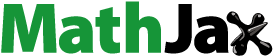
ABSTRACT
Kolaviron (KV) is a biflavonoid isolate mixture of defatted Garcinia kola seed extract patented for treatment of hepatic disorders and inflammation-related conditions. The clinical and experimental-based reports on liver impairment of valproic acid, a front-line synthetic drug used in the treatment of epilepsy gained public attention. Therefore, the mitigative effect of Kolaviron on perturbed redox status and genotoxicity caused by sodium valproate was investigated in murine hepatic tissue. Kolaviron at a dose of 200 mg/kg and sodium valproate (SVP) at a dose of 500 mg/kg were administered orally and consecutively, at an hour interval once a day for two weeks. Treatment with Kolaviron reduced the SVP-increased activities of alanine aminotransferase, aspartate aminotransferase and alkaline phosphatase. Significant increase (P < 0.05) in plasma advanced oxidized protein products (AOPPs) and concomitant reduction in total thiol were observed in liver of SVP-treated rats. Supplementation with Kolaviron significantly reduced the SVP-induced AOPP formation and restored the plasma surfydryl protein level. In addition, Kolaviron ameliorated the SVP-mediated decrease in the activities of glutathione-S-transferase, superoxide dismutase, catalase and reduced glutathione level (P < 0.05). Co-treatment of rats with Kolaviron elicited antigenotoxic effect by inhibiting the induction of micronucleated polychromatic erythrocytesin bone marrow and prevented the formation of DNA fragmentation promoted by SVP. In conclusion, Kolaviron offered protection against sodium valproate-induced genotoxicity, augmented antioxidant status and prevented hepatic oxidative stress in rats.
Introduction
Off-target toxicity of many effective modern day drugs against neurological diseases is a major concern to some patients undergoing treatments, as some drugs have adverse effects on major organs that expressed their metabolizing enzymes in addition to their purported pharmacological activities. Valproic acid (VPA) also known as 2-propylpentanoic acid is a front-line synthetic drug used for treatment of some types of epilepsy and myriads of neurological conditions, such as bipolar disorder, neuropathic pain and migraine [Citation1,Citation2]. It is a choice drug for the treatment of epilepsy and neurodegenerative diseases that affect more than 70 million people worldwide [Citation3,Citation4]. However, administration of VPA could elevate hepatic damage markers in patients as well as in experimental rat models [Citation5,Citation6] and cause definite liver damage that may lead to resultant fatal liver failure [Citation7,Citation8]. VPA was linked with rare fatal hepatotoxicity [Citation9] due to oxidative stress resulting from overproduction of reactive species, and its reactive metabolites were suggested to play a key role in the etiology of its toxicity [Citation10]. Cytochrome P450 metabolize VPA to electrophilic metabolite (E) 2, 4-diene-VPA which was a suggested marker for assessing oxidative stress in liver cells and a major cause of necrotic cell death and hepatotoxicity in human [Citation10]. Since treatment with VPA was associated with oxidative stress through dose-dependent increase in plasma and hepatic level of endogenous lipid peroxidation (LPO) markers [Citation11], it is very important to search for new therapeutic agent that can counteract its undesirable hepatotoxic effects and therefore preserve its desirable therapeutic outcomes.
Kolaviron, a bioflavonoid isolate from Garcinia kola seed, is an important folkloric medicinal plant product in Nigeria. Kolaviron is a patented mixture of Garcinia biflavonoid GB1, GB2 and kolaflavanone for the treatment of mouth infections, inflammation and hepatic disorders [Citation12]. It possessed some pharmacological activities, such as antioxidant, antiinflammatory, antigenotoxic, hepatoprotective and neuroprotective activities in various experimental models via multiple biochemical mechanisms [Citation13,Citation14]. Specifically, several studies have shown the protective ability of Kolaviron against hepatotoxicity induced by aflatoxin B1 [Citation15], Chlorambucil [Citation16], diclofenac [Citation17] and many chemicals and drug-induced liver toxicity. However, the hepatoprotective potential of Kolaviron on VPA-induced liver damage has not been explored.
The present study therefore investigated the protective effects of Kolaviron on the hepatotoxicity induced by VPA and elucidated the underlying mechanisms in order to develop strategies to prevent or alleviate VPA-induced off target liver injury. This may enhance the VPA regimen so that it could be well tolerated with minimal adverse effect .
Materials and methods
Chemicals and reagents
Sodium valproate is a product of Glentham Life Sciences Ltd. Unit 5 Ingoldmells Court Edinburgh Way Corsham Wiltshire, SN13 9XN United Kingdom, 1-chloro-2, 4-dinitrobenzene (CDNB), Glutathione (GSH), 5ʹ,5ʹ-dithio-bis-2-nitrobenzoic acid (DTNB), thiobarbituric acid (TBA), epinephrine and hydrogen peroxide were purchased from Sigma® Chemical Company (London, UK). The alanine aminotransferase (ALT) and aspartate aminotransferase (AST) kits were obtained from Fortress Diagnostics Ltd., Antrim, UK. The extracted Kolaviron was obtained from Dr. Awogbidin of Biochemistry Department, University of Ibadan, Nigeria. Chemicals and reagents used in this study were of analytically standard grade and got from British Drug House Poole UK.
Experimental animals
Twenty four Wistar rats (male) of weight range 160 g-180 g were used for this study. The animals were brought from Veterinary Medicine Department, University of Ibadan and acclimatized for two weeks in the animal house of Department of Chemical Sciences, Ajayi Crowther University, Oyo. They were kept in research plastic cages and fed with standard commercial rat feed and clean tap water being supplied ad libitum. Anesthesia was not involved in this research and the protocol conformed to the guidelines of the National Institute of Health for laboratory animal care and use [Citation18].
Drug treatments and animal grouping
This study employed simple randomized design. After two weeks of adaptation period, the animals were randomly assigned into four main experimental groups of six animals each. The doses of drug and Kolaviron were carefully chosen from literature review [Citation7,Citation19–21] and administered once daily by oral gavage using oral cannular as shown in
Table 1. Experimental design
Collection of blood and liver samples
After 24 hours of final treatment and 2 hours prior to sacrifice, the animals were injected intraperitoneally with 0.04% colchicine (1 mL/100 g body weight). The blood were first collected into lithium heparinized tubes from each animal through retro orbitals plexus and thereafter sacrificed. The bone marrow of femurs was collected for the assessment of bone marrow micronucleus (MN) frequency and livers were also excised for estimation of catalase (CAT), superoxide dismutase (SOD), glutathione-S-transferase (GST), LPO, estimated by quantitation of malondialdehyde (MDA), GSH and percentage DNA fragmentation.
Determination of plasma and liver protein content
The Biuret method of Gornall et al. [Citation22] was used to determine protein concentration in the plasma and liver homogenate after which the protein concentration in the samples was extrapolated from the standard bovine serum albumin curve.
Assay of biomarkers of hepatotoxicity
Biomarkers of hepatotoxicity: activities of ALT and AST were assayed using Fortress Diagnostics kits based on the manufacturer’s procedure. Activities of plasma ALT and AST were determined based on the described principle of Reltman and Frankel [Citation23]. The activity alkaline phosphatase (ALP) was determined in plasma according to Tietz [Citation24]. The p-nitrophenol formed by the hydrolysis of p-nitrophenyl phosphate confers a yellowish color to the reaction mixture, and its intensity can be monitored at 405 nm to give a measure of enzyme activity.
Assay for oxidative stress maker in the plasma
Plasma advanced oxidation protein product (AOPP) was determined by the method described by Witko et al. [Citation25] and modified by Zhang et al. [Citation26]. Plasma total thiol was measured spectrophotometrically using DTNB (2, 2ʹ-dinitro-5, 5ʹ-dithiodibenzoic acid) [Citation27].
Assessment of hepatic biomarkers of oxidative-stress magnitude
The method of Jollow et al. [Citation28] was used to evaluate the level of hepatic-reduced glutathione where 5,5′-dithiobis-(2-nitrobenzoic acid) reacted with reduced glutathione to give a product that possessed molar absorption at wavelength 412 nm. The activity of hepatic GST was carried out following the procedure of Habig et al. [Citation29]. Briefly, the assay mixture (3 mL) was made up of 150 μL volume of CDNB (3.37 mg/mL), 30 μL of reduced GSH (0.1 M), 2.79 mL phosphate buffer (0.1 M, pH 6.5) and 30 μL of liver homogenate. Absorbance at wavelength of 340 nm was measured against the blank after incubation period of 60 seconds.
The activity of SOD in hepatic tissue was determined by the procedure of Misra and Fridovich [Citation30] where inhibition of auto-oxidation of epinephrine at alkaline medium pH 10.2 was being monitored. The procedural method outlined by Sinha [Citation31] was employed to estimate catalase activity in liver based on dichromate reduction in acetic acid to chromic acetate when heated in the presence of hydrogen peroxide (H2O2). The product, chromic acetate, was measured at 570 nm using spectrophotometer. The quantity of H2O2 remained was then extrapolated using the standard curve of H2O2. The unit for activity of catalase was reported as micromole of H2O2 consumed per min per mg protein.
Assay of LPO in liver
The magnitude of LPO in liver homogenate was evaluated as described by Vashney and Kale [Citation32]. The method entailed the spectrophotometric measurement of stable pink compound formed from reaction of malondialdehyde with thiobarbituric acid. LPO is reported in nmole/mg protein as:
where E532 represents molar extinction coefficient for MDA = 1.56 × 105 M − 1 Cm−1
Percentage DNA fragmentation assay
The endonuclease cleavage of the end product of apoptosis was assessed in percentage DNA fragmentation assay following Wu et al. [Citation33]. DNA extracted from homogenate was treated with diphenylamine (DPA). The chromophore formed was measured on the spectrophotometer at 620 nm. Briefly, the livers were homogenized in 10 volumes of Tris-EDTA buffer (TE) pH 8.0 containing Triton-X100. Homogenate was centrifuged at 27,000 g for period of 20 minutes to separate the intact chromatin (pellet named A) from fragmented ones (supernatant named B). The pellet (A) was suspended in Tris-EDTA buffer of pH 8.0 without Triton-X100. 0.5 mL aliquot quantity of pellet and supernatant were placed in different test tubes and 1.5 ml of newly prepared solution of DPA was added to each test tubes. Reaction mixture was incubated at 37°C for 20 hours. Absorbance of mixture was then measured at 620 nm.
Calculation
Quantity of fragmented DNA was estimated by using the formula:
Micronucleus (MN) assay
The clastogenic effects were evaluated in the murine bone marrow using MN assay according to Schmid [Citation34] as the occurring frequency of MN in polychromatic erythrocytes. Bone marrow samples from the two femur bones were used for preparing slides. The slides were fixed in absolute methanol, air-dried and pre-treated with May-Grunewald solution and then stained in 5% Giemsa solution. The slides were blindly scored for micronucleated polychromatic erythrocytes under a light microscope.
Statistical analysis
Data were presented as the mean ± standard deviation (SD) of six replicates. Statistical significance was determined by one-way analysis of variance followed by Duncan’s multiple comparison between control and treated rats in all groups using SigmaPlot® statistical package (Systat Software Inc., San Jose, CA, USA). P-values less than 0.05 (P< 0.05) were considered statistically significant.
Results
Influence of Kolaviron on sodium valproate-induced changes on plasma liver biomarkers of rats
Sodium valproate significantly increased the level of AST, ALT and ALP () by 109.08%, 44.09% and 46.00%, respectively, when compared with the control group (P< 0.05). However, administration of Kolaviron significantly ameliorated the Sodium valproate-induced increase in plasma activities of ALT, AST and ALP in rats.
Figure 2. Protective effects of Kolaviron on sodium valproate-induced changes in the plasma activities of aspartate aminotransferase (a), alanine aminotransferase (b) and alkaline phosphatase (c) in rats. Data represent the means ± SD for six rats in each group; * significantly different from the Control; # significantly different from sodium valproate (P< 0.05)

Effect of Kolaviron on VPA – induced oxidative stress in the plasma of rat
Administration of VPA showed a significant elevation in the concentration of advanced oxidation protein products (AOPPs) present in the blood plasma by 71.42% with concomitant reduction in total thiol content by 30.38% when compared to the control group (). However, co-treatment with Kolaviron significantly (P< 0.05) attenuated the effect of VPA toxicity by reducing the generation of plasma AOPP and caused an increase in thiol protein concentration in the plasma when compared to animal group treated with only sodium valproate.
Figure 3. Effect of Kolaviron on plasma concentration of advanced-oxidized protein products (a) and total thiol (b) in sodium valproate-induced toxicity in Wistar rats. Data represent the means ± SD for six rats in each group; * significantly different from the control; # significantly different from sodium valproate (P< 0.05)

Activity of antioxidant enzymes and non-enzymatic molecules
represents protective effect of Kolaviron co-treatment against sodium valproate-induced changes in hepatic biomarkers of oxidative stress in rats. Hepatic SOD, CAT and GST activities were significantly reduced in the valproate-treated group by 46.24%, 35.81%, and 55.59%, respectively, when compared with values of the control group. The hepatic GSH level was also significantly reduced in animal group treated with VPA alone by 38.47% when compared to control. However, treatment with Kolaviron significantly ameliorated the activities of hepatic SOD, CAT, GST and GSH levels.
Figure 4. Protective effect of Kolaviron co-treatment against sodium valproate-induced changes in hepatic biomarkers of oxidative stress in rats. Data represent the means ± SD for six rats in each group; * significantly different from the Control; # significantly different from sodium valproate (P< 0.05). (a): superoxide dismutase activity, (b): catalase activity, (c): glutathione S-transferase activity, (d): reduced glutathione

Influence of Kolaviron on sodium valproate-induced hepatic LPO in rat
The resultant reduction in hepatic antioxidant status induced by valproate administration was accompanied by a significant increase in the level of LPO in valproate-treated group by 340.70% when compared with the control as shown in . Treatment with Kolaviron significantly attenuated this increase in hepatic MDA as related to valproate group.
Effect of Kolaviron on sodium valproate-induced hepatic percentage DNA fragmentation in rat
The reduced hepatic antioxidant status induced by valproate administration was accompanied by a significantly increased level of percentage DNA fragmentation as depicted in . The level of percentage DNA Fragmentation was significantly increased in valproate-treated group by 56.11% when it was compared with the control. Treatment with Kolaviron significantly attenuated this increase in hepatic percentage DNA fragmentation as compared with valproate alone group.
Antigenotoxic influence of Kolaviron on VPA-induced clastogenicity
The effect of Kolaviron on sodium valproate-induced formation of mPCEs is shown in . Kolaviron treatment did not induce mPCEs. Cotreatment of rats with Kolaviron significantly inhibited the induction of mPCEs by sodium valproate (P< 0.05).
Figure 7. Effects of co-treatment with Kolaviron on frequency of occurrence of micronucleated polychromatic erythrocytes (mPCEs) in rats treated with sodium valproate. Data represent the means ± SD for six rats in each group; * significantly different from the control; # significantly different from sodium valproate (P < 0.05)

Discussion
VPA is an effective antiepileptic drug, but its usage is associated with liver injury that may result in fatal liver failure through oxidative stress and inflammatory mechanisms [Citation7,Citation8]. The present result demonstrated that Kolaviron offers protection against VPA-induced hepatic dysfunction, oxidative stress and biomolecular destructure. Liver seems to be much vulnerable to drug toxicity as it is the main metabolic engine for drugs and xenobiotic in body [Citation35,Citation36]. VPA-mediated hepatotoxicity has been well documented [Citation37] and classified from mere rise in serum liver enzymes and decline in plasma albumin to fatal reaction with toxicological features like microvesicular necrosis and steatosis [Citation9].
Determination of the activities of cytoplasmic enzymes, such as ALT, AST and ALP that leak into the blood circulation provides a reliable assessment of liver function. This is because their leakage into plasma is linked to marked centrilobular degeneration, necrosis and infiltration of the hepatocyte [Citation38]. Although AST measurement is commonly used to assess liver as it is a sensitive indicator of mitochondrial damage especially in the hepatocyte centrilobular regions [Citation39], the determination of ALT is more liver-specific to determine hepatocellular damage [Citation40]. The increased plasma ALP activity has been associated with hepatobiliary injury, overproduction or its leakage [Citation38]. The administration of VPA alone significantly raised the activities of ALT, AST and ALP in the plasma of animals when compared with the control group. This was in agreement with result obtained by other researchers on VPA-induced hepatotoxicity [Citation41,Citation42]. When Kolaviron was co-administered with VPA, the plasma activities of ALP, ALT and AST significantly declined, thereby suggesting the effectiveness of Kolaviron in protecting liver cell integrity and maintaining healthy biliary outflow.
AOPPs which were first detected in chronic uremic patients are dityrosine-containing cross-linked protein products with utility in the evaluation of the degree of oxidant-mediated protein damage [Citation25,Citation43]. AOPPs are a precocious marker of oxidized proteins and circulate for prolonged periods in the blood as cells only degrade them within hours and days. AOPPs when determined in the liver can be considered as a marker for monitoring oxidative stress in drug-induced hepatotoxicity [Citation44]. The generation of intracellular oxidized proteins is associated with increased production of ROS which results from a disruption in the balance between pro-oxidants and antioxidants [Citation45]. It is produced in plasma when the plasma albumin is subjected to oxidation by various oxidants like ROS and hypochlorous acid [Citation46], and its elevated levels have been associated with some pathological conditions, such as atherosclerosis, diabetes, nephropathies and cancer [Citation47]. Sulfhydryl (SH) group has been shown to be sensitive to oxidative damage and its depletion was associated with certain pathological condition-like ischemia insult [Citation48]. The thiol content especially sulfhydryl groups on albumin protein are considered as main antioxidants in plasma or reducing groups in the body fluids. It has been shown that reduced levels of protein thiols correlates negatively with the levels of AOPPs [Citation49]. The significant increase in the level of plasma AOPPs with concomitant reduction in plasma total thiol observed in VPA-treated animal group relative to control in this study suggests the induction of oxidative stress as a result of SVP treatment with subsequent damage to tissue proteins. However, coadministration of biflavonoid Kolaviron significantly reduced the elevated level of AOPPs in drug-treated group and effectively restored the total thiol status when compared to SVP alone treated group. This shows that Kolaviron may improve plasma antioxidant activity by preserving surfydryl protein pools.
A decrease in the level of GSH as well as the activities of hepatic CAT and GST were observed in SVP-treated group. GSH is a tripeptide biomolecule involved in myriads of metabolic processes more importantly in the detoxification of xenobiotics to remove electrophilic metabolites through conjugation catalyzed by GST. The cellular redox status is assessed by reduced glutathione level and the correspondent depletion in GSH level and GST activity has been associated with severe oxidative stress [Citation50,Citation51]. The observed decrease in hepatic activity of GST from this study correlates with the depletion of hepatic GSH, confirming sodium valproate toxicity in liver organ. This is corroborated by the findings of other researchers that have reported the induction of oxidative stress and depletion of antioxidant status by VPA [Citation52,Citation53]. However, Kolaviron supplementation significantly improved the redox balance in the hepatic tissue of the rats by the significant increase observed in the concentration of hepatic GSH and activities of hepatic GST and CAT.
LPO is an important manifestation of oxidative-stress damage that has been known to play a remarkable role in the toxicity of many chemical agents [Citation54]. Biological membrane is made up of phospholipids with polyunsaturated fatty acid tails that was responsible for its fluid property [Citation55]. LPO is initiated by excessive free radical attack on unsaturated fatty acid moiety that leads to a complex series of compounds like lipid peroxides, reactive carbonyl compounds and MDA [Citation56–58]. The result of this study showed a significant decrease in the activities of CAT and SOD in animal group treated with SVP when compared to control group. The enzymatic activities of antioxidant molecule like CAT and SOD are vital in the maintenance of redox balance in the cell [Citation59] and their reduction may expose liver to oxidative stress [Citation24]. This is because endogenous radical like superoxide radical is dismutated to hydrogen peroxide and dioxygen where the former is converted to water and molecular oxygen by catalase [Citation60]. Therefore, the significant increase in LPO observed in the valproate-treated group relative to control may be as a result of reduced activities of SOD and CAT by valproate which exposed the liver to oxidative stress. Moreover, reduction in hepatic SOD activity which leads to accumulation of superoxide anion radical might have responsible for decrease observed in activity of catalase [Citation61]. However, treatment with Kolaviron in this study restored the cellular activities of CAT and SOD which resulted into corresponding significant decrease in hepatic MDA level, a product of LPO. The increased activities of SOD and CAT in Kolaviron administered rats might have resulted from its ability to scavenge the free radicals generated by valproate-induced LPO, thereby decreasing the utilization of these antioxidant enzymes to reduce the oxidative threat induced by the drug. Therefore, KV may reduce the SVP-induced oxidative stress due to its free radical scavenging activity and hence preserved the cellular activity of SOD and CAT.
VPA treatment was reported to cause significant nuclear alterations in normal drug-filtering organs like liver and diminution of some members of the structural maintenance of chromatin (SMC) proteins, SMC-associated proteins, DNA methyltransferase and heterochromatin proteins [Citation62,Citation63]. Findings implicated LPO in DNA damage since many of its products do interact with DNA to induce oxidative DNA damage [Citation64]. More importantly, genotoxicity has been associated with the generation of free radicals [Citation65]. VPA-associated genotoxicity and increase in chromosomal aberration rates have been reported by several authors both in animal models and human population [Citation66–68]. The potential of Kolaviron to prevent vaproic acid genotoxicity using percentage DNA fragments in liver and induction of micronucleated polychromatic erythrocytes as biomarkers was investigated. MN assay is commonly used to determine the genotoxic tendency of myriads of chemicals and antigenotoxic activity of many substances. In this current work, valproate treatment increased the percentage DNA fragmentation in the liver of rats and the occurrence of polychromatic micronucleated cells in bone marrow cells, which suggest that the drug might have induced DNA strand breakage and chromosomal breakage. Kolaviron has earlier been shown to elicit protective effect against the formation of DNA strand breaks and oxidized bases in drug-induced hepatotoxicity [Citation69]. The result of this study clearly indicated that Kolaviron has protective effect against VPA – induced genotoxicity as revealed by the reduced percentage DNA fragmentation and lower frequency of the occurrence of mPCEs in animal group co-administered with Kolaviron. The geno-protective effect of Kolaviron may be through its antioxidant mechanism.
Conclusion
This current work clearly demonstrated that administration of SVP perturbed the antioxidant status of rats toward hepatic impairment and induction of genotoxicity. However, coadministration of Kolaviron offers protection against SVP-induced hepatic impairment and genotoxicity by antioxidative mechanism via inhibition of LPO and augmentation of antioxidant systems. Therefore, dietary supplementation with Kolaviron may protect against hepatic damage by VPA treatment.
Disclosure statement
No potential conflict of interest was reported by the author(s).
References
- Romoli M, Mazzocchetti P, D’Alonzo R, et al. Valproic acid and epilepsy: from molecular mechanisms to clinical evidences. Curr Neuropharmacol. 2019;17(10):926–946.
- Nasrallah H, Ketter T, Kalali A. Carbamazepine and valproate for the treatment of bipolar disorder: a review of the literature. J Affect Disord. 2006;95(1–3):69–78.
- Ngugi A, Bottomley C, Kleinschmidt I, et al. Estimation of the burden of active and life-time epilepsy: a metaanalytic approach. Epilepsia. 2010;51(5):883–890.
- Johannessen CU, Johannessen SI. Valproate : past, present, and future mechanisms of action. CNS Drug Reviews. 2003;9(2):199–216.
- Javad A, Mohammad AK, Seyed AJ, et al. Evaluation of liver enzymes rising in patients treated with sodium valproate (VPA). Int J Pediatr. 2015;3:2–3.
- Gayam V, Mandala AK, Khalid M, et al. Valproic acid induced acute liver injury resulting in hepatic encephalopathya case report and literature review. J Com Hosp Intern Med Perspect. 2018;8(5):311–314.
- Zhu MM, Li HL, Shi LH, et al. The pharmacogenomics of valproic acid. J Hum Genet. 2017;62(12):1009–1014.
- Felker D, Lynn A, Wang S, et al. Evidence for a potential protective effect of carnitine-pantothenic acid co-treatment on valproic acid-induced hepatotoxicity. Expert Rev Clin Pharmacol. 2014;7(2):211–218.
- Spiller H, Krenzelok E, Klein-Schwartz W, et al. Multicenter case series of valproic acid ingestion: serum concentrations and toxicity. J Toxicol Clin Toxicol. 2000;38:755–760.
- Ahangar N, Naderi M, Noroozi A, et al. Zinc deficiency and oxidative stress involved in valproic acid induced hepatotoxicity: protection by zinc and selenium supplementation. Biol Trace Elem Res. 2017;179(1):102–109.
- Tong V, Teng XW, Chang TK, et al. Valproic acid I: time course of lipid peroxidation biomarkers, liver toxicity, and valproic acid metabolite levels in rats. Toxicol Sci. 2005;86(2):427–435.
- Iwu M. Antihepatotoxic constituents of Garcinia kola seed. Experientia. 1985;41(5):699–700.
- Alabi QK, Akomolafe RO, Olukiran OS, et al. Assessment of haematological and biochemical effects of kolaviron in male Wistar rats. Br J Pharm Res. 2017;16(3):1–14.
- Farombi E, Awogbindin I, Farombi T, et al. Neuroprotective role of kolaviron in striatal redoinflammation associated with rotenone model of Parkinson’s disease. Neurotoxicology. 2019;73:132–141.
- Farombi EO, Adepoju BF, Ola-Davies OE, et al. Chemoprevention of aflatoxin B1-induced genotoxicity and hepatic oxidative damage in rats by kolaviron, a natural bioflavonoid of Garcinia kola seeds. Eur J Cancer Prev. 2005;14(3):207–214.
- Olayinka E, Ore A. Kolaviron and L-ascorbic acid attenuate chlorambucil-induced testicular oxidative stress in rats. J Toxicol. 2014;2014:1–9.
- Alabi QR, Akomolafe RO. Kolaviron diminishes diclofenac-induced liver and kidney toxicity in wistar rats via suppressing inflammatory events, upregulating antioxidant defenses, and improving hematological indices. Dose-Response: Int J. 2020; 16(3):1–12.
- Institute for Laboratory Animal Research. Guide for the care and use of laboratory animals. 8th ed. 2011. Epub ahead of print 2011 doi:https://doi.org/10.2307/1525495.
- Adedara IA, Daramola YM, Dagunduro JO, et al. Renoprotection of Kolaviron against benzo (A) pyrene-induced renal toxicity in rats. Ren Fail.2015;37:497–504.
- Omole JG, Ayoka OA, Alabi QK, et al. Protective effect of kolaviron on cyclophosphamide-induced cardiac toxicity in rats. J Evid Based Integr Med. 2018;23:1–11.
- El-shenawy NS, Hamza RZ. Nephrotoxicity of sodium valproate and protective role of L-cysteine in rats at biochemical and histological levels. J Basic Clin Physiol Pharmacol. 2016;27(5):497–504.
- Gornall AG, Bardawill CJ, David MM. Determination of serum proteins by means of the biuret reaction. J Biol Chem. 1949;177(2):751–766.
- Reitman S, Frankel S. A colorimetric method for the determination of serum glutamic oxalacetic and glutamic pyruvic transaminases. Am J Clin Pathol. 1957;28(1):56–63.
- Tietz N, Pruden E, Siggaard-Andersen O. Liver function. In: Burtis A, Ashwood ER, editors. Tietz textbook of clinical chemistry. London, UK: Saunders, WB; 1994. p. 1354–1374.
- Witko-Sarsat V, Friedlander M, Capeillere-Blandin C, et al. Advanced oxidation protein products as a novel marker of oxidative stress in uremia. Kidney Int. 1996;49(5):1304–1313.
- Zhang G, Oleksii AS, Siew-Kim K, et al. Plasma advanced oxidative protein products are associated with anti-oxidative stress pathway genes and malaria in a longitudinal cohort. Malar J. 2014;13. DOI:https://doi.org/10.1186/1475-2875-13-134.
- Motchnik P, Frei B, Ames B. Measurement of antioxidants in human blood plasma. Methods Enzym. 1994;234:269–279.
- Jollow D, Mitchell J, Zampaghone N, et al. Bromobenzene induced liver necrosis, protective role of glutathione and evidence for 3,4-bromobenzene oxide as the hepatotoxic metabolite. Pharmacology. 1974;11(3):151–169.
- Habig WH, Pabst MJ, Jakoby WB. Glutathione S transferases. The first enzymatic step in mercapturic acid formation. J Biol Chem. 1974;249(22):7130–7139.
- Misra HP, Fridovich I. The role of superoxide anion in the autoxidation of epinephrine and a simple assay for superoxide dismutase. J Biol Chem. 1972;247(10):3170–3175.
- Sinha AK. Colorimetric assay of catalase. Anal Biochem. 1972;47(2):389–394.
- Varshney R, Kale RK. Effects of calmodulin antagonists on radiation-induced lipid peroxidation in microsomes. Int J Radiat Biol. 1990;58(5):733–743.
- Wu B, Ootani A, Iwakiri R, et al. T cell deficiency leads to liver carcinogenesis in Azoxymethane-treated rats. Exp Biol Med. 2005;231:91–98.
- Schmid W. Chemical mutagen testing in vivo somatic mammalian cells. Agents Action. 1973;3(2):77–85.
- Bissell D, Gores G, Laskin D, et al. Drug-induced liver injury: mechanisms and test systems. Hepatology. 2001;33(4):1009–1013.
- Pessayre D, Berson A, Fromenty B. Mitochondria in steatohepatitis. Semin Liver Dis. 2001;21(01):57–69.
- Tong V, Teng XW, Chang TK, et al. Valproic acid II: effects on oxidative stress, mitochondrial membrane potential, and cytotoxicity in glutathione-depleted rat hepatocytes. Toxicol Sci. 2005;86(2):436–443.
- Ramaiah SK. A toxicologist guide to the diagnostic interpretation of hepatic biochemical parameters. Food Chem Toxicol. 2007;45(9):1551–1557.
- Panteghini M. Aspartate aminotransferase isoenzymes. Clin Biochem. 1990;23(4):311–319. Penna, C., Mancardi, D., Rastaldo, R. 2009. Cardioprotection: a radical view: free radicals in pre and postconditioning. Biochim Biophys Acta, 1787: 781–793.
- Shyamal S, Latha PG, Shine VJ, et al. Hepatoprotective effects of pittosporum neelgherrense wight&Arn., a popular Indian ethnomedicine. J Ethnopharmacol. 2006;107(1):151–155.
- Nazmy EA, El-khouly OA, Atef H, et al. Sulforaphane protects against sodium valproate-induced acute liver injury. Can J Physiol Pharmacol. 2016;95:420–426.
- Al-Amoudi WM. Protective effects of fennel oil extract against sodium valproate-induced hepatorenal damage in albino rats. Saudi J Biol Sci. 2017;24(4):915–924.
- Alderman C, Shah S, Foreman JC, et al. The role of advanced oxidation protein products in regulation of dendritic cell function. Free Radic Biol Med. 2002;32(5):377–385.
- Afolabi OK, Oyewo EB. Effects of ciprofloxacin and levofloxacin administration on some oxidative stress markers in the rat. World Acad Sci Eng Technol Int J Biol Vet Agric Food Eng. 2014;8:38–42.
- Penna C, Mancardi D, Rastaldo R. Cardioprotection: a radical view: free radicals in pre and postconditioning. Biochim Biophys Acta. 2009;1787(7):781–793.
- Capeillere-Blandin C, Gausson V, Descamps-Latscha B, et al. Biochemical and spectrophotometric significance of advanced oxidized protein products. Biochim Biophys Acta. 2004;1689(2):91–102.
- Descamps-Latscha B, Witko-Sarsat V, Nguyen-Khoa T, et al. Advanced oxidation protein products as risk factors for atherosclerotic cardiovascular events in nondiabetic predialysis patients. Am J Kidney Dis. 2005;45(1):39–47.
- Soszynski M, Bartosz G. Decrease in accessible thiols as an index of oxidative damage to membrane proteins. Free Rad Biol Med. 1997;23(3):463–469.
- Kolagal V, Karanam SA, Dharmavarapu PK, et al. Determination of oxidative stress marker and their importance in early diagnosis of uremia-related complications. Indian J Nephrol. 2009;19(1):8–12.
- Chance B, Sies H, Boveris A. Hydroperoxide metabolism in mammalian organs. Physiol Rev. 1979;59(3):527–605.
- Aniya Y, Naito A. Oxidative stress-induced activation of microsomal glutathione-S-transferase in isolated rat liver. Biochem Pharmacol. 1993;45(1):37–42.
- Chang T, Abbott F. Oxidative stress as a mechanism of valproic acid-associated hepatotoxicity. Drug Metab Rev. 2006;38(4):627–639.
- Silva MFB, Aires CCP, Luis PBM, et al. Valproic acid metabolism and its effects on mitochondrial fatty acid oxidation: a review. J Inherit Metab Dis. 2008;31(2):205–216.
- Buyukokuroglu ME, Cemek M, Yurumez Y, et al. Antioxidative role of melatonin in organophosphate toxicity in rats. Cell Biol Toxicol. 2008;24(2):151–158.
- Catala A. Lipid peroxidation modifies the assembly of biological membranes. The lipid whisker model. Front Physiol. 2015;5. Epub ahead of print. DOI:https://doi.org/10.3389/fphys.2014.00520
- Valenzuela A. The biological significance of malondialdehyde determination in the assessment of tissue oxidative stress. Life Sci. 1990;48:301–309.
- Valko M, Rhodes C, Moncol J, et al. Free radicals, metals and antioxidants in oxidative stress-induced cancer. Chem Biol Interact. 2006;160:1–40.
- Li X, Fang P, Mai J, et al. Targeting mitochondrial reactive oxygen species as novel therapy for inflammatory diseases and cancers. J Hematol Oncol. 2013;6(1):1–19.
- Notas G, Koutroubakis IE, Kouroumalis EA. Oxidants and antioxidants in liver disease. In: Panglossi HV, editor. Antioxidants: new research. New York: Nova Science; 2012. p. 2–48.
- Droge W. Free radicals in the physiological control of cell function. Physiol Rev. 2002;82(1):47–95.
- Boone L, Meyer D, Cusick P. Selection and interpretation of clinical pathology indicators of hepatic injury in preclinical studies. Vet Clin Path. 2005;34(3):182–188.
- Marchion D, Bicaku E, Daud A, et al. Valproic acid alters chromatin structure by regulation of chromatin modulation proteins. Cancer Res. 2005;65(9):3815–3822.
- Kortenhorst M, Isharwal S, Van Diest P, et al. Valproic acid causes dose- and time-dependent changes in nuclear structure in prostate cancer cells in vitro and in vivo. Mol Cancer Ther. 2009;8(4):802–808.
- Agarwal S, Draper H. Isolation of a malondialdehyde-deoxyguanosine adduct from rat liver DNA. Free Rad Biol Med. 1992;13(6):695–699.
- Mitchell L, Adzick N, Melchionne J, et al. Spina bifida. Lancet. 2004;364(9448):1885–1895.
- Denli M, Aydin H, Dundaroz R, et al. Genotoxicity evaluation in female patients on valproic acid monotherapy using alkaline single cell gel electrophoresis (comet assay. East J Med. 2000;5:61–65.
- Witczak M, Kociszewska I, Wilczynski J, et al. Evaluation of chromosome aberrations, sister chromatid exchange and micronuclei in cultured cord-blood lymphocytes of newborns of women treated for epilepsy during pregnancy. Mutat Resh Genet Toxicol Env Mutagen. 2010;701(2):111–117.
- Sanaa R, Galaly E, Abdella M, et al. Effects of royal jelly on genotoxicity andnephrotoxicity induced by valproic acid in albino mice. Beni-suef Univ J Basic Appl Sci. 2014;3(1):1–15.
- Farombi EO. Genotoxicity of chloroquine in rat liver cells: protective role of free radical scavengers. Cell Biol Toxicol. 2006;22(3):159–167.