Comment on: Yamaguchi, H. Murphy, K.R. Fukatsu, N. Sato, K. Yamanaka, A. and de Lecea, L. (2023). Dorsomedial and preoptic hypothalamic circuits control torpor. Curr. Biol. 33, 5381–5389.e4.
Endothermic animals, including humans, generate metabolic heat to maintain a stable internal temperature, enabling activity in cold environments but at the cost of requiring substantial food as an energy source. Consequently, during the winter, when temperatures are low and food scarcity is challenging, some endotherms voluntarily enter a hypoactive metabolic state known as torpor to survive by conserving energy. Animals that enter torpor reduce their body temperature and lower their heart rate and respiration to a fraction of the normal levels, from half to just a few percent. Torpor is classified by its duration into “hibernation” and “daily torpor” (diurnal hypometabolism). The definition of hibernation is entering torpor for a period ranging from several days to weeks during the winter season, while daily torpor is defined as torpor that ends within 24 hours. Over 200 animal species, including birds and rodents, are known to enter torpor. However, the mechanisms underlying the regulation of torpor and hibernation remain largely elusive.
When fasted in a cold environment, mice temporarily reduce their body temperature and basal metabolism, entering daily torpor. Before the drop in body temperature, a decrease in oxygen consumption and carbon dioxide production can be observed, indicating that mice in torpor suppress thermogenesis. During torpor, mice take a curled-up posture and constrict blood vessels near the body surface, including the limbs and tail. Thus, it can be inferred that mice physiologically suppress heat production and dissipation during torpor to conserve energy. The use of laboratory mice, widely employed in experimental biology, as a model for torpor enables expediting the investigation into the mechanisms underlying torpor.
The central nervous system, especially the several nucleus in hypothalamus, is believed to converge internal nutritional status, environmental temperature, and circadian rhythms to regulate the onset, duration, and termination of torpor. However, the detailed neural underpinnings of these processes had long needed to be clarified. In recent years, with the vast advancement of techniques for manipulating neural activity such as optogenetics, chemogenetics, and the creation of transgenic animals, researchers, including us, have started uncovering some of the neural circuits that control torpor [Citation1–5]. In this paper, we will introduce our latest findings related to the hypothalamic mechanisms controlling torpor and provide an overview of the future challenges and prospects in the field.
To comprehensively identify the neuronal circuits controlling torpor, we performed a whole-brain screen for torpor-activated neurons with optically clear, intact brain volume imaging [Citation1]. As a result, we found that the antero-preoptic area (anterodorsal preoptic area and anteroventral preoptic area), medial preoptic area (MPA), paraventricular nucleus, and dorsomedial hypothalamus (DMH) were all strongly activated before and during torpor. These findings align with the previous studies that show the preoptic areas [Citation2] and the DMH [Citation3,Citation4] are implicated in the regulation of torpor. Following genetic silencing of the activity of Vgat-expressing GABAergic neurons in the DMH or Trpm2-expressing neurons in the MPA led to the absence of significant body temperature reductions during torpor, indicating the essential role of these neurons in thermoregulation during torpor.
Fasted mice enter torpor in the latter part of the dark phase, suggesting that the circadian clock regulates the timing of torpor entrance. Where are the inputs regarding environmental temperature, internal nutritional status, and circadian clock converged? Neurons in the DMH are remarkable because they receive projections from both the arcuate nucleus (Arc), responsible for detecting peripheral fasting cues, and the suprachiasmatic nucleus (SCN), the central circadian pacemaker. This positioning designates them as a hub for orchestrating torpor regulation (). Furthermore, it might be possible that the DMH directly receives the internal nutritional status from peripheral tissues such as the stomach and adipose tissues via the bloodstream because many of the DMH neurons express the ghrelin and/or leptin receptors (). An engineered CRISPR/Cas9 system allows us to knock down a specific gene in genetically-defined neurons [Citation6]. In future research, it would be intriguing to investigate the phenotype of torpor after cell-type specific knockdown of these receptors in the DMH Vgat-expressing neurons.
Figure 1. The Vgat-expressing neurons in the DMH serve as an intermediary hub in the pathway connecting the MPA to the Raphe Pallidus (RPa), potentially converging information on internal nutritional status and circadian rhythms. The Vgat-expressing DMH neurons project to both the MPA and RPa. Conversely, the DMH receives projections from the MPA, SCN, and Arc. Moreover, the DMH expresses receptors for leptin and ghrelin, suggesting the possibility of directly receiving signals of internal nutritional signal from the bloodstream.
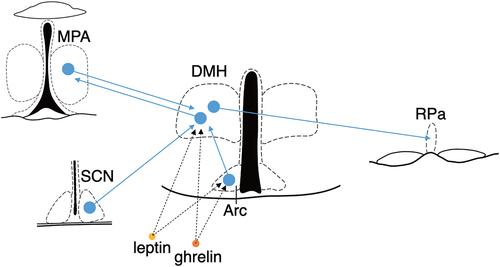
Torpor encompasses a multifaceted array of behaviors designed to minimize energy expenditure, wherein animals not only curtail heat production but also mitigate heat dissipation from the body surface by vasoconstriction and curled-up posture. While our optogenetic stimulation of Trpm2-expressing neurons in the MPA induced hypothermia, it fell short in eliciting the characteristic curled-up posture seen in natural torpor. Instead, an increase in locomotor activity was observed, potentially serving as a compensatory mechanism to counterbalance the induced hypothermia. Also, the magnitude of the decrease in body temperature was smaller than that observed during natural torpor. These results indicated that the activation of Trpm2-expressing neurons in the MPA is insufficient to induce torpor. To date, including our research, no single neural nucleus capable of replicating all features of torpor through its activation has been identified. A combination of activations and deactivations of multiple neural nuclei likely regulates a full expression of torpor. Our whole-brain screening has identified brain areas beyond the MPA and DMH that are activated or deactivated during torpor, suggesting they contribute to future research on torpor.
Using mice as a torpor model has significantly advanced the elucidation of neural circuits controlling torpor. However, daily torpor in mice, unlike hibernation in real hibernators such as squirrels and hamsters, exhibits shallow and brief reductions in body temperature. Are the neural circuits regulating daily torpor and hibernation the same? If so, how is the depth and duration of temperature reduction during torpor regulated? Furthermore, are these neural circuits conserved in animals that do not enter torpor, such as humans? Therefore, future comparative neuroscientific research across hibernating and non-hibernating species will be necessary. With recent advancements in gene editing technology, neuron-type-specific manipulations in various animal species are feasible, leading to a deeper understanding of the mechanisms underlying torpor and hibernation.
References
- Yamaguchi H, Murphy KR, Fukatsu N, et al. Dorsomedial and preoptic hypothalamic circuits control torpor. Curr Biol. 2023;33(24):5381–5389.e4. DOI:10.1016/j.cub.2023.10.076
- Hrvatin S, Sun S, Wilcox OF, et al. Neurons that regulate mouse torpor. Nature. 2020;583(7814):115–121. DOI:10.1038/s41586-020-2387-5
- Ambler M, Hitrec T, Wilson A, et al. Neurons in the dorsomedial hypothalamus promote, prolong, and deepen torpor in the mouse. J Neurosci. 2022;42(21):4267–4277. DOI:10.1523/JNEUROSCI.2102-21.2022
- Hitrec T, Luppi M, Bastianini S, et al. Neural control of fasting-induced torpor in mice. Sci Rep. 2019;9(1):15462. DOI:10.1038/s41598-019-51841-2
- Takahashi TM, Sunagawa GA, Soya S, et al. A discrete neuronal circuit induces a hibernation-like state in rodents. Nature. 2020;583(7814):109–114. DOI:10.1038/s41586-020-2163-6
- Yamaguchi H, Hopf FW, Li S-B, et al. In vivo cell type-specific CRISPR knockdown of dopamine beta hydroxylase reduces locus coeruleus evoked wakefulness. Nat Commun. 2018;9(1):5211. DOI:10.1038/s41467-018-07566-3