ABSTRACT
The persistence and clinical consequences of rabies virus (RABV) infection have prompted global efforts to develop a safe and effective vaccines against rabies. mRNA vaccines represent a promising option against emerging and re-emerging infectious diseases, gaining particular interest since the outbreak of COVID-19. Herein, we report the development of a highly efficacious rabies mRNA vaccine composed of sequence-modified mRNA encoding RABV glycoprotein (RABV-G) packaged in core–shell structured lipopolyplex (LPP) nanoparticles, named LPP-mRNA-G. The bilayer structure of LPP improves protection and delivery of RABV-G mRNA and allows gradual release of mRNA molecules as the polymer degrades. The unique core–shell structured nanoparticle of LPP-mRNA-G facilitates vaccine uptake and demonstrates a desirable biodistribution pattern with low liver targeting upon intramuscular immunization. Single administration of low-dose LPP-mRNA-G in mice elicited potent humoral immune response and provided complete protection against intracerebral challenge with lethal RABV. Similarly, single immunization of low-dose LPP-mRNA-G induced high levels of virus-neutralizing antibody titers in dogs. Collectively, our data demonstrate the potential of LPP-mRNA-G as a promising next-generation rabies vaccine used in human and companion animals.
Introduction
Rabies is a neurological disease caused by RABV with a mortality rate approaching 100%. Due to its zoonotic nature, rabies remains widely prevalent in more than 150 countries, particularly developing countries [Citation1–4]. Approximately 17 million people are vaccinated and treated each year after exposure to RABV [Citation5]. However, RABV still causes about 60,000 deaths per year worldwide, with over 150 countries and half of the population at risk of exposure to the RABV [Citation6–8]. In most cases, rabies transmits through bites by rabid dogs [Citation9–11]. Massive vaccination of stray dogs in developing countries is considered a promising and effective method of control rabies and there is increasing consensus regarding the effectiveness of this approach [Citation8]. Therefore, it is of great significance to develop a safe, effective, and economical rabies vaccines for controlling and preventing rabies [Citation12, Citation13].
mRNA vaccines have the advantages of easy design, short production cycles, and high ability to induce both humoral and cellular immunity, with the efficacy of mRNA vaccines having recently been demonstrated by the COVID-19 mRNA vaccine [Citation14–16]. mRNA vaccines contain mRNA encoding disease-specific antigens that are synthesized by host cells, thus triggering immune response [Citation17]. Accordingly, there is considerable interest in the rapid development of mRNA vaccines against emerging infectious diseases [Citation18, Citation19]. Further, there is an almost negligible risk of mRNA integration into the host genome, thus providing a safe and controllable pattern of gene expression [Citation20]. However, bare mRNA molecules are unable to directly cross the cell membrane barrier, impeding the efficiency of cytoplasmic mRNA delivery systems. Current mRNA vaccines are constrained by inherent instability and suboptimal thermostability following LNP encapsulation, which diminishes the immunogenicity of mRNA vaccines [Citation21–24]. Effective mRNA delivery systems that overcome intracellular internalization and endosome escape are required to achieve effective mRNA therapy [Citation25].
One method of delivering mRNA vaccines is direct inoculation of mRNA molecules packaged in lipid nanoparticles (LNP), which are absorbed by antigen-presenting cells [Citation26, Citation27]. Recent studies of LNP have demonstrated rapid distribution throughout the body and liver following intramuscular administration [Citation27, Citation28]. The excessive accumulation of mRNA vaccines in the liver may induce potential side effects, and exploring the targeted expression of mRNA in vivo is considered to be the key point of the next-generation mRNA vaccines [Citation29, Citation30]. Herein, we used a core–shell structured LPP platform for mRNA vaccine production. The LPP platform packages mRNA molecules into a polymer multi-complex core loaded into a phospholipid bilayer structure. The obtained nanoparticles protect mRNA molecules in the complex core from RNase degradation that increases stability and allows release of mRNA with gradual dissociation of the polymer/mRNA complex [Citation31].
RABV-G, as the only surface glycoprotein of RABV, is predominantly responsible for inducing high-efficiency virus-neutralizing antibodies [Citation32]. According to reports, Bai and co-workers have recently developed a single-dose nucleoside-modified rabies mRNA-LNP vaccine encoding RABV-G, which can induce potent and persistent immune response [Citation33]. Different from previous approaches, in this study, we developed a rabies mRNA vaccine expressing RABV-G based on the LPP system. This mRNA vaccine contains a linear single-stranded RNA consisting of a 5’ cap, the untranslated region (UTR), the antigen-coding region, and a 3’ poly(A) tail and is delivered via LPP encapsulation. Intramuscular administration of LPP-mRNA-G demonstrated a favourable biodistribution pattern with limited accumulation in the liver or other major organs, thus indicating a benign and desirable toxicity profile. The immunogenicity and protective effects of this mRNA vaccine have also been evaluated in mice and dogs, which indicate that LPP-mRNA-G may represent a promising candidate as an efficacious rabies vaccine.
Materials and methods
Cells, viruses, reagents, and animals
HEK-293 T and BSR cells were purchased from the National Collection of Authenticated Cell Cultures (Shanghai, China). Adherent cells were cultured in DMEM supplemented with 1% penicillin–streptomycin (Thermo Fisher) and 10% FBS (Thermo Fisher). The RABV challenge virus strain (CVS), CVS-11 and CVS-24, was maintained in our lab as previously described [Citation34, Citation35]. Antibodies with direct-labelled fluorescein for flow cytometric analyses were purchased from BioLegend (CA, USA). FITC anti-mouse CD4 antibody (RRID:AB_312713), APC anti-mouse CD185 (CXCR5) antibody (RRID:AB_2561970), and PE anti-mouse CD279 (PD1) antibody (BioLegend, Cat.no.135206) were used as markers of T follicular helper (Tfh) cells in inguinal lymph nodes (LNs); FITC anti-mouse CD45R/B220 antibody (RRID:AB_312991), 647 anti-mouse GL7 antibody (BioLegend, Cat.no.144606), and PE anti-mouse CD95 antibody (BioLegend, Cat.no.554295) were used as markers of GC B cells in LNs; FITC anti-mouse CD45R/B220 antibody (RRID:AB_312991) and APC anti-mouse CD138 (Syndecan-1) antibody (RRID:AB_10962911) were used to analyse the number of PCs in BMs. HRP-labelled goat anti-mouse IgG (RRID:AB_2904507), IgG1 (RRID:AB_10695944), IgG2a (RRID:AB_10680049), and IgG2b (RRID:AB_10695945) for ELISA. HRP-labelled goat anti-dog IgG (RRID:AB_2927648) for ELISA. Anti-RABV-G monoclonal antibody and anti-RABV-P monoclonal antibody was prepared in our lab as previously described [Citation35–38]. The licensed commercial inactivated rabies vaccine purchased from Intervert International B.V. (Boxmeer, Netherlands) was used as a reference control. For simplicity, this vaccine is referred to as ITV in the manuscript. One dose of the ITV vaccine contains at least 2 international units (IU) of inactivated rabies virus Pasteur RIV strain. Lipid, PEG-lipid, and cationic compound (SW-01) were prepared by Stemirna Therapeutics (Shanghai, China). SW-01 is a cationic polymer that has been studied for mRNA delivery previously [Citation29]. ICR and C57BL/6 female mice (4-week-old) were purchased from Hunan SJA Laboratory Animal Co., Ltd (Changsha, China). Mice were housed in the Animal Facility at Huazhong Agricultural University. The 3-month-old beagles were purchased from and housed in Yizhicheng Biological Technology Co., Ltd (Yingcheng, China).
mRNA production
mRNA was produced using T7 RNA polymerase on linearized plasmids encoding codon-optimized G protein of a RABV vaccine strain Flury-LEP (GenBank: GU565703). The plasmid was linearized using the restriction endonuclease BspQI (NEB, Cat.no.R0712L). mRNA was transcribed to contain a 75 nucleotide-long poly(A) tail, with 100% of UTP substituted with 1-methylpseudo UTP to produce m1Ψ-modified mRNA. The m7G (5’) ppp (5’) G RNA Cap Structure Analog was used for co-transcriptional capping of mRNAs. The modified mRNAs included a 5’ Cap1, a 5’ untranslated region (5’ UTR) with part of the xenopus laevis Beta globin gene sequence, a coding region for G, a 3’ untranslated region (3’ UTR) with a segment from a partial sequence of the human α-globin, and a poly(A) tail. mRNA was purified by overnight LiCl precipitation at −20°C, centrifuged at 18,800 × g for 20 min at 4°C to pellet, washed with 70% EtOH, centrifuged at 18,800 × g for 1 min at 4°C, and resuspended in RNase-free water. Purified mRNA was analysed by agarose gel electrophoresis and stored at −80°C until further use.
mRNA transfection in vitro
For mRNA transfection into HEK-293 T or N2a cells, 3 × 105 cells per well were seeded in 12-well plates. Two micrograms of mRNA were transfected into HEK-293 T or N2a cells using jetPRIME kits (Polyplus Transfection, #0000000647) according to the manufacturer’s instructions. At 3–96 h (h) after transfection, cells were lysed and supernatant was collected for subsequent detection. G protein monoclonal antibody was used for indirect immunofluorescence analysis.
Preparation of LPP-mRNA-G vaccines
LPP nanoparticles were prepared using the two-step method described above [Citation29]. In brief, the cationic compound (SW-01) was dissolved in 25 mM sodium acetate (pH5.2) to prepare a SW-01 solution (0.5 mg/ml). The mRNA solution (0.1 µg/µl) and SW-01 solution were quickly stirred and mixed according to the volume ratio of 5:1, and then stood at room temperature for 30 min to form the mRNA/SW-01 complex. Then, lipids were dissolved in ethanol at molar ratios of 40:15:43.5:1.5 (ionizable lipid: 1, 2-dioleoyl-sn-glycero-3-phosphoethanolamine [DOPE]: cholesterol: PEG-lipid). The lipid solution was blended with the mRNA/SW-01 complexes using a microfluidic mixer (Inano D, Micro&Nano Technology Inc, China) at a proportion of 3 parts aqueous phase to 1 part ethanol phase. Finally, LPP-mRNA-G was sterilized with a 0.22 µm filter (Millipore) and stored at 4°C with an RNA concentration of about 0.1 mg/ml, and stored at 4°C for no more than 8 h for later use.
Nanoparticle characterization
Measurements of particle size (DLS) and potential were performed using a Zetasizer Nano ZS instrument (Malvern Instrument Co., Ltd.). Nanoparticles at appropriate concentrations were added to l cm test dishes and transferred to a dynamic light scattering instrument to measure particle size and surface electrical properties. Transmission electron microscopy (TEM) was performed after dilution of LPP-mRNA nanoparticles loaded with mRNA, with 10 µl aliquots placed onto copper mesh and left to stand for 10 min. Excess liquid was removed with filter paper and the morphology of LPP-mRNA nanoparticles was observed using a Talos L120C microscope (Thermo Fisher) under an infrared lamp.
Biodistribution of LPP-mRNA-G in the mouse body
The quadriceps muscle of the mouse hind leg was inoculated with 15 µg LPP-mRNA-G. Tissue samples from euthanized mice, including brain, heart, liver, spleen, lung, kidney, muscle, and LNs, were collected at 8, 24, 48, and 72 h after inoculation of LPP-mRNA-G. Before sampling, the mice were perfused to remove blood components and reduce the potential impact caused by nanoparticles in the blood. The mRNA and protein level of RABV-G in tissues was measured by signal enhancing b-DNA assays and ELISA, respectively. Quantification of RABV-G expression in vivo is based on a double-antibody sandwich ELISA method. Briefly, a polyclonal antibody specific for RABV-G was pre-coated onto plate wells. Standards and tissue homogenates were added to the wells and incubated for 2 h at room temperature. After three washes, plates were incubated with HRP-conjugated another anti-RABV-G monoclonal antibody for 2 h at room temperature, followed by three washes and incubation with TMB substrate. The absorbance at 450 nm was measured. A standard curve of absorbance at 450 nm versus concentration was fit with a linear equation for accurate RABV-G protein quantification. Quantification of mRNA level of RABV-G is based on a branched DNA (bDNA) principle that quantify RABV-G mRNA in samples. Briefly, the tissue homogenate was lysed by lysis the mixture with 100 µl of 1x RNA per well. Lysates were transferred to mRNA capture plates containing a specific capture probe, incubated overnight at 4°C, processed for bDNA amplification and analysed according to the manufacturer's instructions (Panomics QuantiGene2.0 Assay). The lymph nodes and muscle tissues were cryosectioned for immunofluorescence staining of RABV-G protein expression and then detected using RABV-G protein monoclonal antibodies. For in situ hybridization, probe DNA was synthesized based on the mRNA-G sequence. According to the principle of base complementarity to form a hybridized double-stranded nucleic acid that could be detected, probe DNA was labelled with a fluorescent dye, denatured into a single strand, and hybridized with treated muscle and lymph node tissue DNA. Results were observed and recorded under a fluorescence microscope. Images were captured using a LEICA Versa 200 microscope and processed using Caseviewer C. V 2.4 Image-Pro Plus 6.0 software.
Flow cytometry
T follicular helper cells (Tfh), germinal cell (GC) B cells, and plasma cells (PCs) isolated from draining lymph nodes (LNs) or bone marrows (BMs) were analysed by flow cytometry as previously described [Citation36]. Three groups of 4-week-old female C57BL/6 mice (n = 5) were immunized with a 100 µl of 5 µg LPP-mRNA-G vaccine, 0.1 dose ITV vaccine, or DMEM by intramuscular injection. LNs and BMs were collected 7 or 14 days after immunization. LNs and BMs were processed into single-cell suspensions and resuspended in phosphate-buffered saline (PBS) containing 0.2% (wt/vol) bovine serum albumin (BSA). Red blood cells were removed using lysis buffer (cat. no. 555899, BD Biosciences Inc., Franklin Lakes, NJ, USA). After washing twice, single cells were suspended in PBS containing 0.2% BSA (1 × 106 cells/sample) and incubated with fluorescence-conjugated antibodies in the dark for 30 min at 4°C. After incubation for 30 min at 4 °C, cells were washed twice with PBS containing 0.2% (wt/vol) BSA. Finally, stained cells were analysed using a BD FACSVerse system. Data were analysed using FlowJo software (TreeStar, CA, USA).
ELISpot assay
ELISpot assays were conducted to analyse RABV-specific ASCs. Multiscreen HA ELISpot plates (Millipore, MA, USA) were coated with 500 ng/well purified RABV-G expressed by Semliki Forest virus (SFV-G) as described previously and incubated at 4 °C overnight[Citation34]. Three groups of 4-week-old female C57BL/6 mice (n = 5) were immunized with 5 µg LPP-mRNA-G vaccine, 0.1 dose ITV vaccine, or DMEM by intramuscular injection. Drained LNs were collected 7 or 14 days after immunization and processed into single-cell suspensions. The next day, coated plates were washed three times with PBS-Tween (PBST; 0.5% tween-80, w/v) and blocked with RPMI 1640 supplemented with 10% FBS for 2 h at 37°C. Cell suspensions were transferred to blocked ELISpot plates and incubated for 24 h. The assay was conducted with biotin-conjugated mouse IgG antibody (Bethyl Laboratories, TX, USA), streptavidin-alkaline phosphatase (Mabtech, Stockholm, Sweden), and BCIP/NBT-plus (Mabtech, Stockholm, Sweden). Then, plates were scanned and analysed using a Mabtech IRIS FluoroSpot/ELISpot reader and RAWspot technology for multiplexing at the single-cell level.
ELISA
RABV-specific ELISA was performed to determine antibody isotypes as described previously [Citation34, Citation39]. Briefly, sera were collected and inactivated at 56°C for 30 min. ELISA plates were coated overnight at 4 °C with purified RABV virions diluted to a concentration of 500 ng per well in a protein coating buffer (5 mM Na2CO3, pH 9.6). After overnight incubation at 4°C, plates were washed three times with PBS-Tween (PBST; 0.5% tween-80, w/v) and blocked in 5% low-fat milk-PBS for 6 h at 4°C. Sera were then diluted in PBST containing 5% (wt/vol) low-fat milk at 1:2,000 for IgG, 1:100 for IgG1, and 1:200 for IgG2a and IgG2b. Afterward, 100 µl of diluted serum was added to the plates and incubated for 2 h at 37°C. Media was then discarded from the plates before washing three times in PBST. Then, 100 µl of horseradish peroxidase (HRP)-conjugated goat anti-mouse IgG, IgG1, IgG2a, and IgG2b was added to each well and incubated for 1 h at 37°C. After incubation, plates were washed three times with PBST and a chromogenic reaction with 100 µl of tetramethylbenzidine (TMB) substrate (Biotime Biotechnology, Shanghai, China) was allowed to continue for 25 min before the addition of 50 µl of 2 M H2SO4. Optical densities were recorded at 450 nm using a SpectraMax 190 spectrophotometer (Molecular Devices, CA, USA).
Virus-neutralizing antibody measurement
Virus-neutralizing antibody (VNA) titers were measured using fluorescent-antibody virus neutralization (FAVN) assays as previously described [Citation40]. Briefly, serum from mice was separated and inactivated for 30 min at 56°C. Then, 100 µl of DMEM was added to 96-well plates and 50 µl of test serum or standard serum was added into the first column and serially diluted three-fold. Each sample was added to four adjacent wells. A rabies challenge virus (CVS-11) suspension was added to each well. Plates were then incubated at 37°C for 1 h. Following incubation, 2 × 104 BSR cells were added to each well and the solutions were incubated at 37°C for 72 h. Samples were then fixed with 80% ice-cold acetone for 30 min and stained with FITC-conjugated antibodies against the RABV N protein. Fluorescence was observed under an Olympus IX51 fluorescence microscope (Olympus, Tokyo, Japan). Fluorescence values were compared with reference serum values (obtained from the National Institute for Biological Standards and Control, Hertfordshire, UK). Results were normalized and quantified in international units per ml (IU/ml).
Histopathology
At specified times during the experiment, mice were euthanized and organs were dissected. Tissues were fixed in 4% (v/v) paraformaldehyde solution for 72 h. Paraffin sections were prepared with a thickness of 3 − 4 µm. Hematoxylin and eosin staining was used to identify histopathological changes in tissues. Histopathological analyses of organs and tissues were performed under a light microscope. For immunofluorescence assay, RABV-P expression was detected by using anti-P protein monoclonal antibodies.
Mouse immunization and challenge
ICR mice (4-week-old) were randomly allocated to the indicated groups. Mice received a single-dose immunization of 5, 1, or 0.2 µg of LPP-mRNA-G. As a positive control, mice were administered with 0.1 dose ITV vaccine, a commercial rabies vaccine. For mouse vaccination, ICR mice received intramuscular inoculation with LPP-mRNA-G, ITV vaccine, or placebo (sterile PBS only) using a 1 ml sterile syringe. Blood was collected from the orbital sinus under anesthesia, followed by centrifugation and serum collection. A mouse model of rabies using the virulent RABV strain CVS-24 has previously been characterized [Citation36]. An intracranial challenge with 30 µl of 50 LD50 CVS-24 was performed 3 weeks post immunization (wpi). Body weight and survival of mice were monitored for 21 days after the challenge.
Vaccination and serum collection in dogs
For dog vaccination, 3-month-old beagles were randomized into five groups, with 5 dogs per group. One group was inoculated with one dose of ITV vaccine and the other four groups were administered either 50, 10, 2, or 0.4 µg LPP-mRNA-G into the quadriceps muscle (n = 5/group). The 50 μg LPP-mRNA-G group was intramuscularly inoculated with the same dose at 3 weeks post the primary immunization for safety evaluation. Blood was collected from the jugular vein at weeks 0, 1, 2, 3, 4, 5, and 6 for measuring antibody titers. Daily clinical sign observations, including measurements of anal temperature and body weight, were performed throughout the trial.
Statistical analyses
Data analyses were performed using GraphPad Prism software 8.0 (GraphPad Software, Inc., CA). Survival statistical analysis was performed using a log-rank (Mantel–Cox) test. For other data, statistical differences between groups were analysed by using one-way ANOVA followed with post-hoc tests. The following notations were used to indicate significant differences between groups: *, P < 0.05; **, P < 0.01; ***, P < 0.001; ****, P < 0.0001; ns, no significant difference.
Ethics statement
All mouse studies were conducted at the Laboratory Animal Center of Huazhong Agricultural University. During the RABV challenge study, the mice with body weight drop more than 25%, severe paralysis, or inability to feed were euthanized by CO2. The experimental protocol was reviewed and approved by the Scientific Ethics Committee of Huazhong Agricultural University with the approval number, HZAUMO-2021-0116. The immunization of dogs was conducted at the General Animal Room Facility of Yi-zhi-cheng Biological Technology Co., Ltd (Hubei, China). This study was reviewed and approved by the Scientific Ethics Committee of Huazhong Agricultural University with the approval number, HZAUDO-2022-0001.
Results
Construction and characterization of a rabies mRNA vaccine based on LPP in vitro and in vivo
Having previously demonstrated the concept of mRNA vaccination in humans using rabies glycoprotein as an antigen [Citation41], we designed an mRNA vaccine encoding glycoprotein of the RABV vaccine strain Flury-LEP (mRNA-G), with 100% UTP substituted with 1-methylpseudo UTP to reduce innate immunogenicity [Citation42, Citation43] (A). To mediate efficient and prolonged antigen expression by mRNA in vivo, mRNA-G was further prepared for vaccination by encapsulation in LPPs, resulting in LPP-mRNA-G. The system consisted of a core structure of a polymer/mRNA multi-complex encapsulated in a bilayer phospholipid molecular layer (B). Transmission electron microscope (TEM) analysis showed that LPP-mRNA-G exhibited an electron-dense core (C). The dynamic light scattering of LPPs in PBS indicated that the average particle size was 122.4 nm (D), with a narrow polydispersity index (PDI) of 0.104. Zeta potential measurements showed that the zeta potential increased from −7.78 mV at pH 7.4 to +14.95 mV at pH 4.0 (E). These results suggest that LPPs respond to pH changes.
Figure 1. Construction and characterization of the LPP-mRNA-G vaccine in vitro and in vivo. (A) Schematic of nucleoside-modified RABV mRNA. Each structural element is optimized and modified to modulate mRNA stability, translation ability, and immunostimulatory characteristics. (B) Schematic of the LPP-mRNA-G vaccine construct. (C) Representative transmission electron microscopy image of LPPs in solution following mRNA encapsulation. Scale bar, 50 nm. (D) LPP particle size by dynamic light scattering. (E) Zeta potential of LPPs at pH 4.0 and 7.4. For c and e, one representative result from three independent experiments is shown.
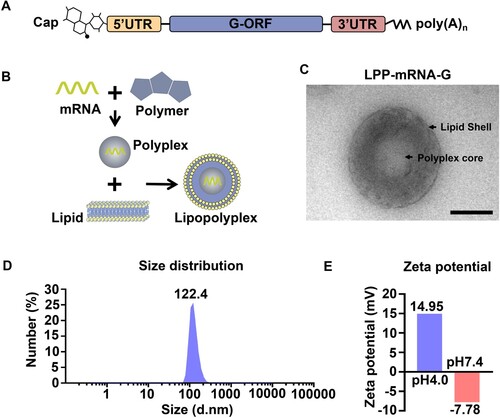
Cellular expression profile of LPP-mRNA-G and biodistribution in the mouse body
The transfection ability of LPP-mRNA-G was evaluated by direct incubation with 293 T cells. The results showed that G protein was expressed in 293 T cells (A). LPP-mRNA-G led to significantly higher gene expression compared to the same dose of naked mRNA and the commercial transfection reagent jetPRIME. Gene expression could be detected just 3 h after the addition of LPP-mRNA-G, with peak expression observed at around 72 h (B).
Figure 2. Transfection efficiency of LPP-mRNA-G in cells and biodistribution in the mouse body. (A) HEK-293 T cells were transfected with LPP-mRNA-G. G protein levels in cell lysates at 24 h were measured by western blotting. (B) Transfection efficiency of LPP-mRNA-G. The mRNA-G was transfected into HEK-293 T cells using LPP or the commercial transfection reagent, jetPRIME. The expression of G protein was observed under fluorescence microscopy after incubation for 3, 12, 72, or 96 h. Scale bar, 200 µm. (C) Duration and distribution of LPP-mRNA-G in vivo. Groups of ICR mice (n = 3) were vaccinated with one injection of 15 µg LPP-mRNA-G. Three mice per group were euthanized at 8, 24, 48, and 72 h after inoculation. Draining lymph node, muscle, brain, heart, liver, kidney, spleen, and lung samples were harvested from each mouse for quantitation of mRNA-G levels using b-DNA assays. The pie chart represented the of G-mRNA accumulative distribution of the sum at each timepoint (8, 24, 48, and 72 h) post immunization (top right corner panel). Data are presented as the mean. (D) Duration and distribution of G protein production from LPP-mRNA-G in vivo. Groups of ICR mice (n = 3) were vaccinated with one injection of 10 µg LPP-mRNA-G. Three mice per group were euthanized at 8, 24, 48, and 72 h after inoculation. Draining lymph node, muscle, brain, heart, liver, kidney, spleen, and lung samples of each mouse were harvested for quantitation of G protein expression levels by ELISA. The pie chart represented the of G protein accumulative distribution of the sum at each timepoint (8, 24, 48, and 72 h) post immunization (top right corner panel). Data are presented as the mean. (E) Expression levels of G protein were further detected by immunofluorescence assays using monoclonal antibodies against G protein in lymph nodes and muscle tissues. mRNA concentrations were determined by in situ hybridization using probes specific for the mRNA component of LPP-mRNA. Scale bars, 200 or 50 µm.
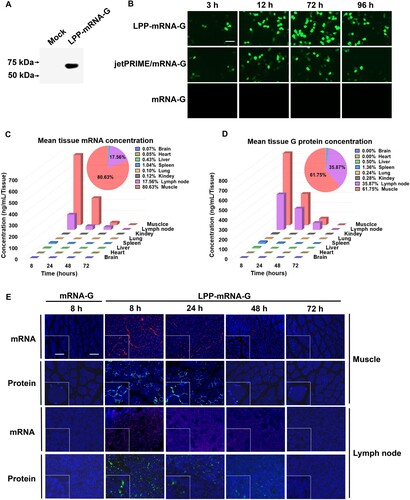
The biodistribution of mRNA vaccines in tissues after administration may influence safety and efficacy. To examine the duration and distribution of LPP-mRNA-G in vivo, ICR mice were immunized with 15 µg LPP-mRNA-G via the intramuscular route, with naked mRNA-G used as a control. Mice were euthanized at 8, 24, 48, and 72 h post immunization. Muscle, draining lymph node, brain, heart, liver, kidney, spleen, and lung samples were obtained for measuring RABV-G mRNA levels by signal enhancing b-DNA assays and protein levels by ELISA, respectively. The results demonstrated that most of the LPP-mRNA-G nanoparticles were distributed in the muscles of the injection site (80.63%), with a smaller proportion observed in the draining LNs (17.56%). The remaining nanoparticles were distributed in multiple tissues including the brain (0.07%), heart (0.05%), liver (0.43%), spleen (1.04%), lung (0.10%), and kidney (0.12%). mRNA levels peaked at 8 h post-inoculation and rapidly decreased from 8 to 72 h before becoming undetectable at 72 h (C). In addition, the distribution and duration of RABV-G protein level exhibited a similar pattern to that of mRNA level (D). In situ hybridization and fluorescent immunohistochemistry revealed that mRNA and protein level of RABV-G in muscles and lymph nodes was not detectable after 72 h (E). Overall, these data indicate that the LPP-mRNA-G platform has a favourable biodistribution profile devoid of liver accumulation and has utility in providing efficient and sustained expression of mRNA molecules.
LPP-mRNA-G induces potent antibody production in mice
The antibody responses induced by the LPP-mRNA-G vaccine candidate were analysed in mice. In order to better simulate clinical practice, we used the outbred ICR mice to evaluate the antibody production induced by vaccines. ICR mice were intramuscularly immunized with 5, 1, or 0.2 µg LPP-mRNA-G at day 0. As a positive control, mice were administered with 0.1 dose of ITV vaccine since previous studies had shown that this dose produced a protective effect in mice [Citation36]. Blood samples were collected for 8 consecutive weeks for virus-specific VNA, IgG, and antibody subtype analyses (A). After single-needle vaccination, all doses of LPP-mRNA-G-immunized mice produced a VNA titer more than 0.5 IU/ml. VNA titers of the 5 µg LPP-mRNA-G group were higher than those in ITV vaccine-immunized mice. VNA titers in the ITV vaccine group were significantly decreased at 4 wpi, while VNA titers of the 5 µg LPP-mRNA-G group only began to decrease significantly after 5 wpi, but still remained above the sero-conversion level (VNA ≥ 0.5 IU/ml) after 8 wpi (C).
Figure 3. A single dose of LPP-mRNA-G vaccination induces high levels of antibody production in mice. (A) Vaccine immunization strategy. Groups of mice were immunized by intramuscular injection of 5, 1, or 0.2 µg of LPP-mRNA-G. A licensed commercial inactivated rabies vaccine (ITV) was included as a positive control and the same volume of PBS was injected as a placebo. Serum was collected for IgG and VNA testing for 8 consecutive weeks. The total IgG and VNA produced in PBS-inoculated mice were undetectable. (B) Detection of RABV IgG antibody by ELISA. One-way ANOVA was used to evaluate intergroup differences. ns, no significant difference; *, P < 0.05; **, P < 0.01. Error bars indicate means with SEM (n = 10). (C) VNA titers were measured using fluorescent-antibody virus neutralization (FAVN) assays. One-way ANOVA was used to evaluate intergroup differences. ns, no significant difference; *, P < 0.05; **, P < 0.01. Error bars indicate means with SEM (n = 10).
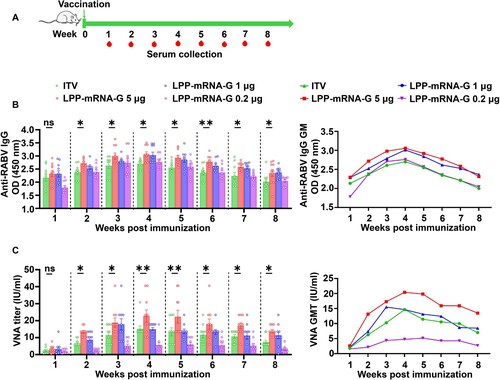
To further examine antibody response, we performed enzyme-linked immunosorbent assays to measure serum levels of IgG, IgG1, IgG2a, and IgG2b at 8 weeks after a single immunization with each vaccine. The titers of total IgG (B), IgG1(Figures S1A), IgG2a (Figures S1B), and IgG2b (Figures S1C) induced by 5 µg LPP-mRNA-G were significantly higher than those of ITV vaccine. The IgG2a/IgG1 ratio (Figures S1D) and IgG2b/IgG1 ratio (Figures S1E) showed LPP-mRNA-G induced a Th1-skewed humoral response. We studied the dose-dependence of the LPP-mRNA-G vaccine and monitored VNA titers over 8 weeks. VNA levels increased rapidly and remained at a consistently high level (5 − 20 IU/ml) in the 5 and 1 µg LPP-mRNA-G groups, and at consistently low levels (≥ 0.5 IU/ml) in the 0.2 µg group. Taken together, these results suggest that LPP-mRNA-G induces potent humoral immune response in mice.
LPP-mRNA-G facilitates the generation of Tfh cells, GC B cells, PCs, and RABV-specific ASCs in mice
T follicular helper cells (Tfh), a specialized subset of CD4 + T cells, provide instructive signals that lead to the survival, affinity maturation, and fate decision of germinal cell (GC) B cells [Citation44, Citation45]. Thus, the role of LPP-mRNA-G in Tfh and GC B cell induction was further investigated. C57BL/6 mice (n = 5) were immunized with 5 µg of LPP-mRNA-G, 0.1 dose of ITV vaccine (as a control), or DMEM. Flow cytometry analysis was then performed to quantify Tfh cells (CD4+ PD1+ CXCR5+; A, Figure S2A) and GC B cells (B220+ GL7+ CD95+; C, Figure S2B) in draining lymph nodes (LNs) at days 7 and 14 post immunization (dpi). Significantly greater numbers of Tfh (B) and GC B cells (D) were observed in draining LNs from mice immunized with LPP-mRNA-G compared to draining LNs from mice immunized with ITV vaccine at 7 and 14 dpi.
Figure 4. LPP-mRNA-G facilitates the generation of Tfh cells, GC B cells, PCs, and RABV-specific ASCs in mice. (A) Representative flow cytometric plots of Tfh cells. C57BL/6 mice were immunized with 5 µg of LPP-mRNA-G, 0.1 dose of ITV vaccine, or DMEM. At 7 and 14 dpi, inguinal LN cells were stained with markers of Tfh cells (CD4+ CXCR5+ PD1+). (B) Statistical analyses of CD4+ CXCR5+ PD1+ Tfh cell plots. One-way ANOVA was used to evaluate intergroup differences. *, P < 0.05; ***, P < 0.001. Error bars indicate means with SD (n = 5). (C) Representative flow cytometric plots of GC B cells. At 7 and 14 dpi, inguinal LN cells were stained with markers of GC B cells (B220+ GL7+ CD95+). (D) tatistical analyses of B220+ GL7+ CD95+ GC B cell plots. One-way ANOVA was used to evaluate intergroup differences. *, P < 0.05; ***, P < 0.01. Error bars indicate means with SD (n = 5). (E) Representative flow cytometric plots of PCs. At 7 and 14 dpi, BM cells were stained with markers of PCs (B220low CD138+). (F) Statistical analyses of B220low CD138+ PC plots. One-way ANOVA was used to evaluate intergroup differences. *, P < 0.05; ***, P < 0.0001. Error bars indicate means with SD (n = 5). (G) Representative images of ELISpot assays. At 7 and 14 dpi, inguinal LN cells were prepared and RABV-specific ASCs were counted by ELISpot assay. (H) Statistical analyses of the number of RABV-specific ASCs. One-way ANOVA was used to evaluate intergroup differences. *, P < 0.05; ***, P < 0.0001. Error bars indicate means with SD (n = 5).
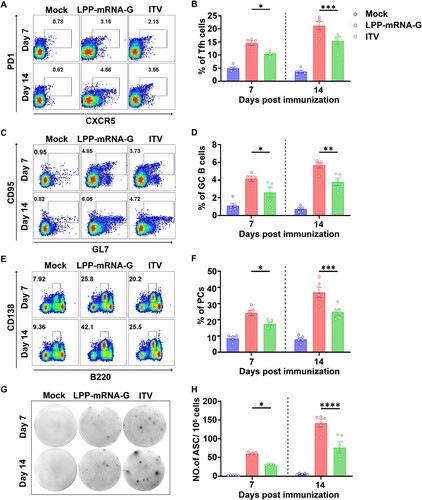
In the GC reaction, B cells gain an affinity for the cognate antigen and expand preferentially. In this process, GC B cells can differentiate into either memory B cells (MBCs) or plasma cells (PCs) secreting large quantities of antibodies in response to antigens [Citation46, Citation47]. To examine the effects of LPP-mRNA-G on PC formation, the percentage of PCs (B220low CD138+; E; Figure S2C) was assessed by flow cytometry after inoculation with LPP-mRNA-G, ITV vaccine, or DMEM (n = 5). Mice immunized with LPP-mRNA-G generated significantly more PCs in bone marrows than those immunized with ITV vaccine (F). Further, the generation of RABV-specific antibody-secreting cells (ASCs) in LNs was evaluated by ELISpot assay at 7 and 14 dpi (G). As expected, mice immunized with LPP-mRNA-G had significantly more RABV-specific ASCs than mice immunized with ITV vaccine (H). Taken together, these data suggest that LPP-mRNA-G promotes the generation of Tfh cells, GC B cells, PCs, and RABV-specific ASCs compared to the ITV vaccine in mice.
LPP-mRNA-G induces strong secondary antibody response in mice
MBCs generate immunoglobulins rapidly and vigorously upon secondary infections [Citation48]. To assess whether LPP-mRNA-G induces memory immunity after immunization, we examined the abundance of MBCs by analysing B220 + CD38 + CD138− cells with flow cytometry after primary immunization (A, Figure S2D). Significantly more MBCs were observed in mice immunized with LPP-mRNA-G compared to mice immunized with ITV vaccine at 100 dpi (B). In order to further evaluate the impact of LPP-mRNA-G and ITV vaccine on the secondary antibody response, at 100 dpi, mice (n = 5) were subjected to booster immunization using the same doses as the primary immunization (5 µg LPP-mRNA-G, 0.1 dose of ITV vaccine, or DMEM). Two weeks after secondary immunization, PCs (B220low CD138+) in BMs (C) and RABV-specific ASCs in LNs (E) were evaluated by flow cytometry and ELISpot assays, respectively. LPP-mRNA-G induced significantly more abundant PCs (D) and RABV-specific ASCs than ITV vaccine (F). Correspondingly, LPP-mRNA-G induced higher RABV-specific IgG levels (G) and VNA titers (H) compared to the ITV vaccine in mice at 14 days after receiving a boosting dose. Together, these results suggest that LPP-mRNA-G induces increased numbers MBCs and stronger secondary immune responses compared to the ITV vaccine.
Figure 5. LPP-mRNA-G induces strong secondary antibody response in mice. (A) Representative flow cytometric plots of MBCs. C57BL/6 mice were immunized with 5 µg of LPP-mRNA-G, 0.1 dose of ITV vaccine, or DMEM. At 100 dpi, inguinal LN cells were stained with markers of MBCs (B220 + CD38 + CD138−). (B) Statistical analyses of B220 + CD38 + CD138− MBC plots. One-way ANOVA was used to evaluate intergroup differences **, P < 0.01. Error bars indicate means with SD (n = 5). (C) Representative flow cytometric plots of PCs. Mice (n = 5) were boosted 5 µg of LPP-mRNA-G, 0.1 dose of ITV vaccine, or DMEM at 100 dpi after primary immunization. At 14 days after the second dose, single BM cells were stained with markers of PCs (B220low CD138+). (D) Statistical analyses of B220low CD138+ PC plots. One-way ANOVA was used to evaluate intergroup differences. **, P < 0.01. Error bars indicate SD of the mean (n = 5). (E) Representative images of ELISpot assays. At 14 days after the second dose, inguinal LN cells were prepared and RABV-specific ASCs were counted by ELISpot assay. (F) Statistical analyses of the number of RABV-specific ASCs. One-way ANOVA was used to evaluate intergroup differences. ****, P < 0.0001. Error bars indicate means with SD (n = 5). (G) Detection of RABV IgG antibody by ELISA before and after the second dose (14 dpi). One-way ANOVA was used to evaluate intergroup differences. ns, no significant difference; ***, P < 0.001. Error bars indicate means with SEM (n = 5). (H) VNA titers were measured by fluorescent-antibody virus neutralization (FAVN) assays before and after the second dose (14 dpi). One-way ANOVA was used to evaluate intergroup differences. ns, no significant difference; ***, P < 0.001. Error bars indicate means with SEM (n = 5).
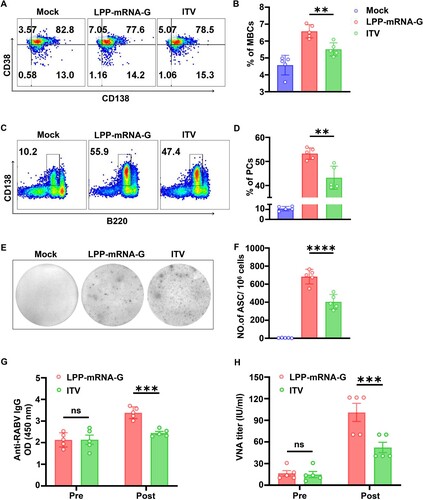
A single low-dose immunization with LPP-mRNA-G provides a full protection for mice against lethal RABV challenge
To further explore the protective effect of LPP-mRNA-G against RABV infection in vivo, ICR mice were immunized with a single dose of 5, 1, or 0.2 µg LPP-mRNA-G or 0.1 dose of ITV rabies vaccine via the muscular route and challenged with a lethal dose of 50 LD50 virulent RABV strain, CVS-24, after 3 wpi (A). We observed 100% survival in mice administered with 1 or 5 µg LPP-mRNA-G vaccine, 70% survival in mice administered with 0.2 µg LPP-mRNA-G vaccine, and 50% survival in mice administered with ITV vaccine. In addition, all placebo-inoculated mice succumbed to rabies within 10 days (B). In all vaccine-immunized groups, a steady increase in body weight following a brief decline was observed; in contrast, a sustained decrease in body weight was observed in the negative control group (C). These results indicated that 1 µg LPP-mRNA-G vaccine provided effective protection against lethal RABV challenge. A separate group of mice inoculated with 1 µg LPP-mRNA-G were euthanized 7 days after immunization, and tissues were harvested for measurements of viral load and histopathological analyses. Immunofluorescence assays with a monoclonal antibody against RABV-P demonstrated that inoculation with LPP-mRNA-G and ITV vaccine effectively inhibited virus replication in mouse brains; however, sporadic viral particles was detected in the ITV vaccine group (Figure S3A). Histopathological examination demonstrated that immunization of mice with LPP-mRNA-G conferred protection against RABV challenge as no lesions were observed in the brain. A decrease in the number of Purkinje cells, a slight decrease in dendritic complexity, and a decrease in cell surface area were observed in ITV vaccine-treated mice (Figures S3B and S3C).
Figure 6. A single immunization of LPP-mRNA-G provides full protection against lethal RABV challenge in mice. (A) Scheme of LPP-mRNA-G immunization and RABV challenge. Each mouse received intramuscular injections of 5, 1, or 0.2 µg LPP-mRNA-G followed by intracranial injection of 50 LD50 of the RABV strain, CVS-24. Mice receiving a 0.1 dose of ITV vaccine, were used as positive controls. (B) Survival rates. A log-rank test was used to evaluate intergroup differences in survival rates. *, P < 0.05; **, P < 0.01. (C) Changes in body weight. Error bars indicate means with SD (n = 10).
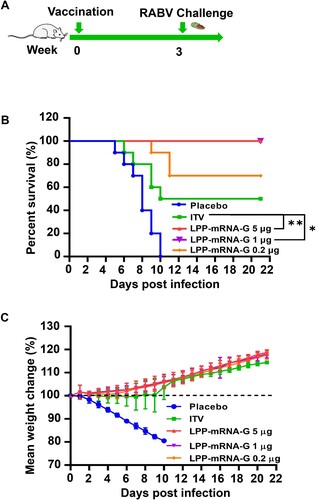
It has been reported that viral infection and related neuroinflammation early in life are involved in the pathogenesis of various emotional, cognitive, and behavioural disorders [Citation49]. We aimed to determine whether transient RABV infection post vaccination causes the development of neurological diseases and their associated sequelae. We selected mice challenged with RABV for 7 days or 6 months as behavioural subjects. The T-maze test, climbing pole test, and balance beam test are effective methods for assessing spatial memory, learning, and motor balance, respectively, in mice. We observed no differences in behaviour between LPP-mRNA-G vaccinated mice and healthy, normal mice on day 7 of RABV infection, with significant differences observed between ITV vaccine-vaccinated mice and other groups (Figures S3D–S3F, left panel). Notably, testing of surviving mice demonstrated persistent deficits in spatial memory, learning, and motor ability on T-maze and pole-climbing testing in ITV vaccine-treated mice compared with healthy mice (Figures S3D and S3E, right panel). In the balance beam test, no significant differences were observed in ITV vaccine-treated and LPP-mRNA-G-treated mice compared with healthy mice (Figure S3F, right panel). These findings indicate that LPP-mRNA-G promotes the elimination of RABV during the early stages of virus infection and reduces the incidence of neurological sequelae related to RABV infection.
A single low-dose vaccination with LPP-mRNA-G elicits potent antibody production in dogs
To further investigate the immunogenicity of LPP-mRNA-G in dogs, we first evaluate the safety of LPP-mRNA-G by intramuscularly inoculating a high-dose of LPP-mRNA-G in beagles (50 µg /dog, n = 5). At 3 weeks post the primary immunization, the beagles were received the same dose by intramuscular immunization. The body weights (Figure S4A), temperatures (Figure S4B) and serum biochemical indexes (Table S1-2) both showed that even immunization with high-dose of LPP-mRNA-G caused no obvious side effects in dogs. Then groups of beagles (n = 5) were inoculated intramuscularly with 10, 2, or 0.4 µg LPP-mRNA-G or one dose ITV vaccine, respectively (A). LPP-mRNA-G induced higher titers of RABV-specific VNA and G-specific IgG than ITV vaccine (B). To investigate the duration of vaccine-induced humoral immunity, we monitored VNA titers over a 6-week period. The results demonstrated higher VNA titers in dogs that received 10 µg or 2 µg LPP-mRNA-G compared to dogs in the ITV vaccine group. VNA titers were maintained above 0.5 IU/ml despite decreasing after 6 weeks of immunization. VNA titers in the 0.4 µg LPP-mRNA-G group were lower than in dogs that received ITV vaccine but remained above 0.5 IU/ml, which is threshold of sero-conversion level defined by WHO (C).
Figure 7. A single dose of LPP-mRNA-G elicits potent antibody production in dogs. (A) Scheme of LPP-mRNA-G immunization and evaluation. Beagles received three different doses (10, 2, or 0.4 µg) of LPP-mRNA-G by intramuscular injection. After inoculation, injection sites were continuously observed for signs of adverse reactions. Body temperature and body weight were measured daily. Animals in the simulation group received injections of PBS. Beagles receiving one dose ITV vaccine, were used as positive controls. Sera were collected at the indicated time points. (B) Detection of RABV IgG antibody by ELISA. Error bars indicate SEM of the means (n = 5). (C) VNA titers were measured using fluorescent-antibody virus neutralization (FAVN) assays. Error bars indicate means with SEM (n = 5). (D) The proportion of dogs with seroconversion induced by vaccination. VNA values greater than 0.5 IU/ml were considered positive.
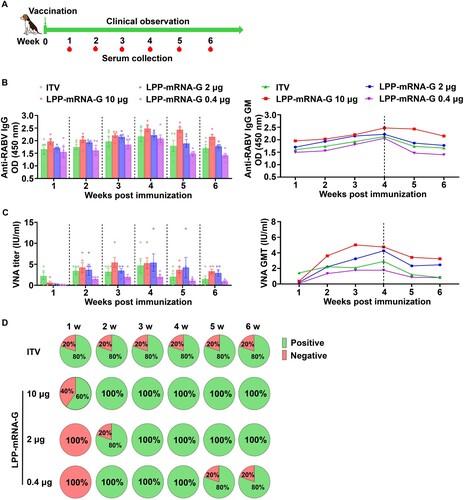
Notably, the seroconversion rate remained at 80% in ITV vaccine groups 1–6 wpi, with seroconversion defined as antibody titers ≥ 0.5 IU/ml [Citation32]. In the 10 µg LPP-mRNA-G group, 60% of dogs had detectable VNA titers at 1 wpi. In the 2 and 0.4 µg LPP-mRNA-G groups, no dogs had detectable VNA titers in the 1 wpi, but all dogs seroconverted at 3 wpi. In the 0.4 µg group, all dogs seroconverted at 2 wpi, but 20% of dogs were negative for VNA at 5 wpi (D). These results suggest that 2 µg LPP-mRNA-G can induce a strong humoral immune response in dogs. Additionally, the RABV-specific IgG levels were analysed in all dogs and demonstrated a similar pattern to VNA titers (B). Taken together, these results demonstrate a single, low dose of LPP-mRNA-G can induce potent antibody production in dogs.
Lastly, the stability of mRNA vaccines plays a crucial role in their storage and transportation. To assess vaccine stability, LPP-mRNA-G formulations were stored at different temperatures for various durations (0, 1, 4, 7, and 14 days) before being administered via intramuscular injection to mice, and neutralizing antibody levels were measured four weeks later. The results indicated that LPP-mRNA-G formulations showed a decline in immunogenicity only after 14 dpi under 4°C storage conditions (Figure S5A). At 25°C, neutralizing antibody levels remained at 5.3 IU/ml even on the 14 dpi (Figure S5B), while at 37°C, they were at 4.0 IU/ml on the 7 dpi (Figure S5C). These findings suggest that LPP-mRNA-G vaccine exhibits some degree of thermal stability.
Discussion
By utilizing affordable vaccines, the World Health Organization aims to achieve the goal of zero rabies deaths by 2030 [Citation50]. Although currently licensed inactivated virus-based rabies vaccines have demonstrated efficacy, their cost and availability have hampered vaccination in developing countries [Citation8, Citation11]. In this study, we developed a nucleoside-modified G-encoded mRNA vaccine against RABV and demonstrated that a single, low dose of LPP-mRNA-G induced robust humoral immunity and provided complete protection against lethal RABV challenge in mice. The ideal biodistribution pattern of LPP-mRNA-G observed in vivo may reduce the risks of vaccination. It is notable that, in a study on the evaluation of rabies mRNA vaccine in dogs, we found that a single, low dose of LPP-mRNA-G induced robust VNA responses with a high rate of sero-conversion in dogs.
Technical developments in the field of mRNA modification and delivery systems have facilitated the application of mRNA vaccines for the prevention and treatment of infectious diseases [Citation25, Citation51]. mRNA vaccines are potentially quicker and more cost-efficient to produce, and more agile and flexible allowing rapid development of novel vaccines against emerging infectious diseases in large quantities [Citation52, Citation53]. So far, mRNA vaccines have been shown to be safe and immunogenic in human and animals [Citation32, Citation41, Citation54, Citation55]. In a clinical study conducted by CureVac, mRNA vaccines encoding RABV-G (CV7201 and CV7202) induced high antibody titers and strong CD8+ and CD4+ T-cell response [Citation41, Citation54]. According to a study by Lutz et al. in 2017, unmodified mRNA formulated with optimized LNPs demonstrated outstanding immunogenicity and long-term efficacy in non-human primates (NHPs) [Citation56]. These vaccines not only compete with approved vaccines but also exhibit superior performance in terms of functional antibody and T-cell responses, providing strong evidence for the feasibility of mRNA technology as an effective prophylactic vaccine development platform against infectious diseases. The currently under development mRNA vaccines for rabies have been demonstrated to possess outstanding immunogenicity, including the induction of effective antibody and cellular immune responses [Citation56]. Herein, we develop a novel rabies mRNA vaccine based on LPP, which exhibits excellent immunogenicity in mice and dogs even with a single low dose.
While a variety of delivery systems for mRNA vaccines have been developed, concerns regarding the unfavorable biodistribution pattern remain due to the unique size and composition of mRNA vaccine delivery systems [Citation29]. Adverse biodistribution not only reduces mRNA utilization efficiency but also potentially reduces the safety of vaccines [Citation57, Citation58]. Intramuscular administration of LPP-mRNA-G demonstrated a favourable biodistribution pattern with limited accumulation in the liver or other major organs, indicating a benign and desirable toxicity profile [Citation29]. It is worth noting that our results show that some nanoparticles reach the brain through the blood–brain barrier, although this content is extremely low (0.07%). Fortunately, we did not detect the corresponding antigen expression in the brain, and because of the high immunogenicity of LPP-mRNA-G vaccine, we can inoculate a lower dose, which may potentially increase the safety of the vaccine. No matter LPP or LNP, the previous studies on biological distribution and safety also focused on liver, spleen, lung, kidney, lymph nodes and muscles, and there was a lack of sufficient safety data for nanoparticle vaccines to enter the brain [Citation28, Citation59, Citation60]. All these remind us that we need to pay attention to the potential safety risks that nanoparticle vaccines may bring into the brain. Furthermore, the biodistribution of LPP-mRNA-G observed in the present study differed from the pattern of transgene expression by encapsulated mRNAs due to variations in mRNA release efficiency between tissues. The distribution of LPP-mRNA-G in muscles was more than 4 times that in LNs following intramuscular administration; however, the mRNA expression efficiency was only 1.7 times greater in muscles compared to LNs. This finding indicates that a non-linear relationship exists between LPP-mRNA-G exposure and protein expression in different tissues.
A single, low dose of LPP-mRNA-G (1 µg) induced high VNA titers in mice, which conferred complete protection against lethal RABV challenge. Our results in a mouse model demonstrate that LPP-mRNA-G generates greater numbers of Tfh cells, GC B cells, PCs, and RABV-specific ASCs than ITV vaccine, thereby increasing the immunogenicity of LPP-mRNA-G. At the same time, 0.2 µg LPP-mRNA-G produced a lower VNA titer but provided a higher protection level compared to the ITV vaccine. This phenomenon may be due to the involvement of CD4+ T cells induced by LPP-mRNA-G, in addition to the production of VNA. Indeed, mRNA vaccines have previously been shown to elicit increased numbers of CD4+ T cells [Citation32]. Although the role of CD4+ T cells in protection against RABV has yet to be fully elucidated, sufficient CD4+ T helper cell responses are required for optimal humoral immunity induction and virus clearance in the central nervous system [Citation32, Citation54, Citation61, Citation62].
Although the mRNA vaccines are able to induce potent antibody response, further improvements are imperative for the development of a viable mRNA vaccines. In view of the successful induction of strong humoral immune responses and high VNA titers in mouse models with low doses of LPP-mRNA-G, we continued the use of a low, single dose immunization strategy in dogs. Using this strategy, VNA responses were induced that were well above the WHO threshold of 0.5 IU/ml. In dogs, VNA titers almost all reached the threshold of 0.5 IU/ml at 2 wpi, and remained stable in the 10 and 2 µg LPP-mRNA-G groups stable throughout the experiment. Meanwhile, only 80% of dogs respond to a single dose of ITV, and a qualified rabies vaccine should provide 100% response. In canine experiments, individual differences may occur, leading to some dogs not exhibiting an immune response after receiving the control vaccine. These individual differences may involve multiple factors, including immune system diversity, genetic factors, and the overall health condition of the dogs. Especially in cases with a limited sample size, these individual differences can be amplified. LPP-mRNA-G vaccine can achieve 100% immune response in the case of single-needle immunization. Administering only one dose of ITV vaccine may lead to the loss of immune response, which reminds us of the importance of improving the immune response rate while developing a single-needle rabies vaccine. As shown with mRNA vaccines against SARS-Cov-2, vaccine-induced decreases in antibody titers may increase the risk of breakthrough infections [Citation63, Citation64]. These findings underscore the importance of assessing antibody durability in vaccine research. Therefore, this study needs to continue monitoring the long-term antibody dynamics in dogs vaccinated with LPP-mRNA-G to provide valuable information regarding the persistence of immune protection.
Current rabies vaccines, whether inactivated or mRNA vaccines, have proven effective in controlling the disease. The main obstacles to broader vaccine coverage, especially for vaccinating stray dogs in developing countries, are cost and accessibility. While mRNA vaccines have demonstrated effectiveness in generating immunity and preventing rabies infection, their increased cost and storage requirements may exacerbate these existing challenges. It's worth noting that with the strong demand for mRNA technology, ongoing developments in the manufacturing processes of mRNA vaccine raw materials will lead to further cost reductions. Meanwhile, significant progress has been made in the application research of circular mRNA (circRNA). Unlike mRNA, circRNA does not require enzymatic modifications, which further reduces production costs [Citation65]. Furthermore, the application of freeze-drying technology to mRNA vaccines has made long-term storage of mRNA vaccines possible [Citation66, Citation67]. In our research, the LPP-mRNA-G vaccine showed some degree of thermal stability, which may be attributed to the unique complex core of LPPs that contributes to enhancing mRNA stability. The introduction of LPP-mRNA-G vaccines will provide more options for addressing issues like cost and accessibility.
In summary, the results of the present study demonstrate that a single, low dose of LPP-mRNA-G provides complete protection against RABV infection in a mouse model. LPP-mRNA-G may have utility as a safe and effective platform for vaccine development against rabies, as well as other emerging and re-emerging infectious diseases, due to its excellent stability, delivery efficiency, and favourable biodistribution pattern.
Supplemental Material
Download JPEG Image (6.7 MB)Supplemental Material
Download JPEG Image (7 MB)Supplemental Material
Download JPEG Image (9.9 MB)Supplemental Material
Download JPEG Image (1.8 MB)Supplemental Material
Download JPEG Image (1.6 MB)Supplemental Material
Download MS Word (139.9 KB)Acknowledgments
We thank Yihua Cai, Chunxiu Chen, Mingcheng Xu, Lei Huang, Songhe Kan, and Haili Wang at Stemirna Therapeutics. Author contributions: Jiawu Wan and Zongmei Wang planned and performed experiments, analysed data, and wrote the manuscript; Chengguang Zhang performed experiments and analysed data; Jianmei Yang, Yinglei Yi, Haiwei Sun, Yuntao Wu and Ruizhong Shen contributed samples and discussed data; Ming Zhou, Huanchun Chen, Zhen F. Fu, Haifa Shen and Hangwen Li planned experiments, analysed, and discussed data; Ling Zhao directed the study and wrote the manuscript. All authors have reviewed the paper.
Disclosure statement
Stemirna Therapeutics filed patents on patent application entitled “A rabies mRNA vaccine for animals”. All other authors declare no conflict of interest.
Data availability
All data associated with this study are present in the paper or the Supplementary Materials. Request for resources, data and reagents should be directed to the lead contact, Ling Zhao ([email protected]). All unique reagents described in this study are available upon request to the lead author with a completed Materials Transfer Agreement.
Additional information
Funding
References
- Jackson AC. Current and future approaches to the therapy of human rabies. Antiviral Res. 2013;99(1):61–67. doi:10.1016/j.antiviral.2013.01.003
- Muller T, Hassel R, Jago M, et al. Rabies in kudu: revisited. Adv Virus Res. 2022;112:115–173. doi:10.1016/bs.aivir.2022.04.001
- Rupprecht CE, Mani RS, Mshelbwala PP, et al. Rabies in the tropics. Curr Trop Med Rep. 2022;9(1):28–39. doi:10.1007/s40475-022-00257-6
- Blanton JD, Rupprecht CE. Travel vaccination for rabies. Expert Rev Vaccines. 2008;7(5):613–620. doi:10.1586/14760584.7.5.613
- Hellgren F, Cagigi A, Arcoverde Cerveira R, et al. Unmodified rabies mRNA vaccine elicits high cross-neutralizing antibody titers and diverse B cell memory responses. Nat Commun. 2023;14(1):3713. doi:10.1038/s41467-023-39421-5
- Warrell MJ. Current rabies vaccines and prophylaxis schedules: preventing rabies before and after exposure. Travel Med Infect Dis. 2012;10(1):1–15. doi:10.1016/j.tmaid.2011.12.005
- Tran CH, Kligerman M, Andrecy LL, et al. Rabies vaccine initiation and adherence among animal-bite patients in Haiti, 2015. PLoS Negl Trop Dis. 2018;12(11):e0006955. doi:10.1371/journal.pntd.0006955
- Senior K. Global rabies elimination: are we stepping up to the challenge? Lancet Infect Dis. 2012;12(5):366–367. doi:10.1016/s1473-3099(12)70096-8
- World Health O. Who expert consultation on rabies. Second report. World Health Organ Tech Rep Ser. 2013;(982):1-139, back cover.
- Stitz L, Vogel A, Schnee M, et al. A thermostable messenger RNA based vaccine against rabies. Plos Neglect Trop D. 2017;11(12). doi:10.1371/journal.pntd.0006108
- Fooks AR, Banyard AC, Ertl HCJ. New human rabies vaccines in the pipeline. Vaccine. 2019;37(Suppl 1):A140–A1A5. doi:10.1016/j.vaccine.2018.08.039
- Amann R, Rohde J, Wulle U, et al. A new rabies vaccine based on a recombinant ORF virus (parapoxvirus) expressing the rabies virus glycoprotein. J Virol. 2013;87(3):1618–1630. doi:10.1128/JVI.02470-12
- Fooks AR, Banyard AC, Horton DL, et al. Current status of rabies and prospects for elimination. Lancet. 2014;384(9951):1389–1399. doi:10.1016/S0140-6736(13)62707-5
- Zhang NN, Zhang RR, Zhang YF, et al. Rapid development of an updated mRNA vaccine against the SARS-CoV-2 omicron variant. Cell Res. 2022;32(4):401–403. doi:10.1038/s41422-022-00626-w
- Laczko D, Hogan MJ, Toulmin SA, et al. A single immunization with nucleoside-modified mRNA vaccines elicits strong cellular and humoral immune responses against SARS-CoV-2 in mice. Immunity. 2020;53(4):724–732. doi:10.1016/j.immuni.2020.07.019
- Lederer K, Castano D, Gomez Atria D, et al. SARS-CoV-2 mRNA vaccines foster potent antigen-specific germinal center responses associated with neutralizing antibody generation. Immunity. 2020;53(6):1281–1295. doi:10.1016/j.immuni.2020.11.009
- Wang Y, Zhang Z, Luo J, et al. mRNA vaccine: a potential therapeutic strategy. Mol Cancer. 2021;20(1):33. doi:10.1186/s12943-021-01311-z
- Sahin U, Kariko K, Tureci O. mRNA-based therapeutics–developing a new class of drugs. Nat Rev Drug Discov. 2014;13(10):759–780. doi:10.1038/nrd4278
- Pascolo S. The messenger's great message for vaccination. Expert Rev Vaccines. 2015;14(2):153–156. doi:10.1586/14760584.2015.1000871
- Pardi N, Hogan MJ, Porter FW, et al. mRNA vaccines - a new era in vaccinology. Nat Rev Drug Discov. 2018;17(4):261–279. doi:10.1038/nrd.2017.243
- Qu L, Yi ZY, Shen Y, et al. Circular RNA vaccines against SARS-CoV-2 and emerging variants. Cell. 2022;185(10):1728. doi:10.1016/j.cell.2022.03.044
- Durymanov M, Reineke J. Non-viral delivery of nucleic acids: insight into mechanisms of overcoming intracellular barriers. Front Pharmacol. 2018: 9. doi:10.3389/fphar.2018.00971
- Fenton OS, Kauffman KJ, McClellan RL, et al. Bioinspired alkenyl amino alcohol ionizable lipid materials for highly potent In vivo mRNA delivery. Adv Mater. 2016;28(15):2939–2943. doi:10.1002/adma.201505822
- Jackson NAC, Kester KE, Casimiro D, et al. The promise of mRNA vaccines: a biotech and industrial perspective. NPJ Vaccines. 2020;5:11. doi:10.1038/s41541-020-0159-8
- Rui Y, Wilson DR, Tzeng SY, et al. High-throughput and high-content bioassay enables tuning of polyester nanoparticles for cellular uptake, endosomal escape, and systemic in vivo delivery of mRNA. Sci Adv. 2022;8(1):eabk2855. doi:10.1126/sciadv.abk2855
- Pardi N, Tuyishime S, Muramatsu H, et al. Expression kinetics of nucleoside-modified mRNA delivered in lipid nanoparticles to mice by various routes. J Control Release. 2015;217:345–351. doi:10.1016/j.jconrel.2015.08.007
- Shi Y, Huang J, Liu Y, et al. Structural and biochemical characteristics of mRNA nanoparticles determine anti-SARS-CoV-2 humoral and cellular immune responses. Sci Adv. 2022;8(47):1827. doi:10.1126/sciadv.abo1827
- Di J, Du Z, Wu K, et al. Biodistribution and non-linear gene expression of mRNA LNPs affected by delivery route and particle size. Pharm Res. 2022;39(1):105–114. doi:10.1007/s11095-022-03166-5
- Yang R, Deng Y, Huang BY, et al. A core-shell structured COVID-19 mRNA vaccine with favorable biodistribution pattern and promising immunity. Signal Transduct Tar. 2021;6(1). doi:10.1038/s41392-021-00634-z
- Chen J, Ye Z, Huang C, et al. Lipid nanoparticle-mediated lymph node-targeting delivery of mRNA cancer vaccine elicits robust CD8(+) T cell response. Proc Natl Acad Sci U S A. 2022;119(34):e2207841119. doi:10.1073/pnas.2207841119
- Persano S, Guevara ML, Li Z, et al. Lipopolyplex potentiates anti-tumor immunity of mRNA-based vaccination. Biomaterials. 2017;125:81–89. doi:10.1016/j.biomaterials.2017.02.019
- Schnee M, Vogel AB, Voss D, et al. An mRNA vaccine encoding rabies virus glycoprotein induces protection against lethal infection in mice and correlates of protection in adult and newborn pigs. Plos Neglect Trop D. 2016;10(6). doi:10.1371/journal.pntd.0004746
- Bai S, Yang T, Zhu C, et al. A single vaccination of nucleoside-modified rabies mRNA vaccine induces prolonged highly protective immune responses in mice. Front Immunol. 2022;13:1099991. doi:10.3389/fimmu.2022.1099991
- Zhang CG, Tian YL, Chen C, et al. Virus-Like vesicles based on Semliki forest virus-containing rabies virus glycoprotein make a safe and efficacious rabies vaccine candidate in a mouse model. J Virol. 2021;95(20). doi:10.1128/JVI.00790-21
- Ren MS, Zhou JJ, Song ZY, et al. Aptamer and RVG functionalized gold nanorods for targeted photothermal therapy of neurotropic virus infection in the mouse brain. Chem Eng J. 2021: 411. doi:10.1016/j.cej.2021.128557
- Wang ZM, Yuan YM, Chen C, et al. Colloidal manganese salt improves the efficacy of rabies vaccines in mice, cats, and dogs. J Virol. 2021;95(23). doi:10.1128/JVI.01414-21
- Luo ZC, Lv L, Li YY, et al. Dual role of toll-like receptor 7 in the pathogenesis of rabies virus in a mouse model. J Virol. 2020;94(9). doi:10.1128/JVI.00111-20
- Zhang YN, Chen C, Deng CL, et al. A novel rabies vaccine based on infectious propagating particles derived from hybrid VEEV-rabies replicon. Ebiomedicine. 2020: 56. doi:10.1016/j.ebiom.2020.102819
- Liang ML, Wang ZM, Wu CJ, et al. A single dose of recombinant VSV-RABV(G) vaccine provides full protection against RABV challenge. Virol Sin. 2022;37(3):455–458. doi:10.1016/j.virs.2022.02.008
- Wang Z, Li MM, Zhou M, et al. A novel rabies vaccine expressing CXCL13 enhances humoral immunity by recruiting both T follicular helper and germinal center B cells. J Virol. 2017;91(3). doi:10.1128/JVI.01956-16
- Aldrich C, Leroux-Roels I, Huang KB, et al. Proof-of-concept of a low-dose unmodified mRNA-based rabies vaccine formulated with lipid nanoparticles in human volunteers: A phase 1 trial. Vaccine. 2021;39(8):1310–1318. doi:10.1016/j.vaccine.2020.12.070
- Andries O, Mc Cafferty S, De Smedt SC, et al. N(1)-methylpseudouridine-incorporated mRNA outperforms pseudouridine-incorporated mRNA by providing enhanced protein expression and reduced immunogenicity in mammalian cell lines and mice. J Control Release. 2015;217:337–344. doi:10.1016/j.jconrel.2015.08.051
- Kariko K, Muramatsu H, Welsh FA, et al. Incorporation of pseudouridine into mRNA yields superior nonimmunogenic vector with increased translational capacity and biological stability. Mol Ther. 2008;16(11):1833–1840. doi:10.1038/mt.2008.200
- Crotty S. T follicular helper cell differentiation, function, and roles in disease. Immunity. 2014;41(4):529–542. doi:10.1016/j.immuni.2014.10.004
- Haberman AM, Gonzalez DG, Wong P, et al. Germinal center B cell initiation, GC maturation, and the coevolution of its stromal cell niches. Immunol Rev. 2019;288(1):10–27. doi:10.1111/imr.12731
- Shlomchik MJ, Weisel F. Germinal center selection and the development of memory B and plasma cells. Immunol Rev. 2012;247:52–63. doi:10.1111/j.1600-065X.2012.01124.x
- Kroese FGM, Wubbena AS, Seijen HG, et al. Germinal-centers develop oligoclonally. Eur J Immunol. 1987;17(7):1069–1072. doi:10.1002/eji.1830170726
- McHeyzer-Williams M, Okitsu S, Wang N, et al. Molecular programming of B cell memory. Nat Rev Immunol. 2012;12(1):24–34. doi:10.1038/nri3128
- Prusty BK, Gulve N, Govind S, et al. Active HHV-6 infection of Cerebellar Purkinje cells in mood disorders. Front Microbiol. 2018;9:1955. doi:10.3389/fmicb.2018.01955
- Abela-Ridder B, Balogh de K, Kessels JA, et al. Global rabies control: the role of international organisations and the global strategic plan to eliminate dog-mediated human rabies. Rev Sci Tech. 2018;37(2):741–749. doi:10.20506/rst.37.2.2837
- Bettini E, Locci M. SARS-CoV-2 mRNA vaccines: immunological mechanism and beyond. Vaccines (Basel). 2021;9(2). doi:10.3390/vaccines9020147
- Schoenmaker L, Witzigmann D, Kulkarni JA, et al. mRNA-lipid nanoparticle COVID-19 vaccines: structure and stability. Int J Pharm. 2021;601:120586. doi:10.1016/j.ijpharm.2021.120586
- Zhang NN, Li XF, Deng YQ, et al. A thermostable mRNA vaccine against COVID-19. Cell. 2020;182(5):1271–1283. doi:10.1016/j.cell.2020.07.024
- Alberer M, Gnad-Vogt U, Hong HS, et al. Safety and immunogenicity of a mRNA rabies vaccine in healthy adults: an open-label, non-randomised, prospective, first-in-human phase 1 clinical trial. Lancet. 2017;390(10101):1511–1520. doi:10.1016/S0140-6736(17)31665-3
- Sun SH, Chen Q, Gu HJ, et al. A mouse model of SARS-CoV-2 infection and pathogenesis. Cell Host Microbe. 2020;28(1):124–133. doi:10.1016/j.chom.2020.05.020
- Lutz J, Lazzaro S, Habbeddine M, et al. Unmodified mRNA in LNPs constitutes a competitive technology for prophylactic vaccines. NPJ Vaccines. 2017;2:29. doi:10.1038/s41541-017-0032-6
- Anderson EJ, Rouphael NG, Widge AT, et al. Safety and immunogenicity of SARS-CoV-2 mRNA-1273 vaccine in older adults. New Engl J Med. 2020;383(25):2427–2438. doi:10.1056/NEJMoa2028436
- Di JX, Du ZL, Wu KZ, et al. Biodistribution and non-linear gene expression of mRNA LNPs affected by delivery route and particle size. Pharm Res-Dordr. 2022;39(1):105–114. doi:10.1007/s11095-022-03166-5
- Yang R, Deng Y, Huang B, et al. A core-shell structured COVID-19 mRNA vaccine with favorable biodistribution pattern and promising immunity. Signal Transduct Target Ther. 2021;6(1):213. doi:10.1038/s41392-021-00634-z
- Yang T, Li C, Wang X, et al. Efficient hepatic delivery and protein expression enabled by optimized mRNA and ionizable lipid nanoparticle. Bioact Mater. 2020;5(4):1053–1061. doi:10.1016/j.bioactmat.2020.07.003
- Perry LL, Lodmell DL. Role of Cd4 + and Cd8+ T-cells in murine resistance to street rabies virus. J Virol. 1991;65(7):3429–3434. doi:10.1128/jvi.65.7.3429-3434.1991
- Tantawichien T, Jaijaroensup W, Khawplod P, et al. Failure of multiple-site intradermal postexposure rabies vaccination in patients with human immunodeficiency virus with low CD4(+) T lymphocyte counts. Clin Infect Dis. 2001;33(10):E122–E1E4. doi:10.1086/324087
- Aldridge RW, Yavlinsky A, Nguyen V, et al. SARS-CoV-2 antibodies and breakthrough infections in the virus watch cohort. Nat Commun. 2022;13(1):4869. doi:10.1038/s41467-022-32265-5
- Gupta RK, Topol EJ. COVID-19 vaccine breakthrough infections. Science. 2021;374(6575):1561–1562. doi:10.1126/science.abl8487
- Qu L, Yi Z, Shen Y, et al. Circular RNA vaccines against SARS-CoV-2 and emerging variants. Cell. 2022;185(10):1728–1744. doi:10.1016/j.cell.2022.03.044
- Ai L, Li Y, Zhou L, et al. Lyophilized mRNA-lipid nanoparticle vaccines with long-term stability and high antigenicity against SARS-CoV-2. Cell Discov. 2023;9(1):9. doi:10.1038/s41421-022-00517-9
- Muramatsu H, Lam K, Bajusz C, et al. Lyophilization provides long-term stability for a lipid nanoparticle-formulated, nucleoside-modified mRNA vaccine. Mol Ther. 2022;30(5):1941–1951. doi:10.1016/j.ymthe.2022.02.001