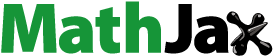
ABSTRACT
The performance of the simultaneous wireless information and power transfer (SWIPT) enabled full-duplex (FD) relaying in non-orthogonal multiple access (NOMA) networks is investigated in both reliability and security aspects. More precisely, for the viewpoint of reliability, we derive in the closed-form expression the outage probability (OP) at both end-users. On the other hand, intercept probability (IP) is considered a helpful metric to measure the security of the considered systems. Moreover, we derive the IP in the closed-form expression too. Numerical results are also given to confirm the correctness of the derived mathematical framework as well as to identify the insights of both metrics as a function of some key parameters such as the transmit power, the power-splitting (PS) ratio, and the power allocation ratio.
1. Introduction
Recently, simultaneous wireless information and power transfer (SWIPT) has gained lots of attraction from both industry and academia thanks to its capabilities to both bear information and charge a battery of low energy devices (LEDs) (Li et al., Citation2020). To make it feasible, the SWIPT-enabled receiver needs to equip an energy harvesting (EH) circuit besides the conventional information decoding circuit. The EH circuit can be made by low-cost passive devices such as resistance and capacitors or from an advanced microprocessor. Additionally, relying on the adopted protocol some other hardware is required. For example, if the power splitting (PS) protocol is taken into consideration, a power splitter is used to divide the incoming signals into two parts. The first part is sent to the EH circuit and the remained part is guided to the information decoding (ID) receiver (Lam et al., Citation2016b). On the other hand, if the time-switching (TS) protocol is used, an electronic switch is mandatory to realize this protocol so that the switch will periodically switch between the EH and ID receivers. Finally, if the receiver is equipped with multiple antennae, an antenna-splitting (AS) protocol can be applied where parts of the antennae are for EH and the remaining is for ID (Lam et al., Citation2016a).
On the other hand, full-duplex (FD) communications (T. N. Nguyen, Tu, et al., Citation2022) and non-orthogonal multiple access (NOMA) are also effective techniques to boost the spectral efficiency (SE) and energy efficiency (EE) of the wireless networks (Bariah et al., Citation2019). In particular, by allowing simultaneous transmitting and receiving of information, FD communications can doubly improve the SE of the whole network provided that the self-interference is appropriately suppressed. It is obvious that the combinations of full-duplex with SWIPT can not only improve the spectral efficiency (SE) but also energy efficiency (EE). Its advantages was proven in Yang et al. (Citation2022), Wang et al. (Citation2017) and Phu et al. (Citation2021b). Particularly, in Yang et al. (Citation2022), the authors designed the transceiver supporting FD SWIPT-enabled networks. They showed that the proposed design achieves lower transmit power compared with FD without SWIPT-enabled networks. The capacity and outage probability of the FD SWIPT-based networks were significantly improved in Wang et al. (Citation2017) and Phu et al. (Citation2021b). NOMA which allows multiple signals concurrently transmit on the same resource blocks (time and frequency) will scale up both the SE and EE of the networks. As a consequence, in this work, we investigate the performance of the SWIPT-enabled NOMA-based wireless networks. Before going to discuss the contributions and novelties of the current work, let us first summarize the state-of-the-art of these techniques.
The performance of FD communications, NOMA-based and SWIPT-supported wireless networks were extensively given in Kurup and Babu (Citation2020), Li et al. (Citation2020), Liu et al. (Citation2019), Liu et al. (Citation2020), T. N. Nguyen et al. (Citation2019), Nguyen et al. (Citation2022a), B. C. Nguyen et al. (Citation2019), Tran et al. (Citation2021), Tu et al. (Citation2018), Tu et al. (Citation2023) and Wu et al. (Citation2019). More precisely, the authors in (Kurup & Babu, Citation2020) studied the adaptive power allocation (APA) in the SWIPT-based full duplex cooperative NOMA networks by employing the TS protocol. Their work, nonetheless, focuses on the reliability problem rather than considering both reliability and security like the present work. Outage probability (OP) under the TS protocol was investigated in Li et al. (Citation2020) where the imperfect channel state information (CSI) and hardware impairments were taken into account. They, however, do not derive the intercept probability (IP). The OP under the SWIPT-based cooperative NOMA networks was addressed in Liu et al. (Citation2019) where the near user acts as a relay for the far user. Their work does not consider the full-duplex transmission owing to the single antenna at all nodes. A non-linear energy harvester was considered in Liu et al. (Citation2020) to capture the non-linear behaviour of the harvested energy. Nevertheless, they also do not study the IP like the current work. The performance of the partial and full relay selection under the EH-enabled two-way relaying networks was investigated in Nguyen et al. (Citation2019a), Nguyen et al. (Citation2019b),and Nguyen et al. (Citation2022a). Nguyen and other authors in B. C. Nguyen et al. (Citation2019) derived the OP of NOMA networks. Tran and others studied the performance of cognitive radio networks (CRNs) subject to the joint impact of interference and hardware noises (Tran et al., Citation2021). Tools from stochastic geometry were applied in Tu et al. (Citation2018) to study the coverage probability of receiver diversity in cellular networks with SWIPT. The impact of imperfect interference cancellation and near field path-loss on the performance of OP was conducted in Tu et al. (Citation2023). They, nonetheless, do not study the security aspect of NOMA networks as well as energy harvesting. Wu and other authors proposed a novel transceiver design for the downlink SWIPT-enabled NOMA systems in Wu et al. (Citation2019).
Different from the above-mentioned works, in the present paper, we investigate the trade-off between the security and reliability of the SWIPT-enabled full-duplex relaying NOMA networks. In particular, we derive both the OP and IP at all destinations in the closed-form expressions. The main contributions and novelties are given as follows:
We take into account several advanced techniques that are full-duplex relaying, NOMA, and SWIPT. The considered systems, as a consequence, are complicated and involve many random variables (RVs) that are not necessarily independent of each other.
We derive in closed-form expression both the OP and IP of all destinations.
We provide simulation results from the Monte-Carlo method to verify the accuracy of the derived mathematical framework.
We highlight the impact of some key parameters on the system performance.
The remainder of the manuscript is organized as follows: Section 2 provides the system model. The main derivations and trends are given in Section 3. Numerical results are provided in Section 4. Section 5 concludes the paper.
2. System model
depicts the system model of the proposed networks, in which a source S communicates with two users denoted by D1 and D2 (D1: weak user and D2: strong user) via the help of a full-duplex relay denoted by R.Footnote1 Besides, there is an eavesdropper denoted by E attempting to wiretap the secure information at R, D1, and D2. It is assumed that the direct link between S and two users does not exist owing to long transmission distance and deep shadow fading (Minh Nam et al., Citation2022). Since the relay is not connected to the power grid, it counts only on the harvested energy from S to operate and forward information to two destinations. It is noted that all nodes in the considered networks are equipped with an antenna except for the relay which is equipped with two antennae. It is noted that if the eavesdropper is equipped with multiple antennae, the wiretap channel will be enhanced. As a consequence, the intercept probability will go up and the systems will be less secure. One of the effective ways to mitigate such cases is to employ artificial noise from the source node and/or the destination to deteriorate the quality of the eavesdropper links. On the other hand, multiple antennae at eavesdropper have a tiny effect on the performance of the outage probability since the signal-to-noise-ratio (SNR) at both destinations is independent of the eavesdropper. As a consequence, the relay node is able to work in the full-duplex mode while all remaining operate in the half-duplex mode.
2.1. Channel modelling
Both large-scale path-loss and small-scale fading are studied in this paper. The impact of shadowing is not taken into account as it is a general case in the literature (Lam & Di Renzo, Citation2020).
2.1.1. Small-scale fading
Let us denote as the channel coefficients from S → R, from R →
, and from R → E, respectively. We also denote
as the self-interference from the transmit antenna to the receive antenna at the relay node. Assuming that
are followed by a complex Gaussian distribution, their channel gains denoted by
are then modelled by an exponential distribution whose cumulative distribution function (CDF) are given as Pham et al. (Citation2020)
(1)
(1) where
are the average channel gain from S to R, from R to two destinations, and from R to E that are a function of the transmission distance defined in the following Section. In the present work, we assume that the global channel state information of all nodes is available at the source node via a high-accuracy feedback network. Nonetheless, it is true that obtaining the global CSI in wireless networks is an extreme task, thus, considering imperfect CSI is a promising extension for the current work. However, it is proven in Tu et al. (Citation2022) that the negative impact of the imperfect CSI can be effectively mitigated by employing multiple antennae and/or multiple relays.
2.1.2. Large-scale path-loss
Let us denote as the large-scale path-loss from node
to node
and is given as follows (N.-L. Nguyen et al., Citation2023):
(2)
(2) where
is the transmission distance from node a to node b and β is the path-loss exponent.
2.2. Transmission procedure
The transmission commences with the transmission from the source S to the relay R. At the relay, it employs one of its antennae to both decode information and harvest energy thanks to the power splitting protocol in the simultaneous wireless information and power transfer enabled systems. Particularly, the incoming signals are split into two parts. The first part is sent to the harvesting circuit which will absorb and store the harvested energy in a super capacity battery. The harvested energy denoted by is formulated as
(3)
(3) where
is the conversion efficiency,
is the PS ratio, T is the transmission duration (in second),
is the transmit power of S, and
is the channel gain from S to R. The transmit power of R is then formulated as
(4)
(4) Besides, the remained part is sent to the information decoding circuit, the received signal denoted by
at the input of this circuit is given below
(5)
(5) where
is the transmitted signal from S which is superimposed of two signals for
and
;
,
are the power allocation for the destination
and
, respectively. Here, the near and far users are identified based on the large-scale path-loss criteria. Particularly, a user who enjoys the larger path-loss is labelled as the far user and another will be the nearer. In the present work, without any explicit explanations, we assume that
is the far user. Other criteria such as one based on simultaneously received power are left for future work.
is the self-interference at R owing to the full-duplex mode and the imperfect cancellation;
is the transmitted signal from relay;
;
is the expectation operator.
is the additive white Gaussian noise. Based on the successive interference cancellation (SIC) principle, the relay first decodes the signal
while considering
as the background noise. It then removes the signal
from the received signal and decodes the signal
. Therefore, the instantaneous signal-to-interference-plus-noise-ratio (SINR) of signals
and
at R are expressed as
(6)
(6) where
is the noise variance. Next, substituting (Equation4
(4)
(4) ) into (Equation6
(6)
(6) ), we have
(7)
(7) After decoding the information of both destinations, the relay re-encodes and forwards this information to both destinations. The received signals at two destinations are then given as
(8)
(8) Here
is the AWGN at the ith destination; the SINR at
is formulated as
(9)
(9) and the SNR of
is held by first subtracting the
and is given as
(10)
(10) Finally, the instantaneous rate of
is computed as
(11)
(11)
3. Performance analysis
In this section, we evaluate the performance of the reliability and security of the considered networks. More precisely, outage probability is chosen as a key metric of the reliability while intercept probability is a representation from the security aspect.
3.1. Outage probability
Outage probability is referred to the probability that the instantaneous rate is below the predefined threshold. Particularly, the OP of the ith destination is mathematically computed as
(12)
(12)
Proof.
Let us commence with the derivation of the , we have
(13)
(13) where
, (a) is held by employing the independent properties of two hops.
and
are given as follows:
(14)
(14) where
is the modified Bessel function of second kind with the 1st order. Finally, by substituting
and
from (Equation14
(14)
(14) ) into (Equation13
(13)
(13) ), we obtain the OP of
. Next, we derive the OP of
as follows:
(15)
(15) where
(16)
(16) We conclude the proof here.
3.2. Intercept probability
The considered system will be wiretapped if E can successfully decode or
from the relay. Mathematical speaking, the IP of
and
is computed as follows:
(17)
(17) Here the last equation is achieved by applying the same steps as the outage probability.
4. Numerical results
Simulation results based on the Monte-Carlo method as T. N. Nguyen et al. (Citation2018) are given in this section to clarify the accuracy of the developed mathematical frameworks as well as to give some insights into the considered metrics. Without loss of generality, the following set of parameters is used in this section: β = 2.5, η = 0.8, R = 0.125 [bits/s/Hz], ,
, ρ = 0.5,
= 0.5,
= 0.75,
= 0.35, and
= 3.
and depict the OP and IP versus . We observe that there is a good agreement between the developed mathematical framework and the Monte-Carlo simulation in both figures. Additionally, increasing
is beneficial for the
but not necessarily for the
. In particular, when
increases from 0.55 to 0.95, the OP of
steadily decreases from 0.2 to below 0.1 while OP of
dramatically scales up from 0.08 to above 0.2. In , we also observe a contrary behaviour of
and
regarding
. More precisely, we see that increasing
, the security of
improves significantly while the security of
is gradually lost.
Figure 2. Outage probability vs. ; solid lines are from Monte-Carlo simulation while markers are from (Equation12
(12)
(12) ).
![Figure 2. Outage probability vs. α1; solid lines are from Monte-Carlo simulation while markers are from (Equation12(12) OPD1=1−2[1−exp(−λRR(1−ρ)[α1−γthα2]γthηρ)]×γthλSRλRD1ηρ(α1−α2γth)K1(2γthλSRλRD1ηρ(α1−α2γth))OPD2=1−2(1−exp(−λRR(1−ρ)α2ηργth))λSRλRD2γthα2ηρΨK1(2λSRλRD2γthα2ηρΨ).(12) ).](/cms/asset/1176c9a4-0350-4a24-b8ba-3a82edba51f2/tjit_a_2218046_f0002_oc.jpg)
Figure 3. Intercept probability vs. ; solid lines are from Monte-Carlo simulation while markers are from (Equation17
(17)
(17) ).

Figure 4. Outage probability vs. Ψ [dB]; solid lines are from Monte-Carlo simulation while markers are from (Equation12(12)
(12) ).
![Figure 4. Outage probability vs. Ψ [dB]; solid lines are from Monte-Carlo simulation while markers are from (Equation12(12) OPD1=1−2[1−exp(−λRR(1−ρ)[α1−γthα2]γthηρ)]×γthλSRλRD1ηρ(α1−α2γth)K1(2γthλSRλRD1ηρ(α1−α2γth))OPD2=1−2(1−exp(−λRR(1−ρ)α2ηργth))λSRλRD2γthα2ηρΨK1(2λSRλRD2γthα2ηρΨ).(12) ).](/cms/asset/21ec98d7-107b-4d85-8617-e2d732a5352c/tjit_a_2218046_f0004_oc.jpg)
and illustrate the performance of the outage probability and intercept probability with respect to the transmit power of source S. We experience again that the derived mathematical frameworks have coincided with the simulation results. Furthermore, surging the transmit power is useful for both OP and IP. More precisely, the OP improves 100 times when the transmit power increases from −5 to 20 dB for two destinations. Looking at , we observe that is substantially better than
, and the gap between the two curves is almost stable for the whole range of observation. Regarding IP, we see that increasing Ψ will increase IP regardless of destinations. However, the
seems to increase quicker than
especially when the system is in the low transmit power regime. Finally, from and , it is important to optimize the transmit power of the source node since we experience a contrary behaviour of the reliability and security aspects.
Figure 5. Intercept probability vs. Ψ [dB]; solid lines are from Monte-Carlo simulation while markers are from (Equation17(17)
(17) ).
![Figure 5. Intercept probability vs. Ψ [dB]; solid lines are from Monte-Carlo simulation while markers are from (Equation17(17) IPx1=Pr(CEx1≥R)=1−Pr(CEx1<R)=1−Pr(α1ηρΨγSRγRE<γth)=2λSRλREγthα1ηρΨK1(2λSRλREγthα1ηρΨ),IPx2=1−Pr(CEx2<R)=1−Pr(α2ηρΨγSRγRE<γth)=2λSRλREγthα2ηρΨK1(2λSRλREγthα2ηρΨ).(17) ).](/cms/asset/b3120fe2-8a82-4e8c-af26-cb96f63da5ae/tjit_a_2218046_f0005_oc.jpg)
Figure 6. Outage probability vs. ρ; solid lines are from Monte-Carlo simulation while markers are from (Equation12(12)
(12) ).
![Figure 6. Outage probability vs. ρ; solid lines are from Monte-Carlo simulation while markers are from (Equation12(12) OPD1=1−2[1−exp(−λRR(1−ρ)[α1−γthα2]γthηρ)]×γthλSRλRD1ηρ(α1−α2γth)K1(2γthλSRλRD1ηρ(α1−α2γth))OPD2=1−2(1−exp(−λRR(1−ρ)α2ηργth))λSRλRD2γthα2ηρΨK1(2λSRλRD2γthα2ηρΨ).(12) ).](/cms/asset/69bbd9de-c9bd-41a2-b558-f0fd76f9f9e8/tjit_a_2218046_f0006_oc.jpg)
and show the impact of the power-splitting ratio ρ on the performance of the OP and IP. In , we see that the OP has a U-shape behaviour with respect to ρ. Particularly, OP starts decreasing when ρ increases after reaching its minimum, it keeps increasing. Interestingly, the OP of does not consistently outperform the OP of
. As for the IP, we observe a different behaviour regarding ρ. More precisely, increasing ρ monotonically increases IP.
Figure 7. Intercept probability vs. ρ; solid lines are from Monte-Carlo simulation while markers are from (Equation17(17)
(17) ).

Figure 8. Outage probability vs. Intercept Probability; solid lines and markers are from (Equation12(12)
(12) ) and (Equation17
(17)
(17) ).
![Figure 8. Outage probability vs. Intercept Probability; solid lines and markers are from (Equation12(12) OPD1=1−2[1−exp(−λRR(1−ρ)[α1−γthα2]γthηρ)]×γthλSRλRD1ηρ(α1−α2γth)K1(2γthλSRλRD1ηρ(α1−α2γth))OPD2=1−2(1−exp(−λRR(1−ρ)α2ηργth))λSRλRD2γthα2ηρΨK1(2λSRλRD2γthα2ηρΨ).(12) ) and (Equation17(17) IPx1=Pr(CEx1≥R)=1−Pr(CEx1<R)=1−Pr(α1ηρΨγSRγRE<γth)=2λSRλREγthα1ηρΨK1(2λSRλREγthα1ηρΨ),IPx2=1−Pr(CEx2<R)=1−Pr(α2ηρΨγSRγRE<γth)=2λSRλREγthα2ηρΨK1(2λSRλREγthα2ηρΨ).(17) ).](/cms/asset/ceaea866-67b2-4cd4-8ba3-f3c6ba686275/tjit_a_2218046_f0008_oc.jpg)
studies the interaction between OP and IP. We observe that the smaller the OP the larger the IP. As a consequence, it is important to optimize a set of parameters that simultaneously satisfies both the reliability and security aspects of the networks.
5. Conclusion
In the present work, we studied both the intercept and outage probability of the dual-hop SWIPT-enabled networks. In particular, we derived the outage probability (OP) and intercept probability (IP) of the considered networks in the closed-form expressions. Simulation results were given to confirm the correctness of the derived frameworks. Finally, the performance of the considered networks can be enhanced in several ways. One of the feasible extensions is to consider the random position of all nodes in the networks via tools from stochastic geometry. Additionally, the imperfect cancellation at the relay could also be considered to capture the practical hardware constraint. Another possible extension was to install more antennae at both source and destinations to further improve the system's reliability and security.
Disclosure statement
No potential conflict of interest was reported by the author(s).
Additional information
Funding
Notes
1 Although reconfigurable intelligent surfaces (RIS) has recently emerged as a potential technique to enhance the system performance of the wireless networks we here employ the relay owing to the following reasons. First, it is certain that both RIS and relaying are effective means to ameliorate wireless networks. However, to truly exploit the benefit of these techniques, global channel state information should be available at the central node, i.e. source node. Nonetheless, estimating channel coefficients in RIS-assisted networks is more challenging compared to relaying networks since most of the RIS elements are made up of passive devices (Van Chien et al., Citation2021). Regarding the relay, ones can straightforwardly estimate the channel coefficient without any problems. Next, another difference between these two techniques is that the RIS is generally classified as a passive device while the relay is an active device with full signal processing functions as well as a power amplifier.
References
- Bariah, L., Muhaidat, S., & Al-Dweik, A. (2019). Error probability analysis of NOMA-based relay networks with SWIPT. IEEE Communications Letters, 23(7), 1223–1226. https://doi.org/10.1109/COML.4234
- Kurup, R. R., & Babu, A. V. (2020). Power adaptation for improving the performance of time switching SWIPT-based full-duplex cooperative NOMA network. IEEE Communications Letters, 24(12), 2956–2960. https://doi.org/10.1109/COML.4234
- Lam, T. T., & Di Renzo, M. (2020). On the energy efficiency of heterogeneous cellular networks with renewable energy sources–A stochastic geometry framework. IEEE Transactions on Wireless Communications, 19(10), 6752–6770. https://doi.org/10.1109/TWC.7693
- Lam, T. T., Di Renzo, M., & Coon, J. P. (2016a). System-level analysis of receiver diversity in SWIPT-enabled cellular networks. Journal of Communications and Networks, 18(6), 926–937. https://doi.org/10.1109/JCN.5449605
- Lam, T. T., Di Renzo, M., & Coon, J. P. (2016b). System-level analysis of SWIPT MIMO cellular networks. IEEE Communications Letters, 20(10), 2011–2014. https://doi.org/10.1109/LCOMM.2016.2590424
- Li, X., Li, J., & Li, L. (2020). Performance analysis of impaired SWIPT NOMA relaying networks over imperfect weibull channels. IEEE Systems Journal, 14(1), 669–672. https://doi.org/10.1109/JSYST.4267003
- Liu, Y., Ding, H., Shen, J., Xiao, R., & Yang, H. (2019). Outage performance analysis for SWIPT-based cooperative non-orthogonal multiple access systems. IEEE Communications Letters, 23(9), 1501–1505. https://doi.org/10.1109/COML.4234
- Liu, Y., Ye, Y., Ding, H., Gao, F., & Yang, H. (2020). Outage performance analysis for SWIPT-based incremental cooperative NOMA networks with non-linear harvester. IEEE Communications Letters, 24(2), 287–291. https://doi.org/10.1109/COML.4234
- Minh Nam, P., Hung, H. D., Tu, L. -T., P. V. Tuan, Duy, T. T., & Hanh, T. (2022). Outage performance of interference cancellation-aided two-way relaying cognitive network with primary TAS/SC communication and secondary partial relay selection. Electronics, 11(22), 3645. https://doi.org/10.3390/electronics11223645
- Nguyen, B. C., Tran, M. H., Tran, T. P., & Nguyen, N. T. (2019). Outage probability of NOMA system with wireless power transfer at source and full-duplex relay. AEU – International Journal of Electronics and Communications. 2019. https://doi.org/10.1016/j.aeue.2019.152957
- Nguyen, N.-L., Tu, L.-T., Nguyen, T. N., Nguyen, P.-L. T., & Nguyen, Q.-S. (2023). Performance on cognitive broadcasting networks employing fountain codes and maximal ratio transmission. Radioengineering, 32(1), https://doi.org/10.13164/re.2023.0001
- Nguyen, T. N., Duy, T. T., Tran, P. T., Voznak, M., Li, X., & Poor, H. V. (2022a). Partial and full relay selection algorithms for AF multi-relay full-duplex networks with self-energy recycling in non-identically distributed fading channels. IEEE Transactions on Vehicular Technology, 71(6), 6173–6188. https://doi.org/10.1109/TVT.2022.3158340
- Nguyen, T. N., Quang Minh, T. H., Tran, P. T., Voznak, M., Duy, T. T., T. -L. Nguyen, & Tin, P. T. (2019). Performance enhancement for energy harvesting based two-way relay protocols in wireless ad-hoc networks with partial and full relay selection methods. Ad Hoc Networks, 84, 178–187. https://doi.org/10.1016/j.adhoc.2018.10.005
- Nguyen, T. N., Tran, M., Nguyen, T. -L., Ha, D. -H., & Voznak, M. (2019a). Multisource power splitting energy harvesting relaying network in half-duplex system over block Rayleigh fading channel: System performance analysis. Electronics, 8(1), 67. https://dx.doi.org/10.3390/electronics8010067
- Nguyen, T. N., Tran, M., Nguyen, T. -L., Ha, D. -H., & Voznak, M. (2019b). Performance analysis of a user selection protocol in cooperative networks with power splitting protocol-based energy harvesting over Nakagami-m/Rayleigh channels. Electronics, 8(4), 448. https://doi.org/10.3390/electronics8040448
- Nguyen, T. N., Tran, P. T., & Vozňák, M. (2018). Power splitting-based energy-harvesting protocol for wireless-powered communication networks with a bidirectional relay. International Journal of Communication Systems, 31(13), e3721. https://doi.org/10.1002/dac.3721
- Nguyen, T. N., Tu, L.-T., Tran, D.-H., Phan, V.-D., Voznak, M., Chatzinotas, S., & Ding, Z. (2022). Outage performance of satellite terrestrial full-duplex relaying networks with co-channel interference. IEEE Wireless Communications Letters, 11(7), 1478–1482. https://doi.org/10.1109/LWC.2022.3175734
- Pham, N. S., Tran, T. D., & Ho, V. K. (2020). SIC-Coding schemes for underlay two-way relaying cognitive networks. Wireless Communications and Mobile Computing. Article ID 8860551, 17 pages. https://doi.org/10.1155/2020/8860551
- Phu, T. T., Phan, V. -D., Nguyen, N. T., & Le, A. V. (2021a). Performance analysis in the decode-and-forward full-duplex relaying network with SWIPT. The Scientific World Journal. Article ID 5548802, 8 pages. https://doi.org/10.1155/2021/5548802
- Phu, T. T., Phan, V. -D., Nguyen, N. T., & Le, A. V. (2021b). Performance analysis in the decode-and-forward full-duplex relaying network with SWIPT. The Scientific World Journal. Article ID 5548802, 8 pages. https://doi.org/10.1155/2021/5548802
- Tran, T. D., Pham, T. D. N., & Tran, T. P. (2021). Performance enhancement for multihop cognitive DF and AF relaying protocols under joint impact of interference and hardware noises: NOMA for primary network and best-path selection for secondary network. Wireless Communications and Mobile Computing, 2021, https://doi.org/10.1155/2021/4345622
- Tu, L. -T., Di Renzo, M., & Coon, J. P. (2018). Stochastic geometry analysis of receiver diversity in cellular networks with SWIPT. In 2018 IEEE 19th International Workshop on Signal Processing Advances in Wireless Communications (SPAWC). IEEE. https://doi.org/10.1109/SPAWC.2018.8445877
- Tu, L. -T., Nguyen, T. N., Tran, T. D., Tran, P. T., Voznak, M., & Aravanis, A. I. (2022). Broadcasting in cognitive radio networks: A fountain codes approach. IEEE Transactions on Vehicular Technology, 71(10), 11289–11294. https://doi.org/10.1109/TVT.2022.3188969
- Tu, L. -T., Phan, V. -D., Nguyen, T. N., Tran, P. T., Duy, T. T., Nguyen, Q. -S., Nguyen, N. -T., & Voznak, M. (2023). Performance analysis of multihop full-duplex NOMA systems with imperfect interference cancellation and near-field path-loss. Sensors, 23(1), 524. https://doi.org/10.3390/s23010524
- Van Chien, T., Tu, L. T., Chatzinotas, S., & Ottersten, B. (2021). Coverage probability and ergodic capacity of intelligent reflecting surface-enhanced communication systems. IEEE Communications Letters, 25(1), 69–73. https://doi.org/10.1109/LCOMM.2020.3023759.
- Wang, D., Zhang, R., Cheng, X., & Yang, L. (2017). Capacity-Enhancing full-duplex relay networks based on power-splitting (PS-)SWIPT. IEEE Transactions on Vehicular Technology, 66(6), 5445–5450. https://doi.org/10.1109/TVT.2016.2616147
- Wu, W., Yin, X., Deng, P., Guo, T., & Wang, B. (2019). Transceiver design for downlink SWIPT NOMA systems with cooperative full-duplex relaying. IEEE Access, 7, 33464–33472. https://doi.org/10.1109/Access.6287639
- Yang, H., Xia, X., Li, J., Zhu, P., & You, X. (2022). Joint transceiver design for network-assisted full-duplex systems with SWIPT. IEEE Systems Journal, 16(1), 1206–1216. https://doi.org/10.1109/JSYST.2021.3062455