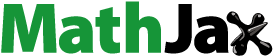
Abstract
Spherical gold/polyacrylic acid (Au/PAA) polymer–inorganic Janus nanoparticles (JNPs) with simultaneous therapeutic and targeting functions were fabricated. The obtained Au/PAA JNPs were further selectively functionalized with folic acid (FA) and thiol PEG amine (SH-PEG-NH2) on Au sides to provide superior biocompatibility and active targeting, while the other PAA sides were loaded with 5-aminolevulinic acid (5-ALA) to serve as a photosensitizer (PS) for photodynamic therapeutic (PDT) effects on MCF-7 cancer cells. The PS loading of 5-ALA was found to be 83% with an average hydrodynamic size and z-potential of 146 ± 0.8 nm and −6.40 mV respectively for FA-Au/PAA-ALA JNPs. The in vitro PDT study of the JNPs on MCF-7 breast cancer cells under 636 nm laser irradiation indicated the cell viability of 24.7% ± 0.5 for FA-Au/PAA-ALA JNPs at the IC50 value of 0.125 mM. In this regard, the actively targeted FA-Au/PAA-ALA JNPs treatment holds great potential for tumour therapy with high cancer cell-killing efficacy.
Introduction
Janus nanoparticles (JNPs), a special class of colloids with significant physicochemical properties, have come to light for various biological applications. They possess two or more nanocomponents with different physicochemical characterizations in one single particle, allowing the simultaneous realization of multiple functions [Citation1]. These differences pertain to optical, chemical, electrical, and magnetic properties [Citation2]. In addition, they offer easy and selective functionalization of each side, enabling simultaneous therapeutics and diagnostics applications [Citation3].
One of the promising applications of the JNPs is in drug delivery. Generally, when different functions such as cell targeting and imaging are on the same surface of NPs, they may interfere with each other. Therefore, JNPs can unite in a single structural unit by especially separating on two sides of the JNPs [Citation4,Citation5].
JNPs are divided into different categories including polymer–inorganic, polymer–polymer, and inorganic–inorganic hybrid particles with different morphologies such as mushroom-like, dumbbell-like, half-raspberry-like, acorn-like, and snowman-like [Citation6,Citation7]. Compared to polymer–polymer and inorganic–inorganic JNPs, polymer–inorganic JNPs can provide great advantages of different complementary chemistries concerning both hemispheres, which enables the integration of different functions for biomedical applications [Citation7]. The main strategy to synthesize polymeric-inorganic JNPs is surface nucleation/seeded growth which allows the second material to grow on the surface of one material [Citation8]. The inorganic side (e.g. gold, TiO2, CuS, and Pb) can provide the conjugation of active cell targeting (such as FA–PEG–SH), while the drug is attached to the polymeric side. JNPs are considered potential ‘second-generation NPs’ for a combination of multiple treatments in cancer research. They offer the advantage of heterogeneous and distinct structures for the development of multifunctional nanomedicines [Citation8,Citation9].
Phototherapy such as photodynamic therapy (PDT) utilizes photosensitizers (PSs) to produce singlet oxygen species to obliterate tumour cells upon laser irradiation. It is noted that phototherapeutic agents provide low toxicity in the dark with high selectivity relative to chemotherapeutic agents [Citation10].
The application of JNPs together with PDT can improve the synergy effect of treatment modalities which is an intriguing research field. In this sense, we present the fabrication of a facile and selective growth strategy for synthesizing uniform bifunctional FA-Au/PAA-ALA JNPs. As illustrated in Scheme 1, the procedure for the controlled synthesis of concentric and eccentric Au/PAA NPs consists of the preparation of Au NPs followed by the addition of polyacrylic acid (PAA) to form concentric core/shell Au/PAA NPs. The reversible structural transformation of nanostructures from concentricity to eccentricity can then be achieved by introducing different amounts of isopropyl alcohol (IPA). The concentric Au/PAA NPs are formed when the ratio of water to IPA is 1:4 and interestingly by tuning the ratio of water: IPA to 1:9, the Au NPs move towards the edge of the PAA shell to form eccentric Au/PAA JNPs [Citation11,Citation12].
This reversible structural transformation process can be explained thermodynamically with the Gibbs free energy per surface area at a constant temperature and pressure which is equal to the surface tension since a negative Gibbs free energy controls a spontaneous process [Citation11]. Therefore, the change of the interfacial energy of σAu−PAA, σAu−solvent, and σPAA−solvent makes the spreading coefficient (–ΔG = S = σAu−solvent − (σAu−PAA + σPAA−solvent)) lower than 0, as the σAu-solvent decreases more than σPAA-solvent resulting in the gradual move of the Au NPs to the edge of PAA shell [Citation11,Citation13].
The eccentric Au/PAA core/shell NPs are a good example to investigate effective drug delivery carriers with high loading capacity for 5-aminolevulinic acid (5-ALA). Therefore, Au NPs on the one side of eccentric core/shell nanostructures were functionalized selectively with folic acid (FA) as a targeting agent and PAA on the other hemisphere attached to 5-ALA PS to investigate the possibility of enhanced PDT within in vitro breast cancer cultured cells via active targeting drug delivery.
Although PAA has been approved by the Food and Drug Administration (FDA) which provides great advantages in cancer treatment, biosafety concerns of inorganic materials (e.g. Au and Ag) have limited their approval for clinical use so this work is a proof-of-concept study. Furthermore, long-term bio-toxicity, pharmacokinetics, elimination mechanisms, and biodistributions of the JNPs should be comprehensively investigated on different cancer cells to introduce them to clinical cancer diagnosis and treatment.
Materials and methods
Materials
Gold (III) chloride trihydrate (HAuCl4.3H2O), tri-sodium citrate, tannic acid (ACS reagent), polyacrylic acid (PAA), 5-aminolevulinic acid hydrochloride (5-ALA·HCl, ≥98%), N-ethyl-N′-(3-dimethyl aminopropyl) carbodiimide (EDC, BioXtra), N-hydroxysuccinimide (NHS, 98%), SH-PEG2k-NH2 (HCl salt, average Mn 2,000), folic acid (FA ≥97%), dimethyl sulphoxide (DMSO, anhydrous, ≥99.9%), acetone (HPLC ≥99.8%) and 2-(N-morpholino) ethanesulphonic acid buffer (MES) and phosphate-buffered saline (PBS, 10× concentrate) buffer were purchased from Sigma Aldrich. Isopropyl alcohol (IPA, ACS reagent) and ammonium solution (28-30%) were supplied from Merck. Aqua regia solution was used to wash all glassware and Millipore water was used throughout this study.
Fabrication of FA-Au/PAA-ALA JNPs
Synthesis of gold-Poly acrylic acid (Au/PAA) JNPs
Citrate-Au NPs with a size of ∼12 nm were synthesized by reducing 1 ml of 1% HAuCl4.3H2O with 79 ml MQ water and a 20 ml aqueous solution consisting of 4 ml of 1% tri-sodium citrate and 20 µL of tannic acid. The solution was then stirred for 30 min at ∼60 °C under a reflux system. The synthesized AuNPs were centrifuged and dispersed in Millipore water for further experiments. PAA (5 µL, 0.2 g/mL) was then mixed with 2 ml of AuNPs under sonication followed by the addition of 7.5 µL NH4OH (2.0 mol/l) and stirred for 10 min. 18 ml IPA was added to the mixture dropwise and stirred overnight to provide Au/PAA JNPs.
Attachment of 5-ALA to Au/PAA JNPs
To attach 5-ALA to the JNPs, carboxyl groups of the PAA were activated by an equivalent volume of 0.3 mol/l EDC and NHS (in 100 mmol/L MES buffer, pH = 5.5). 1 ml 0.3 mol/l 5-ALA was added to the mixture and then agitated for 2 h. Au/PAA-5-ALA was collected by centrifuging and dispersed in PBS.
The amount of the PS attached to the JNPs was determined by finding the difference in 5-ALA concentration in the solution before and after the attachment. The percentage of PS loading is calculated using the below equation.
where a and b represent the initial 5-ALA concentration and concentration of 5-ALA in the supernatant respectively.
Conjugation of FA to SH-PEG-NH2
FA was first conjugated to SH-PEG-NH2 to increase the flexibility of FA to recognize folate receptors on cancer cells. Therefore, carboxyl groups of 16 mg FA were activated with 10 mg EDC and 6.4 mg NHS in 1 ml DMSO and stirred overnight under a light-protected atmosphere. Afterward, 40 mg SH-PEG-NH2 was added to the mixture and stirred for a further 24 h followed by centrifuging in ice-cold acetone (−20 °C) to remove the dicyclohexylurea by-product. The FA-PEG-SH pellet was obtained by several steps of acetone washing and drying under a vacuum.
Attachment of FA-PEG-SH to Au/PAA-ALA JNPs
5 mg FA-PEG-SH was dissolved in 1 ml of a mixture of DMSO: PBS (1: 40 v/v) and mixed with 1 ml of Au/PAA-5-ALA JNPs and stirred for a few minutes to provide Au-S linkage. The resulting FA-PEG-Au/PAA-5-ALA JNPs were then purified with centrifugation to remove the excess FA and dispersed in PBS. The final actively targeted JNPs were referred to as FA-Au/PAA-ALA JNPs throughout the paper and Au/PAA-ALA JNPs were considered as passive JNPs.
The FA content attached to the JNPs was calculated by measuring the absorbance at 365 nm and comparing it with a calibration curve.
Physicochemical characterizations
All characterization techniques were performed by using the instrument explained in our previous paper [Citation14].
In vitro cell culture
MCF-7 breast cancer cells (American Type Cell Culture, ATCC, Manassas, VA, USA, ATCC HTB-22) were cultured as adherent monolayers in Dulbecco’s Modified Eagle’s Medium (DMEM), 10% (v/v) foetal bovine serum (FBS), 1% (v/v) penicillin/streptomycin and 1% (v/v) and amphotericin-β at 37 °C in a humidified atmosphere of 5% CO2, at approximately 85% confluency. Once MCF-7 monolayer cells were achieved, they were sub-cultured into 3.4 cm diameter cell culture plates at a seeding ratio of 1.0 × 105 cells/ml for in vitro cellular experiments.
Cellular uptake of the FA-Au/PAA-ALA JNPs
MCF-7 cells were seeded on sterile coverslips in 3.4 cm diameter and incubated for 4h at 37 °C for cellular attachment. The media was then replaced with serum-free media containing the PS or the JNPs followed by a further 20 h incubation in the dark [Citation14]. The cells were fixed with 4% paraformaldehyde and permeabilized with a permeabilization solution of 0.5% Triton X-100 in 1X PBS. In the next step, the cells were counterstained with 300 nM of 4′, 6-diamidino-2-phenylindole (DAPI) for 5 min and were observed on the Carl Zeiss Axio Observer Z1 (Live imaging microscope). All preparations were carried out at room temperature in the dark.
Photodynamic effect of FA-Au/PAA-ALA JNPs
The PDT effect of the 5-ALA, passive, and active JNPs was investigated in serum-free media [Citation14] using light irradiation at 10 J/cm2 doses with a semiconductor diode laser with an output power of 68 mW, light intensity of 7.5 mW/cm2 and the peak wavelength of 636 nm. After 4 h of incubation, as explained in section 2.4, the cells were subjected to various concentrations of 5-ALA and the JNPs and incubated for 20 h. Following incubation, the cells that required PDT treatment received laser light irradiation in PBS. All light irradiations were carried out in a minimum light exposure atmosphere to prevent any additional light inferring reactions. After irradiation, fresh medium was added followed by an additional 24-h incubation until the ATP viability assay was performed.
Determination of cellular ATP Levels-Viability study
Measurement of cellular adenosine triphosphate (ATP) levels for cell viability in control and experimental groups was performed using CellTiter-Glo® as explained in our previous paper [Citation14]. The luminescent signal of the cells was recorded in relative light units (RLUs) and true cell viability values were obtained by subtracting background luminescence values from experimental readings. Finally, the ATP viability assay was presented as a percentage relative to the cells only (control).
Detection of apoptosis using flow cytometry
The apoptosis cell death pathway of MCF-7 cells was evaluated using fluorescein isothiocyanate (FITC) Annexin V/propidium iodide (PI) kit (BD Scientific: BD/556570) via flow cytometry. Post 24 h PDT, the cells were stained using FITC–annexin V and PI in the dark, according to the manufacturer’s manual. The dead cells were discriminated based on forward- and side-scatter parameters; annexin V+/PI- was identified as early apoptotic while annexin V+/PI + showed late apoptotic cells. A sum of Q2 and Q4 quadrant populations of analysed cells was considered as the percentage of apoptotic cells.
Statistical analysis
Biochemical assays were conducted in triplicate, and error bars reported standard deviation. Student’s t-test and one-way ANOVA were employed in the 95% confidence interval (p < 0.05*, p < 0.01**, or p < 0.001***) to compare the significant difference between control and experimental groups.
Results and discussion
Fabrication and characterizations of FA-Au/PAA-ALA JNPs
The controlled fabrication of the JNPs is depicted in Scheme 1. Au NPs with the size of 12.0 nm ± 1.5 were mixed with PAA and ammonium hydroxide to obtain concentric Au/PAA core/shell NPs. With an increase of a mixture of water: IPA to a ratio of 1:9, the Au NPs move towards the edge of the PAA sphere, resulting in eccentric JNPs with a thickness of 26.4 nm ± 0.03 of the PAA side. Generally, a negative Gibbs free energy and the change of the interfacial energy between Au NPs, PAA, and solvent determine a spontaneous process of a transition from concentric to eccentric geometry [Citation11,Citation15]. As described in the introduction, the concentric core/shell NPs are formed at the ratio of 1:4 for water: IPA which hinges on the balance of the interfacial energies σ PAA-Au, σPAA-solvent, and σAu-solvent where σ surface tension is equal to the Gibbs free energy per surface area at a constant pressure and temperature [Citation11]. Therefore, with the introduction of IPA to concentric NPs to a ratio of 1:9, σAu-solvent decreases more than σPAA-solvent resulting in the moving of the Au NPs to the edge of the PAA shell while the addition of more water can cause the movement of Au NPS to the centre of PAA shell [Citation11].
PAA hemisphere can provide an abundance of carboxyl groups to load the positively charged drugs by electrostatic interactions or attach the amino groups of the drugs via amide interactions (EDC/NHS reaction) [Citation16]. Moreover, due to the swelling capacity and porous structure of the PAA shell, more PS can be incorporated into the NPs [Citation11]. In this regard, the percentage of the 5-ALA loading was calculated to be 83.0% ± 2.5 or 0.25 mol L−1. The prepared Au/PAA-ALA JNPs were then selectively functionalised with SH-PEG-NH2 and FA on the Au hemisphere for cancer cell-specific targeting while the other hemisphere acted as PS delivery and PDT agent. It should be noted that due to the absence of surfactant during the synthesis of Au NPs, their surface is available for Au-S linkage with SH-PEG-FA where SH-PEG-FA was prepared via an amide linkage between the carboxyl groups of FA and amino groups of SH-PEG-NH2. The content of FA in FA-Au/PAA-ALA JNPs based on the regression equation was determined to be 2.14 mg mL−1.
The fabrication of the NPs and JNPs was monitored by using UV/Vis absorption spectroscopy. The LSPR peak of the citrate Au NPs and the Soret absorption peak in the spectrum of free 5-ALA appeared at 525 nm and 261 nm respectively (, insets) which can be observed in the spectra of the synthesised JNPs (). The broadening of the LSPR peak after the addition of PAA to form the JNPs in can be attributed to a heterogenous growth pattern and a polydisperse particle morphology [Citation17]. A red shift in the plasmonic band of the JNPs compared to Au NPs is due to coating with other molecules or agglomeration of the NPs during the purification process [Citation18] as well as a change in the local dielectric function of their surrounding medium [Citation19]. Additionally, a broadening and slight red shift of the LSPR and the Soret band of 5-ALA were indicative of the strong interaction of 5-ALA with the JNPs [Citation20]. The incorporation of FA in the spectrum of the FA-Au/PAA-FA JNPs was proved with distinguished absorption maxima at 365 nm.
Figure 1. Intercellular localization of the free 5-ALA, passive Au/PAA-ALA, and final active FA-Au/PAA-ALA JNPs in MCF-7 cells under an equivalent concentration of 5-ALA.
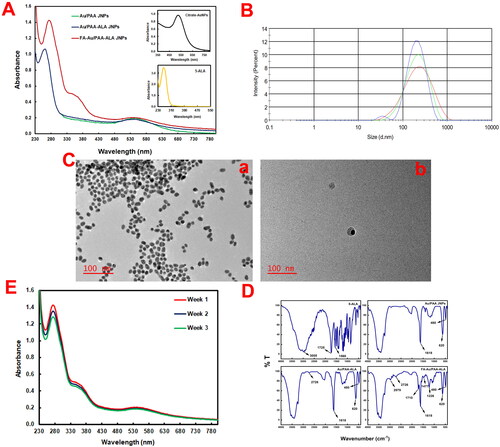
The average hydrodynamic size, polydispersity index (PDI), and ζ-potential of FA-Au/PAA-ALA JNPs in PBS were also measured to be 146 ± 0.8 nm (), 0.33, and −6.40 mV respectively. Citrate capped-Au NPs with the size of 12.0 nm ± 1.5 ( (a)) with high stability due to the presence of sodium citrate were synthesised according to a published method [Citation21]. Au/PAA JNPs were then fabricated via a mild and facile route with an average size of ∼ 38 nm. (b) indicates the successful formation of the Janus structure.
Figure 2. Characterizations of the JNPs. (A) UV/Vis spectra of Au/PAA, Au/PAA-ALA, and FA-Au/PAA-ALA JNPs (insets show UV/Vis spectra of the citrate-Au NPs and 5-ALA), (B) the average hydrodynamic size of FA-Au/PAA-ALA JNPs using DLS, (C) TEM images of (a) citrate-Au NPs and (b) Au/PAA JNPs, (D) FTIR spectra of 5-ALA, Au/PAA, Au/PAA-ALA, and FA-Au/PAA-ALA JNPs, (E) photostability of the final actively targeted FA-Au/PAA-ALA JNPs in PBS over three weeks.
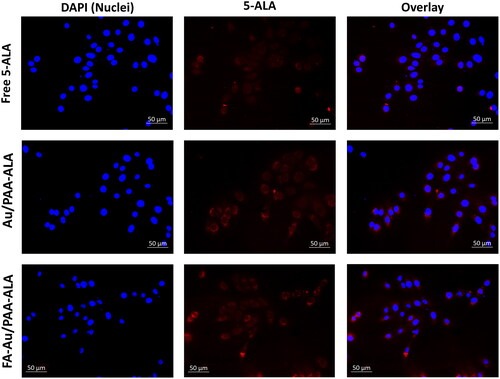
illustrates the FTIR spectra of 5-ALA, Au/PAA, Au/PAA-ALA, and FA-Au/PAA-ALA JNPs. The peak in Au/PAA at 1618 cm−1 was attributed to the carboxylic groups of the PAA and confirmed the formation of the JNPs. Further, the bands at 620 cm−1 and 480 cm−1 appeared in all NPs were pertained to gold bonds. FTIR spectra of the FA-Au/PAA-ALA JNPs showed that FA was attached to the surface of the PAA side through amide linkage between carboxyl groups of the PAA and amino groups of FA which made a strong absorption peak around 1710 cm−1 related to amide-carbonyl (-NH-CO-) stretching vibrations. The phenyl ring of folic acid was evident from the band at 1477 cm−1. The presence of HS-PEG-NH2 on the surface of the Au side was confirmed by the band at 1226 cm−1 attributable to C-N stretching vibrations. Additionally, FA-modified PEG showed a band at 2979 cm−1 corresponding to -C-H- symmetric stretching vibration [Citation22].
ALA molecules tend to make dimer by hydrogen bonds between O-H of one molecule and C = O of another ALA to form O-H….C = O. Therefore, the peak at 2726 cm−1 in the spectra of Au/PAA-ALA and FA-Au/PAA-ALA is associated with the coupled C-O stretching and O-H and confirms the presence of ALA [Citation23].
The photostability of the final JNPs was also tested over 3 weeks (). The LSPR band of the JNPs and peak of FA remained at ∼542 nm and 365 nm respectively with no significant changes. A negligible decrease was only noted on the Soret band of 5-ALA. The selective modification with PEG on the Au hemisphere also enhanced their stability and biocompatibility.
Intercellular localization of FA-Au/PAA-FA JNPs
To evaluate the internalisation, MCF-7 cancer cells were accumulated with the JNPs under an equivalent concentration of the 5-ALA. As shown in , the red fluorescence of 5-ALA within the treated cells was monitored with free PS, passive, and active JNPs. It was observed that the actively targeted JNPs could readily be internalised by MCF-7 compared with either the PS or the passive JNPs. Although the accumulation of the passive JNPs demonstrated the same tendency, the intracellular 5-ALA fluorescence in the FA-Au/PAA-ALA JNPs group was brighter than in the Au/PAA-ALA group.
Photodynamic effect of FA-Au/PAA-FA JNPs
The cells were exposed to varying concentrations of the free PS, final FA-Au/PAA-FA JNPs, and the IC50 value of the passive Au/PAA-FA JNPs and were then irradiated at a wavelength of 636 nm using a fluence of 10 J/cm2 (7.5 mW/cm2). As can be observed in , no morphological changes in MCF-7 cells were noted for free PS, passive, or active JNPs under the equivalent concentration of 5-ALA with no irradiation confirming no phototoxicity or dark toxicity. While the cells were treated with 5-ALA, passive and active targeting JNPs under laser irradiation induced detachment, rounding up of the cells, and loss of cellular shape. However, the cells treated with the actively targeted JNPs-mediated PDT demonstrated the most significant changes in cell morphology indicative of non-viable cells.
In vitro ATP viability against MCF-7 cells
The potential viability of the free PS, passive, and actively targeted JNPs was investigated on MCF-7 cells by ATP cell viability assay. As illustrated in , the cell viability for MCF-7 cells can reach ∼99% after incubating with either PS, passive, or active JNPs under no irradiation for 20 h even at a high dosage (2.0 mM). These results, therefore, revealed that the JNPs had great biocompatibility and weak ROS generation capacity upon laser Irradiation for further applications.
Based on , the IC50 value of free 5-ALA was determined as 0.25 mM at a fluence of 10 J/cm2 which was consistent with our previous results where the IC50 value of free 5-ALA was found to be 0.5 mM at a fluence of 5 J/cm2 [Citation14]. Therefore, it was concluded that the more applied fluence, the lower the IC50 value. To evaluate the therapeutic effect of the JNPs plus laser irradiation, MCF-7 cells were treated with IC50 value of the passive and various concentrations of the active JNPs. In contrast to the free PS, passive Au/PAA-FA JNPs plus laser irradiation had higher cell-killing ability due to the good capability of the nanosystem to carry the PS to the vicinity of cancer cells. More importantly, the lowest cell viability in the FA-Au/PAA-ALA JNPs group under laser irradiation was achieved among all experimental groups. In this sense, the IC50 value reached 0.125 mM for Au/PAA-ALA (34.6% ± 0.2) and FA-Au/PAA-ALA JNPs (24.7% ± 0.5). In addition, under the same laser conditions, the cell viability of the FA-Au/PAA-ALA JNPs at the 5-ALA concentration of 0.125 mM was close to that of the free 5-ALA group at the 5-ALA concentration of 0.25 mM.
Effect of the JNPs on apoptosis using flow cytometry
Flow cytometry was applied to evaluate the cell death mechanism on the treated MCF-7 cells with the free PS and JNPs. shows the results of cell apoptosis after different treatments. Compared to the free 5-ALA treated cells under laser irradiation, the cells that received an equivalent concentration of 5-ALA in the passive Au/PAA-ALA showed a higher proportion of apoptotic cells (early apoptosis 53.9% and late apoptosis 8.1% for 0.25 mM 5-ALA). However, MCF-7 cells treated with the final active FA-Au/PAA-ALA JNPs provided an elevated level of apoptosis cells relative to the free PS and the passive JNPs plus irradiation (early apoptosis 62.6% and late apoptosis 5.8% for 0.25 mM 5-ALA). These preliminary results were indicative of the effect of FA to selectively target the folate receptors that are overexpressed on the MCF-7 cells. According to these results, apoptosis was the primary cause of cell death in MC-7 cells, while necrosis was negligible. Loss of membrane and release of intercellular content is associated with the necrosis cell death pathway which can cause harmful inflammatory and immunogenic responses and is not an undesirable pathway for cell death [Citation24].
Conclusions
In summary, the synthesis of a JNP was investigated containing Au NPs at one side and PAA at the opposite side via a selective growth strategy. In the resultant Au/PAA JNPs, the exposed Au domain was selectively functionalized with FA-PEG-SH to achieve long circulation time and biocompatibility, as well as cancer cell-specific targeting while the polymeric domain of the JNPs allows the loading of 5-ALA PS for photodynamic therapeutic effects. Due to integrating different materials in the one-particle unit, FA-Au/PAA-ALA JNPs had more capacity to offer different functionality diversity affecting each other. Besides of the biocompatibility and cell active targeting of Au domains with FA-PEG-SH, the obtained JNPs endow high PS loading for outstanding PDT of breast cancer cells. The viability analysis noted that the final JNPs had no obvious in vitro dark toxicity with high biocompatibility. This preliminary study highlighted the potential of Au/PAA JNPs as a realizable tool for efficient cancer photodynamic therapy.
Author contributions
Hanieh Montaseri made substantial contributions to the conception or design of the work and interpretation of data for the work. Hanieh Montaseri and Heidi Abrahamse were involved in the acquisition, analysis, or interpretation of data for the work. Hanieh Montaseri and Heidi Abrahamse drafted the work and revised it critically for important intellectual content and finally approved the version to be published. Hanieh Montaseri and Heidi Abrahamse agree to be accountable for all aspects of the work in ensuring that questions related to the accuracy or integrity of any part of the work are appropriately investigated and resolved.
Acknowledgments
The authors sincerely thank the University of Johannesburg, the National Laser Centre, and the National Research Foundation–South African Research Chairs Initiative (NRFSARChI) for their financial grant support. The authors sincerely thank the University of Johannesburg, the National Laser Centre, and the University of Johannesburg GES 4.0 PDF Fellowship for their financial grant support.
Disclosure statement
No potential conflict of interest was reported by the author(s).
Data availability statement
The datasets generated during and/or analysed during the current study are available from the corresponding author upon request.
Additional information
Funding
References
- Walther A, Müller AH. Janus particles. Soft Matter. 2008;4(4):663–668. doi: 10.1039/b718131k.[PMC]w
- Yi Y, Sanchez L, Gao Y, et al. Janus particles for biological imaging and sensing. Analyst. 2016;141(12):3526–3539. doi: 10.1039/c6an00325g.
- Shin T-H, Choi Y, Kim S, et al. Recent advances in magnetic nanoparticle-based multi-modal imaging. Chem Soc Rev. 2015;44(14):4501–4516. doi: 10.1039/c4cs00345d.
- Hu S-H, Gao X. Nanocomposites with spatially separated functionalities for combined imaging and magnetolytic therapy. J Am Chem Soc. 2010;132(21):7234–7237. doi: 10.1021/ja102489q.
- Wang F, Pauletti GM, Wang J, et al. Dual surface‐functionalized janus nanocomposites of polystyrene/Fe3O4@SiO2 for simultaneous tumor cell targeting and stimulus‐induced drug release. Adv Mater. 2013;25(25):3485–3489. doi: 10.1002/adma.201301376.
- Bradley LC, Stebe KJ, Lee D. Clickable janus particles. J Am Chem Soc. 2016;138(36):11437–11440. doi: 10.1021/jacs.6b05633.
- Zhang M, Zhang L, Chen Y, et al. Precise synthesis of unique polydopamine/mesoporous calcium phosphate hollow janus nanoparticles for imaging-guided chemo-photothermal synergistic therapy. Chem Sci. 2017;8(12):8067–8077. doi: 10.1039/c7sc03521g.
- Zhang Y, Huang K, Lin J, et al. Janus nanoparticles in cancer diagnosis, therapy and theranostics. Biomater Sci. 2019;7(4):1262–1275. doi: 10.1039/c8bm01523f.
- Zhang H, et al. Galvanic displacement synthesis of monodisperse janus‐and satellite‐like plasmonic–magnetic Ag–Fe@Fe3O4 heterostructures with reduced cytotoxicity. Adv Sci. 2018;5(8):1800271.
- Cheng L, Wang C, Feng L, et al. Functional nanomaterials for phototherapies of cancer. Chem Rev. 2014;114(21):10869–10939. doi: 10.1021/cr400532z.
- Li L, Zhang L, Xing S, et al. Generalized approach to the synthesis of reversible concentric and eccentric polymer-coated nanostructures. Small. 2013;9(6):825–830. doi: 10.1002/smll.201201735.
- Li L, Liu C, Zhang L, et al. Multifunctional magnetic–fluorescent eccentric-(concentric-Fe3O4@SiO2)@ polyacrylic acid core–shell nanocomposites for cell imaging and pH-responsive drug delivery. Nanoscale. 2013;5(6):2249–2253. doi: 10.1039/c3nr33695f.
- Torza S, Mason S. Three-phase interactions in shear and electrical fields. J Colloid Interface Sci. 1970;33(1):67–83. doi: 10.1016/0021-9797(70)90073-1.
- Montaseri H, Kruger CA, Abrahamse H. Targeted photodynamic therapy using alloyed Nanoparticle-Conjugated 5-aminolevulinic acid for breast cancer. Pharmaceutics. 2021;13(9):1375. doi: 10.3390/pharmaceutics13091375.
- Xing S, Feng Y, Tay YY, et al. Reducing the symmetry of bimetallic Au@ Ag nanoparticles by exploiting eccentric polymer shells. J Am Chem Soc. 2010;132(28):9537–9539. doi: 10.1021/ja102591z.
- Santra S, Kaittanis C, Grimm J, et al. Drug/dye‐loaded, multifunctional iron oxide nanoparticles for combined targeted cancer therapy and dual optical/magnetic resonance imaging. Small. 2009;5(16):1862–1868. doi: 10.1002/smll.200900389.
- Oluwafemi OS, Revaprasadu N, Adeyemi OO. A facile “green” synthesis of ascorbic acid-capped ZnSe nanoparticles. Colloids Surf B Biointerfaces. 2010;79(1):126–130. doi: 10.1016/j.colsurfb.2010.03.040.
- Bera K, Maiti S, Maity M, et al. Porphyrin–gold nanomaterial for efficient drug delivery to cancerous cells. ACS Omega. 2018;3(4):4602–4619. doi: 10.1021/acsomega.8b00419.
- Zhang Q, Zhang L, Li S, et al. Designed synthesis of Au/Fe3O4 @C janus nanoparticles for dual-modal imaging and actively targeted chemo-photothermal synergistic therapy of cancer cells. Chemistry. 2017;23(68):17242–17248. doi: 10.1002/chem.201703498.
- Shaikh AJ, Rabbani F, Sherazi TA, et al. Binding strength of porphyrin − gold nanoparticle hybrids based on number and type of linker moieties and a simple method to calculate inner filter effects of gold nanoparticles using fluorescence spectroscopy. J Phys Chem A. 2015;119(7):1108–1116. doi: 10.1021/jp510924n.
- Slot J, Geuze H. A method to prepare isodisperse colloidal gold sols in the size range 3–17 nm. Ultramicroscopy. 1984;15(4):383. doi: 10.1016/0304-3991(84)90144-X.
- Venkatasubbu GD, Ramasamy S, Avadhani GS, et al. Surface modification and paclitaxel drug delivery of folic acid modified polyethylene glycol functionalized hydroxyapatite nanoparticles. Powder Technol. 2013;235:437–442. doi: 10.1016/j.powtec.2012.11.003.
- Chen J, Peng Q, Jodl HJ. Infrared spectral comparison of 5-aminolevulinic acid and its hexyl ester. Spectrochim Acta A Mol Biomol Spectrosc. 2003;59(11):2571–2576. doi: 10.1016/s1386-1425(03)00011-8.
- Melamed JR, Edelstein RS, Day ES. Elucidating the fundamental mechanisms of cell death triggered by photothermal therapy. ACS Nano. 2015;9(1):6–11. doi: 10.1021/acsnano.5b00021.