akin to the reduced p50s of haemoglobin-based oxygen carriers. More than simply driving force across a concentration gradient, it is believed that the low pH of the stomach and anaerobic tissues speeds clathrate release of O2. While some O2 is released in the digestive tract and absorbed across the gastric/intestinal wall, intact claterates are also believed to transit into the blood. When the clathrate moves through higher oxygenation/pH regions, the O2 – per this theory – stays within the claterates, which would prevent the partial pressure of oxygen reporting from central blood samples. It also may explain how a relatively small amount of added oxygen (per Ox66™) produced the significant systemic effects of this study. A targeted therapeutic that can act specifically and selectively on its intended site is desirable because it requires a lower overall systemic dose than one that may invest systemically. This working theory of clathrate release kinetics is supposition from the data and consultation with the manufacturer. In vitro characterization studies are planned to support future in vivo hypoxia models.
A glance at the inflammatory heat map in suggests O2 was associated with a progressive reduction in response to LPS + tidal volume restriction. The 40% FiO2 treatment delivered the most oxygen, as evidenced by hyperoxaemia (), and showed the lowest overall levels of cytokines and vascular growth factors. The amount of O2 delivered by Ox66™ gavage was lower and corresponded to a middle ground of inflammatory expression between NS and 40% FiO2. General inflammatory, T-cell, and hypoxic signalling were reduced by Ox66™, which supports the theory that Ox66™’s oxygen was released in deep, hypoxic tissues. For translational relevance, the elevations in IL-1β, TNF-α and IL-6 in this study are also seen in both bronchoalveolar lavage fluid and circulating plasma in ARDS subjects [Citation29]. Thus, the O2-associated reductions of IL-1b and IL-6 and the return of TNF-A to BL appear relevant to the human condition. Still, since O2 and Ox66™ have no direct immunomodulatory mechanism that we presently understand, we can only implicate relief of hypoxia for the reduction in cytokine signalling. Further, Ox66™ does not appear to be a significant source or stimulator of reactive oxygen species ROS, which could be evident through systemic vasoconstriction – not observed in our previous report of Ox66™ gavage [Citation18] or direct IV administration [Citation27] – in response to nitric oxide scavenging. Additionally, ROS tends to increase TNFα and IL-1β, which both decreased in the Ox66™ group. Indicators of neutrophil and macrophage activity did rise slightly for Ox66™ indicating that additional studies are needed to assess whether the Ox66™ clathrates activate these innate immune cells.
Ox66™ as a viable therapeutic for ARDS and other forms of clinical hypoxia is thus far encouraging. The manufacturer reports that production is straightforward and scalable to meet global needs. Our work suggests it is well-positioned as an additive to existing fluid treatments and vehicles, which may lead to numerous indications. With regards to ARDS, it is unclear if Ox66™ gavage can function as a stand-alone therapy when increased FiO2 and ventilation are indicated. Given that it delivered sufficient oxygen to improve PISFO2 and inflammatory markers, it may at least complement other therapies. Especially when the pulmonary route of O2 administration is compromised.
Conclusion
The results here indicate that orally administered Ox66™ delivers therapeutically relevant oxygenation to capillary beds improving tissue oxygenation and, by extension, reducing inflammatory signalling. Ox66™’s oxygen appeared to be released selectively at sites of tissue hypoxia, which magnified efficacy without risk of hyperoxic injury. Gavage also left the respiratory pathway accessible for other interventions if necessary. Since a more significant effect was associated with the higher oxygen content of the 40% FiO2 group, Ox66™ appears suited to a complementary role in severe situations of pulmonary dysfunction requiring ventilatory support. Further research is compelled on the nature of oxygen offloading from Ox66™ and whether gavage could be useful in reducing the faction of inspired O2 during ventilation to reduce the risk of injury.
Authorship contributions
Bjorn Song: Study design, data analysis, data collection, writing.
Danuel Carr: Data collection, writing.
Erica Bruce: Study design, data analysis, writing.
William Nugent: Study design, data analysis, writing.
Acknowledgements
None.
Disclosure statement
The authors report no conflict of interest.
Additional information
Funding
References
- Cochi SE, Kempker JA, Annangi S, et al. Mortality trends of acute respiratory distress syndrome in the United States from 1999 to 2013. Ann Am Thorac Soc. 2016;13(10):1742–1751. doi: 10.1513/AnnalsATS.201512-841OC.
- Yang X, Yu Y, Xu J, et al. Clinical course and outcomes of critically ill patients with SARS-CoV-2 pneumonia in Wuhan, China: a single-centered, retrospective, observational study. Lancet Respir Med. 2020;8(5):475–481. doi: 10.1016/S2213-2600(20)30079-5
- Han S, Mallampalli RK. The acute respiratory distress syndrome: from mechanism to translation. J Immunol. 2015;194(3):855–860. doi: 10.4049/jimmunol.1402513.
- Rawal G, Yadav S, Kumar R. Acute respiratory distress syndrome: an update and review. J Transl Int Med. 2018;6(2):74–77. doi: 10.1515/jtim-2016-0012.
- Radermacher P, Maggiore SM, Mercat A. Fifty years of research in ARDS. Gas exchange in acute respiratory distress syndrome. Am J Respir Crit Care Med. 2017;196(8):964–984. doi: 10.1164/rccm.201610-2156SO.
- Kallet RH, Zhuo H, Ho K, et al. Lung injury etiology and other factors influencing the relationship between dead-space fraction and mortality in ARDS. Respir Care. 2017;62(10):1241–1248. doi: 10.4187/respcare.05589.
- Cepkova M, Kapur V, Ren X, et al. Pulmonary dead space fraction and pulmonary artery systolic pressure as early predictors of clinical outcome in acute lung injury. Chest. 2007;132(3):836–842. doi: 10.1378/chest.07-0409.
- Kallet RH, Zhuo H, Liu KD, et al. National heart L, blood institute ANI. The association between physiologic dead-space fraction and mortality in subjects with ARDS enrolled in a prospective multi-center clinical trial. Respir Care. 2014;59(11):1611–1618. doi: 10.4187/respcare.02593.
- Nuckton TJ, Alonso JA, Kallet RH, et al. Pulmonary dead-space fraction as a risk factor for death in the acute respiratory distress syndrome. N Engl J Med. 2002;346(17):1281–1286. doi: 10.1056/NEJMoa012835.
- Determann RM, Royakkers A, Wolthuis EK, et al. Ventilation with lower tidal volumes as compared with conventional tidal volumes for patients without acute lung injury: a preventive randomized controlled trial. Crit Care. 2010;14(1):R1. doi: 10.1186/cc8230.
- Silva PL, Pelosi P, Rocco PRM. Supplemental oxygen or something else? J Thorac Dis. 2018;10(26):S3211–S3214. doi: 10.21037/jtd.2018.08.06.
- Guérin C, Reignier J, Richard J-C, et al. Prone positioning in severe acute respiratory distress syndrome. N Engl J Med. 2013;368(23):2159–2168. doi: 10.1056/NEJMoa1214103.
- Karagiannidis C, Brodie D, Strassmann S, et al. Extracorporeal membrane oxygenation: evolving epidemiology and mortality. Intensive Care Med. 2016;42(5):889–896. doi: 10.1007/s00134-016-4273-z.
- Combes A, Hajage D, Capellier G, et al. Extracorporeal membrane oxygenation for severe acute respiratory distress syndrome. N Engl J Med. 2018;378(21):1965–1975. doi: 10.1056/NEJMoa1800385.
- Rachmale S, Li G, Wilson G, et al. Practice of excessive F(IO(2)) and effect on pulmonary outcomes in mechanically ventilated patients with acute lung injury. Respir Care. 2012;57(11):1887–1893. doi: 10.4187/respcare.01696.
- Stapleton RD, Wang BM, Hudson LD, et al. Causes and timing of death in patients with ARDS. Chest. 2005;128(2):525–532. doi: 10.1378/chest.128.2.525.
- Zhang F, Aquino GV, Dabi A, et al. Oral ingestion of a novel oxygenating compound, Ox66, is non-toxic and has the potential to increase oxygenation. Food Chem Toxicol. 2019;125:217–224. doi: 10.1016/j.fct.2018.12.034.
- Nugent WH, Carr DA, MacBryde R, et al. Gavage approach to oxygen supplementation with oxygen therapeutic Ox66 in a hypoventilation rodent model of respiratory distress. Artif Cells Nanomed Biotechnol. 2021;49(1):709–716. doi: 10.1080/21691401.2021.2013251.
- Gray SD. Rat spinotrapezius muscle preparation for microscopic observation of the terminal vascular bed. Microvasc Res. 1973;5(3):395–400. doi: 10.1016/0026-2862(73)90055-1
- Golub AS, Pittman RN. Thermostatic animal platform for intravital microscopy of thin tissues. Microvasc Res. 2003;66(3):213–217. doi: 10.1016/s0026-2862(03)00061-x
- Nugent WH, Song BK, Pittman RN, et al. Simultaneous sampling of tissue oxygenation and oxygen consumption in skeletal muscle. Microvasc Res. 2016;105:15–22. doi: 10.1016/j.mvr.2015.12.007.
- Song BK, Nugent WH, Moon-Massat PF, et al. Effects of a hemoglobin-based oxygen carrier (HBOC-201) and derivatives with altered oxygen affinity and viscosity on systemic and microcirculatory variables in a top-load rat model. Microvasc Res. 2014;95:124–130. doi: 10.1016/j.mvr.2014.07.005.
- Nugent WH, Carr DA, Macko AR, et al. Physiological and microvascular responses to hemoglobin concentration-targeted hemolytic anemia in rats. J Appl Physiol (1985). 2020;128(6):1579–1586. doi: 10.1152/japplphysiol.00767.2019.
- Nugent WH, Jubin R, Buontempo PJ, et al. Microvascular and systemic responses to novel PEGylated carboxyhaemoglobin-based oxygen carrier in a rat model of vaso-occlusive crisis. Artif Cells Nanomed Biotechnol. 2019;47(1):95–103. 10.1080/21691401.2018.1543197
- Nugent WH, Sheppard FR, Dubick MA, et al. Microvascular and systemic impact of resuscitation with pegylated carboxyhemoglobin-based oxygen carrier or hetastarch in a rat model of transient hemorrhagic shock. Shock. 2019;53(4):493–502. doi: 10.1097/SHK.0000000000001370.
- Song BK, Light WR, Vandegriff KD, et al. Systemic and microvascular comparison of lactated ringer’s solution, VIR-HBOC, and alpha-alpha crosslinked haemoglobin-based oxygen carrier in a rat 10% topload model. Artif Cells Nanomed Biotechnol. 2020;48(1):1079–1088. doi: 10.1080/21691401.2020.1809441.
- Carr, D.A., Nugent, W.H., Bruce, E.D. and Song, B.K. 2022. Evaluation of an Injectable, Solid-State, Oxygen-Delivering Compound (Ox66) in a Rodent Model of Pulmonary Dysfunction-Induced Hypoxia. Mil. Med. 188, 1701.
- Beitler JR, Thompson BT, Baron RM, et al. Advancing precision medicine for acute respiratory distress syndrome. Lancet Respir Med. 2022;10(1):107–120. doi: 10.1016/S2213-2600(21)00157-0
- Meduri GU, Annane D, Chrousos GP, et al. Activation and regulation of systemic inflammation in ARDS: rationale for prolonged glucocorticoid therapy. Chest. 2009;136(6):1631–1643. doi: 10.1378/chest.08-2408.