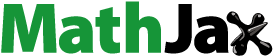
Abstract
Several new amide derivatives having varied aromatic characteristics prepared efficiently via nucleophilic acyl substitution reactions are tested for corrosion inhibition of mild steel in 1 M HCl at 20, 40, and 60 °C range by gravimetric methods. All compounds have shown very good inhibition efficiency (IE%) in acidic solutions. Increasing the temperature (in the presence of the inhibitor in the higher concentration (100–1000 μM) are found to increase the inhibition efficiency of the amide’s derivatives. Results from gravimetric measurements further revealed that Inh. 4 shows excellently at 60 °C giving IE% of 91.88 at 1000 μM. Thermodynamic parameters (ΔGºads, ΔHºads, and ΔS ºads) for the adsorption process and kinetic parameters for the metal dissolution (or hydrogen evolution) reaction in the presence of the new derivatives were determined. Experimental results agree with the Temkin adsorption isotherm. The inhibition of corrosion in 1 M HCl, influenced by both physi- and chemisorption, was found to be under mixed control but predominantly under cathodic control as revealed by the potentiodynamic polarization measurements for Inh. 4. Frontier molecular orbital calculations predicted that the newly synthesized inhibitors with aromatic characteristics serve as donor-centers to the empty d-orbital of the metal atoms and would exhibit higher corrosion IE%.
1. Introduction
Corrosion is a harmful attack on metals caused by contact with the environment. It causes weight loss and inadequate robustness, resulting in major economic and environmental issues. In addition, due to the influences of some aggressive mediators, such as acidic environments, temperature changes, oxygen, mechanical pressure, and moisture, corrosion occurs (Liu, Wang, Gao, & Zhang, Citation2016; Shaban, Elsamad, Tawfik, Abdel-Rahman, & Aiad, Citation2020).
Therefore, protecting the equipment is a crucial step that needs to be arranged ahead of time. HCl is frequently used in a range of applications, such as acid pickling, etching, and descaling processes in a variety of sectors, despite its effect on the metals (Liu, Quan, Zhu, Huang, & Zhou, Citation2020; Kamal, Hussein, Mahmoud, Sultan, & Saad, Citation2018; Tariq, Kamal, Mahmoud, Alade, & Al-Nakhli, Citation2021). As a result, equipment protection is a major problem in terms of cost-cutting and environmental preservation. Based on their chemical structure, organic corrosion inhibitor materials performed admirably against steel corrosion (Ali, Saeed, & El-Sharif, Citation2012; Ali, & El-Sharif, 2012; Ali, Haladu, & El-Sharif, Citation2016a; Ali, Haladu, & El-Sharif, Citation2016b; Ali, Al-Muallem, Rahman, & Saeed, Citation2008a; Ali, Al-Muallem, Saeed, & Rahman, Citation2008b; Shaban, Elbhrawy, et al., Citation2021).
For adsorption within the surfaces of the corroded materials, some functional groups, such as nitrogen, phosphorous, oxygen, double bonds, and sulfur, are considered electronic-rich active centers and hence improve steel protection (Elsharif, Citation2017). To isolate the surface from this forceful medium, the electronically characterized group creates an interaction with the unoccupied d-orbital so that a robust protective coating can be formed (Liu, Zhang, Liu, Li, & Li, Citation2019; Pakiet, Tedim, Kowalczyk, & Brycki, Citation2019). Corrosion inhibitors are interesting in that they have a unique structure that has two opposing polarities: hydrophobic and hydrophilic sections at the same time (Shaban, Aiad, Moustafa, & Aljoboury, Citation2019; Aiad, Shaban, Elged, & Aljoboury, Citation2018; Zhu & Free, Citation2016; Shaban, El-Sherif, & Fahim, Citation2018; Shaban, Aiad, El-Sukkary, Soliman, & El-Awady, Citation2015). Hydrophilic heads are an electronic-rich group with a high propensity for forming interactions through steel surfaces, whereas a protective layer has been formed by the hydrophobic surfactant tail (Heakal & Elkholy, Citation2017; Migahed, Alsabagh, Abdou, Abdel-Rahman, & Aboulrous, Citation2019; Liang et al., Citation2019).
Surfactants are also utilized to prevent corrosion (Dandigunta, Karthick, Chattopadhyay, & Dhoble, Citation2021; Saad, Abdurahman, & Yunus, Citation2021; Lei et al., Citation2020; Tung et al., Citation2021; Elged et al., Citation2021, Elsherif, Elged, & Shaban, Citation2021). The Gemini surfactants are distinguished by two identical portions connected by a spacer, each of which has a hydrophilic and lipophilic moiety (Badr et al., Citation2021, Shaban, Elbhrawy, et al., Citation2021). However, growing environmental consciousness and severe environmental restrictions around the world necessitated the generation of green erosion inhibitors along with producing high inhibitory characteristics while also posing little danger to the environment. Multicomponent reactions, which consist of three or more reactive compounds, must have emerged as a potent and green technology in this context because they can synthesize multiple biologically active complex chemicals in one step in high yields (Beattie, North, & Villuendas, Citation2011). Furthermore, using ecologically friendly solvents and chemicals is critical, as they can participate in numerous green chemistry approaches. Additionally, due to the features of nonhazardous, nonflammable, redox-stable, nontoxic, and readily available water, it has gained much interest in the last few years as a reaction medium. Many of the organic corrosion inhibitors used in carbon steel are hazardous and have to be replaced with new, ecologically friendly inhibitors (Elsharif et al., Citation2020a; Elsharif et al., Citation2020b). New catechol derivatives have recently been synthesized using an environmentally friendly electrochemical technique (Toghan, Abo-Bakr, Rageh, & Abd-Elsabour, Citation2019). Because the heterocyclic unit constitutes most of the chemical and pharmaceutical products, as well as a good corrosion inhibitor, the development of new heterocyclic derivatives is critical. In addition, the consumption of L-proline in water and ionic liquids with respect to asymmetric organocatalyzed reactions can be considered the most environmentally friendly method because it can assist in isolating natural resources without the utilization of dangerous solvents and chemicals such as DMF, DMSO, and other solvents of chlorinate (Sheldon, Citation2005; Nezhad, Sarikhani, Shahidzadeh, & Panahi, Citation2012).
A continuous determination was carried out to develop a corrosion inhibitor that shows better inhibition at low concentrations in corrosive media and is environmentally friendly (Tabatabaei, Ramezanzadeh, Ramezanzadeh, & Bahlakeh, Citation2020; Bahlakeh, Dehghani, Ramezanzadeh, & Ramezanzadeh, Citation2019; Tabatabaei, Asaldous, Bahlakeh, Ramezanzadeh, & Ramezanzadeh, Citation2019a; Ramezanzadeh, Bahlakeh, & Ramezanzadeh, Citation2019; Dehghani, Bahlakeh, & Ramezanzadeh, Citation2019; Tabatabaei, Bahlakeh, Dehghani, Ramezanzadeh, & Ramezanzadeh, Citation2019b).
As prospective inhibitors of minor steel corrosion in an acidic environment, the present work is dedicated to the synthesis of new derivatives from natural fatty acid (E-octadec-9-enoic acid).
To our knowledge, this is the first report aimed at synthesizing these compounds and studying their ability to inhibit corrosion.
2. Experimental
2.1. Materials and methods
E-octadec-9-enoic acid (oleic acid) (≥99.0%, GC), thionyl chloride (≥99.7%), 1-aminoanthraquinone (97%), 1,4-diaminoanthraquinone (≥88%), 1-aminoanthracene (90%), 2-aminoanthracene (96%), hydrochloric acid (HCl) (ACS reagent, 37%), and dimethyl sulfoxide DMSO (≥99%) were procured from Sigma Aldrich. Dialysis was performed using a Spectra/Por Standard Regenerated Cellulose (RC) Membrane that was purchased from Spectrum Laboratories Inc. Dimethyl sulfoxide (DMSO) was dehydrated over calcium hydride for one night before being distilled under reduced pressure at a boiling point of 648–658 °C (4 mm Hg). The high-performance liquid chromatography (HPLC) grade was used for all solvents.
Glass-based equipment was cleaned using deionized (DI) or distilled water. A nitrogen atmosphere was used for each of the processes. To create solutions of HCl, (Fisher Scientific Company), concentrated HCl (A.C.S.) and distilled or DI water was used (1 M). This study included tests using inhibitors with a concentration range of 5–1000 μM.
2.2. Spectral characterization of inhibitors
A Shimadzu IR-Affinity FTIR spectrometer was used to record the infrared spectrum. Moreover, a Bruker spectrometer (400 MHZ capacity) was utilized to record the 1H spectra and 13C NMR spectra. The dissolution of 0.5 ml in a sample was prepared for the experiment along with preparing 0.5 ml of DMSO. Through the utilization of tetramethyl silane (TMS) as the central standard, chemical shifts (δ) are employed in ppm within the entire process. In the same vein, Carlo-Erba elemental evaluation Model 1106 was employed in this research to analyze the elements.
2.3. Corrosion study
2.3.1. Specimens
Mild steel was chosen for this study because it is widely used in oil and gas pipelines. It is inexpensive, suitable for various cutting and coating methods, and has good weldability while providing adequate physical properties. Gravimetric measurements were used to test the corrosion inhibition in HCl (1.00 M) by using mild steel coupons with the following percentage composition: 0.010 (P); 0.005 (Cu); 0.022 (Ni); 0.34 (Mn); 0.089 (C); 99.47 (Fe); 0.005 (V); 0.007 (Mo); and 0.037 (Cr). A test specimen in the form of a flag was produced from a mild steel sheet with a width of 1 mm for the electrochemical measurement for Inh. 4. An insulating paint was used to insulate the flag base, which was around 3 cm in diameter. The exposed area was 2 cm2, with the remaining area being 1 cm2. The mild steel specimen was roughened with emery papers of various grit sizes (100, 400, 600, 800, and 1200), then washed with distilled water and acetone. After drying, the specimen was placed in a desiccator for storage.
2.3.2. Solutions
Concentrated HCl (37%) with deionized water (DI) was used to prepare a solution of 1.00 M hydrochloric acid. The range of inhibitor concentrations was recorded from 5 to 1000 μM in this process. Under 37% HCl, the corrosion rate is high. The higher the HCl concentration, the faster the corrosion. The solubility of the new synthesized derivatives in HCl solutions is quite low at room temperature. To increase the solubility, solutions were stirred at 40 and 60 °C overnight.
2.4. Gravimetric measurements
A mild steel specimen, with dimensions of 2.0 × 2.0 × 0.5 cm, was subjected to the gravimetric corrosion measurements. Freshly polished specimens were tested for 96 h in a 250 ml solution of 1.00 M HCl at 20, 40, and 60 °C in both the absence and presence of inhibitors at concentrations of 5–1000 μM. An increase in temperature will increase the rate of corrosion of metal, while a decrease in temperature will lead to a decrease in the rate of corrosion of a metal. At high temperatures, oxidation reactions occur faster and as a result, the corrosion rate increases. The specimens were then cleaned to eliminate any corrosion products that had been adsorbed. Deionized water was first used for cleaning, then acetone, and finally drying in a vacuum oven at room temperature. EquationEquation (1)(1)
(1) was used to calculate corrosion rates.
(1)
(1)
where A is the specimen area (cm2), W is the weight loss (g), D is the mild steel density (g/cm3), and t is the exposure duration (h). Accordingly, the specimen weight loss in the presence and absence of inhibitor molecules (blank) was calculated. To achieve the predicted standard deviation SD, the operation was carried out three times.
EquationEquations (2)(2)
(2) and Equation(3)
(3)
(3) were used to calculate the inhibitor surface coverage (θ) and the corrosion inhibition efficiency (η%), respectively.
(2)
(2)
(3)
(3)
In both equations, CRi and CRo are the values of corrosion rates in the presence and absence of inhibitors, respectively.
2.5. Electrochemical measurements
The electrochemical performance of Inh.4 was studied at room temperature using electrochemical techniques (Tabatabaei et al., Citation2019b). A Gamry Reference 600 potentiostat equipped with Echem Analyst 6.33 suite for data interpretation was used for the measurements. The experiments were conducted using a standard three-electrode assembly wherein the RCE acted as the working electrode, a saturated calomel electrode (SCE) as a reference electrode, and a graphite rod as the counter electrode. Before conducting the electrochemical tests, the potential of the working electrode was recorded in the open circuit condition to achieve a stable value, EOCP, upon which the electrochemical measurements were performed. Potentiodynamic polarization (PDP) was undertaken in the range ±250 mV versus EOCP with a scan rate of 0.2 mVs−1. All the potential values reported in the present work are in reference to the SCE.
2.6. Computational procedure
The gap between the energy differences of the LUMO and HOMO is examined in Frontier molecular orbital (FMO) studies, which offer fundamental knowledge about electrons. In contrast to LUMO, which consists of an electrophile that accepts electrons from a nucleophile, HOMO is a type of nucleophile that represents electrons. The stability of the molecule can also be similarly determined by the FMO. Molecules have a lower energy gap, are softer, and are more chemically reactive (Hiremath et al., Citation2018; Frisch, Trucks, & Schlegel, Citation2009).
Similar to smaller energy molecules, larger energy molecules may exhibit both great stability and strong chemical hardness. The HOMO and LUMO energies can be used to determine the global reaction factors, where the ionization reaction potential (IP), electronegativity (χ), electron affinity (EA), molecular softness (S), global hardness (ղ), chemical reaction potential (), and electrophilicity index (ω) are all shown and summarized in Table S1. To calculate the fraction of transferred electrons, theoretical values of 7.0 eV and 0 were assigned to χFe and ηFe, respectively.
Table 1. Carbon steel corrosion rate, surface coverage, and % inhibition efficiency in various concentrations of Inh. 1 at various temperatures.
With the support of the LANL2DZ/6-311 g basis set as well as the Gaussian 09 W set (Hiremath et al., Citation2018), FMO theory has helped in the quantum chemical geometry optimization technique of isolated molecules. When picking the implicit solvent model, the optimal estimation approach for solvation energies may be provided, which is why set LANL2DZ/6-3111 g was chosen (Abdulazeez et al., Citation2019). Furthermore, it provides accurate data experiment correlation results at a low cost. In addition, on the potential energy surfaces regarding the minima, vibrational frequency calculations and geometry optimizations were calculated. Water was used as the fundamental factor, and Tomasi’s separated as a consistent reaction field was used as the model (Tomasi, Menucci, & Cammi, Citation2005). The constant sequences of interlocking spheres are positioned in the cavity where the solute is in this model, and the continuum of uniform dielectric constants is placed in this solvent (Al-Saadi, Citation2020). Similar calculations were conducted to evaluate the inhibitor’s reactions in an acid standard, taking into consideration the effects of protonation and protonated versions of the molecules. The vitalities of the maximum molecular highest orbital (EHOMO) and minimum molecular lowest orbital (ELUMO) were also determined as quantum reactivity descriptors (Pearson, Citation1988).
(4)
(4)
(5)
(5)
(6)
(6)
The ionization energy, global hardness, and electron affinity are represented by I, η and A, respectively, in the preceding equation. The electrophilicity index (ω) and electronegativity (χ) are two additional parameters that signify the ability of inhibitors to attract electrons if they are chemically combined with other compounds (Khalil, Citation2003; Ashassi-Sorkhabi, Shaabani, & Seifzadeh, Citation2005; El-Ashry, El Nemr, & Ragab, Citation2012; Migahed et al., Citation2017).
(7)
(7)
(8)
(8)
Electrons must flow from less electronegativity inhibitors to higher electronegativity surface metals in inhibitor–metal interactions until the chemical potentials are equal. The following equation is used to calculate the fraction electron transfer.
(9)
(9)
The terms χinh, ηFe, χFe and, ηinh describe the absolute hardness of the substance iron, the inhibitory characteristics of molecules, and the electronegativities, respectively. In the preceding equation, the rates of χFe and ηFe were labeled 7.0 eV and 0 eV, respectively. All relative calculations were performed using Gaussian featured 09 W, and the graphical interface of Gauss View 5.0 was used to extract, visualize, and evaluate the data (Khalil, Citation2003; Ashassi-Sorkhabi et al., Citation2005; El-Ashry et al., Citation2012; Migahed et al., Citation2017).
2.7. Surface characterization
A field emission scanning electron microscope was used to examine the surface of mild steel coupons that had been submerged in 1.00 M HCl for 24 h in both the absence and presence of inhibitor molecules (FESEM). Also, 50 ml of test solutions with and without inhibitors were submerged into coupons of 1.0 × 1.0 × 0.2 cm in size. After 24 h, the coupons were taken out, rinsed multiple times in ethanol and water to eliminate any corrosion products that had been adsorbed, and then dried in a vacuum oven.
2.8. Synthesis of inhibitors
2.8.1. Part 1: Synthesis of E-octadec-9-enoyl chloride (Inh. 1)
Add 5 ml of DMF in dry benzene and (1.0 ml, 0.0035 mol) solution of E-octadec-9-enoic acid into a single-neck round bottom flask. A condenser was included with the flask. SOCl2 (0.5 ml) was gradually added to the mixture using a dropping funnel because the reaction is endothermic. The mixture was refluxed for an hour at 90 °C after stirring for ten minutes. The entire solvent was extracted from the mixture using a rotary for 10 min. E-octadec-9-enoyl chloride (Inh. 1) produced in this step was used in the second part step reaction.
Yield (94%), as white powder. IR (KBr): v = 3010, 2954, 2923, 2873, 1668, 1631, 1620, 1445, 1370, and 1295 cm−1.
1H NMR (DMSO d6, 400 MHz) δ: 0.88 (t, 3H, –CH3, J = 8.4 Hz), 1.29–1.31 (m, 20H, –CH2–, J = 8.4 Hz), 1.58 (m, 2H, –CH2–CH2–C = O, J = 8.4 Hz), 2.18 (q, 4H, –CH2–CH=, J = 8.4 Hz), 2.84 (t, 2H, –CH2, J = 8.4 Hz), 5.48 (t, 4H, –CH=CH–, J = 8.4 Hz).
13C NMR (DMSO d6, 100 MHz) δ: 14.10 (1C, –CH3), 22.70 (1C, –CH2–CH3), 25.00 (1C, –CH2–), 28.20 (1C, –CH2–), 29.30, 29.40 (2C, –CH2–CH2–), 29.71 (3C, –CH2–CH2–), 29.90 (2C, –CH2–CH2–), 31.90 (1C, –CH2–), 33.71 (2C, –CH2–CH = CH–), 46.70 (1C, –CH2–CO–), 130.60 (2C, –CH=CH–), 172.60 (1C, –C = O).
C18H33ClO (300.91): Calculated %, C, 71.85; H, 11.05; Cl, 11.78; O, 5.32. Found: C, 72.43; H, 10.25; Cl, 11.90; O, 5.30.
2.8.2. Part 2: Preparation of E-octadec-9-enoic amides derivatives (Inhs. 2–5)
A numerous of aromatic amine, including 1-aminoanthraquinone (0.78 gm, 0.0035 mol), 1,4-diaminoanthraquinone (0.833 gm, 0.0035 mol), 1-aminoanthracene (0.77 gm, 0.0035 mol), and 2-aminoanthracene (0.77 gm, 0.0035 mol) separately and (30 ml) dry benzene was added in a round bottom flask. The flask was coupled with a guard tube. The material was poured via a dropping funnel and then blended for 45 min. The mixture was stirred for 12 h at reflux temperature until it reached room temperature (TLC, n-hexane: ethyl acetate, 7:3, v: v).
The mixtures were first washed at (40–60)°C and then at (80–100)°C with petroleum ether. E-octadec-9-enoic amides derivatives (Inhs. 2-5) were obtained after all solvents were eliminated and evaporated using a rotary evaporator.
2.8.2.1. Synthesis of N-(9,10-dioxo-9,10-dihydroanthracen-1-yl)oleamide (Inh. 2)
Yield (90%), as red crystals. IR (KBr): v = 3300, 3010, 2916, 2843, 1668, 1631, 1590, 1445, 1375, and 1266 cm−1.
1H NMR (DMSO d6, 400 MHz) δ: 0.88 (t, 3H, –CH3, J = 8.4 Hz), 1.29–1.33 (m, 20H, –CH2–, J = 8.4 Hz), 1.63 (m, 2H, –CH2–CH2–C = O, J = 8.4 Hz), 2.18 (q, 4H, –CH2–CH=, J = 8.4 Hz), 2.39 (t, 2H, –CH2, J = 8.4 Hz), 5.40 (t, 4H, –CH=CH–, J = 8.4 Hz), 7.62, 7.72, 7.88, 8.29, 8.48 (m, 7H, Ar–H, J = 8.4 Hz), 7.23 (br, 1H, –NH).
13C NMR (DMSO d6, 100 MHz) δ: 14.21(1C, –CH3), 22.71 (1C, –CH2–CH3), 25.60 (1C, –CH2–), 27.71 (2 C, –CH2–CH = CH–), 28.60 (1C, –CH2–), 29.00, 29.31 (2C, –CH2–CH2–), 29.70 (3C, –CH2–CH2–), 29.93 (2C, –CH2–CH2–), 31.90 (1C, –CH2–), 38.30 (1C, –CH2– CO–), 117.90 (1C, Ar–C), 122.40 (1C, Ar–C), 125.90 (1C, Ar–C), 126.80 (2C, Ar–C), 130.63 (2C, –CH=CH–), 132.10 (2C, Ar–C), 133.60, 133.80 (3C, Ar–C), 136.60 (1C, Ar–C), 141.90 (1C, Ar–C), 172.40 (1C, –C = O), 182.10, 185.70 (2C, –C = O).
C33H45NO3 (503.33): Calculated %, C, 78.69; H, 9.00; N, 2.78; O, 9.53. Found: C, 78.69; H, 8.99; N, 2.77; O, 9.55.
2.8.2.2. Synthesis of N-(4-amino-9,10-dioxo-9,10-dihydroanthracen-1-yl)oleamide (Inh. 3)
Yield (89%), as purple crystals. IR (KBr): v = 3027, 3022, 3013, 2952, 2842, 1670, 1631, 1589, 1575, 1443, 1371, 1274, and 1210 cm−1.
1H NMR (DMSO d6, 400 MHz) δ: 0.88 (t, 3H, –CH3, J = 8.4 Hz), 1.29–1.33 (m, 20H, –CH2–, J = 8.4 Hz), 1.63 (m, 2H, –CH2–CH2–C = O, J = 8.4 Hz), 2.20 (q, 4H, –CH2–CH=, J = 8.4 Hz), 2.41 (t, 2H, –CH2, J = 8.4 Hz), 5.42 (t, 4H, –CH=CH–, J = 8.4 Hz), 6.90, 7.85, 7.88, 8.29 (m, 6H, Ar–H, J = 8.4 Hz), 6.27,8.60 (br, 2H, –NH).
13C NMR (DMSO d6, 100 MHz) δ: 14.11 (1C, –CH3), 22.69 (1C, –CH2–CH3), 25.61 (1C, –CH2–), 27.70 (2C, –CH2–CH = CH–), 28.61 (1C, –CH2–), 29.13, 29.33 (2C, –CH2–CH2–), 29.72 (3C, –CH2–CH2–), 29.90 (2C, –CH2–CH2–), 31.93 (1C, –CH2–), 38.34 (1C, –CH2– CO–), 112.80 (1C, Ar–C), 118.70 (1C, Ar–C), 119.90 (1C, Ar–C), 125.80 (1 C, Ar–C), 126.80 (2C, Ar–C), 131.12 (2C, –CH=CH–), 131.90 (1C, Ar–C), 132.10 (2C, Ar–C), 133.60 (2 C, Ar–C), 146.50 (1C, Ar–C), 171.52 (1C, –C = O), 185.70 (2C, –C = O). C33H46N2O3 (518.35): Calculated %, C, 76.41; H, 8.94; N, 5.40; O, 9.25. Found: C, 76.40; H, 8.90; N, 5.41; O, 9.30.
2.8.2.3. Synthesis of N-(anthracen-1-yl)oleamide (Inh. 4)
Yield (82%), as brown crystals. IR (KBr): v = 3287, 3010, 2954, 2916, 2860, 2845, 1626, 1590, 1445, 1422, 1370 and 1292 cm−1.
1H NMR (DMSO d6, 400 MHz) δ: 0.88 (t, 3H, –CH3, J = 8.4 Hz), 1.29–1.33 (m, 20H, –CH2–, J = 8.4 Hz), 1.60 (m, 2H, –CH2–CH2–C = O, J = 8.4 Hz), 2.18 (q, 4H, –CH2–CH=, J = 8.4 Hz), 2.39 (t, 2H, –CH2, J = 8.4 Hz), 5.41 (t, 4H, –CH=CH–, J = 8.4 Hz), 6.62, 7.22, 7.39, 7.40, 7.91, 8.30 (m, 9H, Ar–H, J = 8.4 Hz), 7.23 (br, 1H, –NH).
13C NMR (DMSO d6, 100 MHz) δ: 14.11 (1C, –CH3), 22.70 (1C, –CH2–CH3), 25.60 (1C, –CH2–), 27.73 (2C, –CH2–CH = CH–), 28.60 (1C, –CH2–), 29.00, 29.30 (2C, –CH2–CH2–), 29.71 (3C, –CH2–CH2–), 29.91 (2C, –CH2–CH2–), 31.88 (1C, –CH2–), 38.31 (1C, –CH2– CO–), 108.80 (1C, Ar–C), 118.80 (1C, Ar–C), 119.20 (1C, Ar–C), 121.20 (1C, Ar–C), 125.6, 125.90 (3C, Ar–C), 126.80 (1C, Ar–C), 128.10 (2C, Ar–C), 130.06 (2C, –CH=CH–),130.40 (1C, Ar–C), 131.40 (1C, Ar–C), 132.30 (1C, Ar–C), 142.10 (1C, Ar–C). C33H47NO (473.37) Calculated %: C, 83.67; H, 10.00; N, 2.96; O, 3.38. Found %: C, 83.66; H, 9.99; N, 2.94; O, 3.39.
2.8.2.4. Synthesis N-(anthracen-2-yl)oleamide (Inh. 5)
Yield (88%), as dark gray crystals. IR (KBr): v = 3270, 3012, 2952, 2916, 2862, 2843, 1631, 1575, 1470, 1435, 1375, and 1295 cm−1.
1H NMR (DMSO d6, 400 MHz) δ: 0.88 (t, 3H, –CH3, J = 8.4 Hz), 1.29–1.33 (m, 20H, –CH2–, J = 8.4 Hz), 1.63 (m, 2H, –CH2–CH2–C = O, J = 8.4 Hz), 2.18 (q, 4H, –CH2–CH=, J = 8.4 Hz), 2.39 (t, 2H, –CH2, J = 8.4 Hz), 5.42 (t, 4H, –CH=CH–, J = 8.4 Hz), 6.83, 7.03, 7.39, 7.75, 7.91, 8.08, 8.19 (m, 9H, Ar–H, J = 8.4 Hz), 7.22 (br, 1H, –NH).
13C NMR (DMSO d6, 100 MHz) δ: 14.11 (1C, –CH3), 22.70 (1C, –CH2–CH3), 25.61 (1C, –CH2–), 27.70 (2C, –CH2–CH = CH–), 28.61 (1C, –CH2–), 29.11, 29.31 (2C, –CH2–CH2–), 29.70 (3C, –CH2–CH2–), 29.90 (2C, –CH2–CH2–), 31.90 (1C, –CH2–), 38.50 (1C, –CH2– CO–), 107.50 (1C, Ar–C), 116.40 (1C, Ar–C), 123.20 (1C, Ar–C), 124.70, 124.9 (2C, Ar–C), 125.60 (2 C, Ar–C), 126.91 (1C, Ar–C), 127.90, 128.10 (3C, Ar–C), 130.60 (2C, –CH=CH–),130.80 (1C, Ar–C), 131.61 (1C, Ar–C), 142.32 (1C, Ar–C).
C33H47NO (473.37) Calculated %: C, 83.67; H, 10.00; N, 2.96; O, 3.38. Found %: C, 83.67; H, 9.98; N, 2.99; O, 3.37.
3. Results and discussion
3.1. Characterization of the synthesized derivatives
As shown in Schemes 1 and 2, the acid chloride and the new amide derivatives Inh. 1, Inh. 2, Inh. 3, Inh. 4, and Inh. 5 were synthesized in excellent yields from inexpensive materials namely E-octadec-9-enoic acid, 1-aminoanthraquinone, 1,4-diaminoanthraquinone, 1-aminoanthracene, 2-aminoanthracene, and thionyl chloride, respectively.
Scheme 2. Schematic diagram of the synthesis of N-(9,10-dioxo-9,10-dihydroanthracen-1-yl)oleamide (Inh. 2); N-(4-amino-9,10-dioxo-9,10-dihydroanthracen-1-yl)oleamide (Inh. 3); N-(anthracen-1 yl)oleamide (Inh. 4); and N-(anthracen-2-yl)oleamide (Inh. 5).

The newly synthesized compounds’ FTIR spectra revealed bands in the regions at (2915–2955) cm−1 and (2842–2873) cm−1 for C–H stretching as well as bands at (3010–3022) cm−1 and (1575–1630) cm−1 for = C–H, C = C aromatic, and alkene stretching, respectively.
Furthermore, new amide bands appeared in the regions of 1590–1700 cm−1 and 3140–3300 cm−1, followed by –C = O and N–H, respectively. In addition, in this region, O–H stretching, and N–H is not presented in Inh. 1. Moreover, due to the formation of carbonyl groups with Inh. 1, a new and fresh band appeared at 1668 cm−1.
The 1H NMR spectra for the amide derivatives Inh. 2, Inh. 3, Inh. 4, and Inh. 5 also revealed significant signals for (–CH3) at δ 0.88; (–CH2–CONH–) at δ 2.18–2.20; (–CH2–CH2–CH2–) at δ 1.29–1.33; (=CH–CH2CH2–) at δ 1.58–1.63; (=CH–CH2–CH=) and (CH2CH2CONH–) at δ 2.39–2.84; (–CH = CH–) at δ 5.40–5.84; (br, –NH–) at δ 7.20–8.6 for Inh. 2, Inh. 3, Inh. 4, and Inh. 5. The composition of aromatic protons (=CH– Ar) at δ 6.75–7.53 were presented by the new Inh. 2, Inh. 3, Inh. 4, and Inh. 5. Additionally, at 2.71–2.84 for (–CH2–CH2–NH–) and (–NH–CH2–), Inh. 2, Inh. 3, Inh. 4, and Inh. 5 showed numerous signals.
Similarly, major carbon signals have been shown through Inh. 1, Inh. 2, Inh. 3, Inh. 4, and Inh. 5 in 13C NMR spectra at δ 14.38–14.41 to (–CH3); δ 22.52–22.70 to (CH2–CH3); δ 34.12–28.60 to (–CH2–CH2CO–); δ 27.70–34.11 to (–CH2–CH=); δ 32.10–38.30 to (–CH2–CO–NH); δ 139.69–181.52 (1 C, –CONH); δ 105.55–129.78 to (–CH=CH–). New signals appeared at δ 25.79, 31.90, and 40.36 to (1 C, –NH–CH) for Inh. 2, Inh. 3, Inh. 4, and Inh. 5, respectively.
3.2. Surface characterization
The results of the surface characterization of coupons immersed in 1.00 M HCl for 24 hours with and without 1000 μM concentrations of the inhibitor molecules Inh. 2, Inh. 2, Inh. 3, Inh. 4, and Inh. 5 are shown in . The microscopic examination of a metal surface after immersion in the corrosion medium without and with a corrosion inhibitor allows a visual interpretation of the adsorption and inhibition phenomena at the metal–solution interface in corrosion inhibition studies. The findings showed that, in contrast to the freshly polished coupon, the coupon submerged in 1.00 M HCl without inhibitor molecules () underwent a severe corrosion attack that damaged the microstructure. However, as shown in , these attacks were significantly reduced for the coupons submerged in test media containing 1000 μM of inhibitor molecules. There is a noticeably smoother surface. A scale-like morphology covers the steel surface in the presence of the new derivatives (), suppressing the occurrence of surface defects such as holes and cracks. This promotes the adsorption of the corrosion inhibitor on the metal surface and the formation of a protective film, which provides isolation from the electrolyte’s aggressive attack.
Figure 1. SEM micrographs of (a) specimen immersed in 1.00 M HCl in the absence of inhibitors, (b) specimen immersed in 1.00 M HCl containing 1000 μM of Inh. 5, (c) specimen immersed in 1.00 M HCl containing 1000 μM of Inh. 3, (d) specimen immersed in 1.00 M HCl containing 1000 μM of Inh. 4 at magnification 20 μm at scanning speed 20 mm/s.

3.3. Weight loss study
Weight loss technique was used to assess the protection effectiveness () and corresponding surface coverage (θ) induced by the prepared non-ionic surfactant-inhibitors (EquationEquations (10)
(10)
(10) and Equation(11)
(11)
(11) ) (Mishrif, Noor El-Din, & Khamis, Citation2018).
(10)
(10)
(11)
(11)
where, for an immersion time of 24 h, t = CR and CRo represent the corrosion rate of steel in 1.0 M HCl medium with varying concentrations of the synthetic inhibitors and that without, respectively. The CR was determined using EquationEquation (11)
(11)
(11) , where W and S stand for exposed steel in mg and cm2, respectively.
shows how steel corrosion is affected by different concentrations of the synthetic inhibitors (Inh. 1, 2, 3, 4, and 5) at temperatures of 20, 40, and 60 °C. As seen in , the weight loss of the mild steel specimen decreases as the inhibitor is added incrementally.
Figure 2. Variation of inhibition efficiency and corrosion rate versus logarithm C of the synthesized inhibitors at 20, 40 and 60 °C.

Table 2. Carbon steel corrosion rate, surface coverage, and % inhibition efficiency in various concentrations of Inh. 2 at various temperatures.
Table 3. Carbon steel corrosion rate, surface coverage, and % inhibition efficiency in various concentrations of Inh. 3 at various temperatures.
Table 4. Carbon steel corrosion rate, surface coverage, and % inhibition efficiency in various concentrations of Inh. 4 at various temperatures.
Table 5. Carbon steel corrosion rate, surface coverage, and % inhibition efficiency in various concentrations of Inh. 5 at various temperatures.
The CR is decreased as a result of synthetic chemical adsorption on the steel surface, which also slows down weight loss. The rate of adsorption increases as inhibitor concentration increases. A barrier that protects the steel surface from the hostile solution is formed by adsorption on the synthetic inhibitor surface. Because the direct function can be created within the inhibitor concentration, steel protection is improved by increasing θ.
The results of the weight loss ( and ) show that the carbon tail of the synthetic inhibitor significantly improves steel protection. As previously explained (Elged et al., Citation2021; Fouda, Elmorsi, Shaban, Fayed, & Azazy, Citation2018), the inhibitor tail increases the adsorption affinity and migration rate of the surfactant inhibitors onto surfaces, increasing the surface coverage and inhibiting effectiveness (Abd-Elaal, Elbasiony, Shaban, & Zaki, Citation2018; Mobin & Aslam, Citation2018; Aiad, Shaban, Elged, et al., Citation2018).
The performance of the examined inhibitors is significantly influenced by temperature. As shown in and , raising the temperature of the corrosive solution containing the created inhibitors to 40 °C caused an increase in the steel’s corresponding The inhibition efficiency decreases as the solution temperature is raised to 60 °C. The findings show that increasing the temperature of the solution containing the tested inhibitors causes the
to increase. The tendency of
to rise with temperature is an indication of the synthetic inhibitors’ propensity to conduct both physical and chemical adsorption on steel surfaces. The investigated inhibitors’ chemical structures changed as a result of the rising temperature of the solution (Zhu, & Free, 2016). This promoted an increase in the electron densities of the active centers, which in turn enhanced the adsorption on the corroded surface.
Based on EquationEquation (12)(12)
(12) , an Arrhenius-type plot was created to clarify the corrosion kinetics and determine the apparent activation energy (Ea).
(12)
(12)
where k is the rate of corrosion in each concentration case, A is the Arrhenius constant, T is the temperature in Kelvin, and R is the gas constant. The prepared inhibitors perform well and follow the Arrhenius plot as shown in with a high regression coefficient. Based on the slope of the Arrhenius line, which equals (−Ea/R), the Ea was calculated. According to the physical adsorption of the synthetic inhibitors on the carbon steel surface, the Ea () of the inhibited solution is higher than that of the uninhibited (Blank solution) (Aiad, Shaban, El-Sukkary, El-Awady, & Soliman, Citation2017).
Figure 3. Arrhenius plots for carbon steel dissolution in absence and presence of different concentrations from the synthesized inhibitors.

Table 6. Values of carbon steel activation characteristics in 1.0 M HCl.
From transition state theory, the activation entropy () and enthalpy (
) were extracted (EquationEquation (13)
(13)
(13) ) (Prabhu, Venkatesha, Shanbhag, Kulkarni, & Kalkhambkar, Citation2008).
(13)
(13)
Avogadro’s number, the rate at which metals dissolve, and the Planck constant are denoted by NA, k, and h, respectively. The transition state EquationEquation (13)(13)
(13) was depicted in with the intercept of
and the slope of
which were used to calculate the corresponding
and
respectively (). The endothermic nature of the corrosion process is reflected by the positive values of
that were extracted (Shaban et al., Citation2020).
Figure 4. Kinetic plot Ln k/T for carbon steel dissolution in absence and presence of different concentrations from the synthesized inhibitors.

The extracted values in the presence and absence of the inhibitors () are negative, indicating that the activated complex (rate-determining step) is an association rather than a dissociation, that is, that there is more order being carried out as it passes from reactant to activate complex (Mishrif et al., Citation2018; Fawzy, Abdallah, Zaafarany, Ahmed, & Althagafi, Citation2018; Shamsheera, Prasad, Jaseela, & Joseph, Citation2021).
Several adsorption modules, including Langmuir, El-Awady, and Temkin isotherm, have been conducted based on the θ results from the gravimetric experiment to clarify the expected adsorption mechanism of the synthesized inhibitors on the mild steel. According to and , the Langmuir isotherm (EquationEquation (14)(14)
(14) ) with a high correlation coefficient (R2) ∼ 0.999 is the best fit adsorption module that describes the adsorption of the investigated inhibitors.
(14)
(14)
Table 7. Thermodynamic values derived from the Langmuir adsorption isotherm on carbon steel in 1.0 M HCl.
The Langmuir EquationEquation (14)(14)
(14) is plotted, and the result is a straight line with a high R2 value of 0.999, indicating that the synthesized inhibitors follow the Langmuir isotherm. It was possible to determine the Kads (adsorption equilibrium constant) from the intercept values of the Langmuir curve (). All synthetic inhibitors have a strong affinity for the surfaces of steel, as shown by the extracted Kads values.
EquationEquation (15)(15)
(15) of the Gibbs equation was used to determine the adsorption free energy change (
) in relation to the synthesized inhibitors (Badr, Bedair, & Shaban, Citation2018; Feng et al., Citation2018).
(15)
(15)
EquationEquation (16)(16)
(16) 's slope yields the adsorption heat change (
), where R is the gas constant and T is the temperature in Kelvin (Flores et al., Citation2011):
(16)
(16)
EquationEquation (17)(17)
(17) was used to calculate the standard adsorption entropy (
) (Badr, Hefni, Shafek, & Shaban, Citation2020; Gao et al., Citation2019):
(17)
(17)
The extracted
and
thermodynamic parameters for the inhibitors are shown in . The calculated
values range from −28 to −32.14, reflecting the synthetic inhibitors’ physical and chemical adsorption nature onto the steel surface (Aslam et al., Citation2021). As a result of the synthetic inhibitor’s adsorption on the surface of mild steel, the positive sign of the
values () indicates greater disorder (Shaban, Aiad, Moustafa, et al., Citation2019). Comparing the inhibitive performance of the studied molecules with those of other reported molecules with similar molecular structures (Table S2) revealed that the molecules performed satisfactorily and could serve as inhibitors of mild steel corrosion in acidic medium.
3.4. Polarization studies
The influence of the variation of Inh. 4 concentration was studied using the PDP measurements (McCafferty, Citation2005; McCafferty, Citation2010). The electrochemical data, that is, the corrosion current densities (icorr), corrosion potential (Ecorr), anodic/cathodic slopes (βa/βc), and the corrosion inhibition efficiencies (), were calculated by extrapolation of the linear segments of the anodic and cathodic Tafel slope and given in . The inhibition efficiency was obtained by the following equation:
(10)
(10)
where,
and
denote the corrosion current densities without and containing the corrosion inhibitor, respectively. The results of the PDP measurements are given in , the addition of the inhibitor to the corrosive solution causes a significant decrease in the anodic and the cathodic currents. A variation in the inhibitor Inh. 4 concentrations from 5 to 500 ppm produces around a tenfold decrease in the corrosion current densities from 57.4 µA/cm2 to 5.63 µA/cm2. This corresponds to an inhibition efficiency of 90.19%. The cathodic predominance revealed in the PDP studies for Inh. 4 shows that the inhibitor mainly adsorbs on the cathodic sites of the steel surface. This suggests that under the influence of the acidic electrolyte, the inhibitor exhibits a tendency to undergo protonation. The protonated form of the inhibitor molecule can undergo electrostatic interaction with the pre-adsorbed Cl− ions and can also adsorb directly at the cathodic sites of the metallic surface. On the other hand, the neutral inhibitor molecule can interact with the metallic surface via sharing the π electrons or the lone pair electrons. The filled d-orbitals of the metal surface can also donate the electrons to the inhibitor molecule via back-donation.
Figure 6. Potentiodynamic polarization plots acquired during the corrosion of mild steel in 1.0 M HCl solution without and with different Inh. 4 concentrations at room temperature.

Table 8. Electrochemical polarization parameters obtained at room temperature for the adsorption of Inh. 4.
3.5. Computational results
FMO studies reveal the energy difference between the ELUMO and EHOMO states. The nucleophile HOMO contributes electrons, while the electrophile LUMO takes electrons from the nucleophile. FMO can be used to determine a molecule’s stability. The chemical reactivity has to be found better if there will be a smaller energy gap between the molecules along with making it softer. Also, if there is more energy gap between the molecules, it will have more stability characteristics and hard chemical reactions followed by making them hard.
When using HOMO and LUMO energies to determine these molecules, the global reactivity descriptors can be presented as ionization potential (Ip), hardness (η), electron affinity (EA), electrophilicity index (ω), softness (S), electronegativity (χ), and chemical potential () as shown in (non-protonated molecules) and Table S3 (protonated molecules),
Table 9. Quantum chemical reactivity descriptors of non-protonated molecules at B3LYP/6-31g(d).
By utilizing the related modules of the energy gap (ΔE = ELUMO − EHOMO) for molecules with the application of EHOMO and ELUMO, the calculated values are −0.24860 eV, −0.05210 eV, and 0.1965 eV, respectively. According to the theorem of Koopmans, the negative energies of LUMO and HOMO can be associated with electron affinity (EA) and ionization potential (Ip).
Additionally, () was used to define the chemical potential, so V = − (Ip+EA)/2 = −0.15035 eV and (χ) was defined to show electronegativity as
= − (Ip+ EA)/2 = −0.15035 eV. In the same regard, both values are calculated from the estimated values of EA and Ip. Similarly, the value of chemical hardness (η) = (Ip−EA)/2 = 0.09825 eV, and the estimated value of chemical softness (S) is shown by S = 10.15022 eV, which can be considered the inverse value of chemical hardness (η). The electrophilicity index can be referred to as the reduction in the energy level due to the additional flow of electrons between the acceptor, which is LUMO, and the donor, which is HOMO. Molecule 1 (Inh. 1) contains the value of the electrophilicity index, which is estimated as ω =
/2 η = 0.115038 eV as shown in .
Figure 7. Optimized geometries and frontier orbital distributions of molecules (Inh. 1) to (Inh. 3) at B3LYP/6-31g(d).

In molecule 2 (Inh. 2), the calculations of related energy differences (ΔE = ELUMO−EHOMO) were calculated with the support of EHOMO and ELUMO, in which the values of −0.20115 eV, −0.07396 eV, and 0.12719 eV were estimated.
A value of −0.20115 eV was calculated for the EHOMO, a value of 0.07396 eV was identified for the ELUMO, and the related energy difference (ΔE = ELUMO−EHOMO) was realized as 0.12719 eV for Inh. 2. The opposite energies between HOMO and LOMO have been depicted by ionization potential (Ip) and electron affinity (EA), respectively.
The value of electronegativity V= − (Ip+EA)/2 = −0.137555 eV represents a negative chemical potential (), and the value of (Ip+EA)/2 = 0.137555 eV is computed for electronegativity (χ). The value of S = 15.7245066 eV is computed for chemical hardness (η), and the value of (Ip − EA)/2 = 0.063595 eV is calculated for chemical softness (S).
The additional movement of electrons between the HOMO, which is the donor, and the LUMO, which is the acceptor, causes the electrophilicity index to ω = /2 η = 0.1487647 eV, which is the electrophilicity index of molecule 2. shows the energy flow diagrams.
Moreover, the value of −0.20911 eV is computed for EHOMO, the value of −0.07908 eV is calculated for ELUMO and the value of the related difference between the energy gap (ΔE = ELUMO−EHOMO) is signified for 0.1003 eV, respectively. The adverse energies between HOMO and LOMO have been depicted by ionization potential (Ip) and electron affinity (EA) accordingly. Likewise, the value of = − (Ip+EA)/2 = −0.144095 eV is computed for chemical potential (V), and the value of
= − (Ip+EA)/2 = −0.144095 eV. for electronegativity (χ), respectively. These values are estimated from Ip and EA. The value of (Ip − EA)/2 = 0.065015 eV is calculated for chemical hardness (η), and S = 15.38106 eV is computed for chemical softness (S). The excessive electron flow between the HOMO, which is the donor, and the LUMO, which is the acceptor, causes a reduction in energy, which is referred to as the electrophilicity index (ω). Molecule 3 (Inh.3) has an electrophilicity index of ω =
/2 η = 0.15968 eV. depicts the energy diagrams.
The value of −0.24539 eV is computed for EHOMO, the value of −0.12560 eV is calculated for ELUMO, and the consistent energy difference (ΔE = ELUMO−EHOMO) is calculated as 0.11979 eV for molecule 4 (Inh. 4). In addition, electron affinity (EA) and ionization potential (Ip) are considered opposite energies between HOMO and LUMO.
Furthermore, the value of = − (Ip+EA)/2= −0.185495 eV was calculated for the chemical potential (
), and the value of
= −(Ip + EA)/2 = −0.185495 eV was estimated for electronegativity (χ).
Moreover, the Ip and EA assisted in calculating both values. The values of Ip−EA/2 = 0.059895 eV are calculated for chemical hardness (η), and the value of 16.69588 eV is computed for chemical softness (S). The excessive flow of electrons among the HOMO, which is the donor, and the LUMO, which is the acceptor, causes a reduction in energy, which is referred to as the electrophilicity index. Molecule 4 (Inh. 4) has assisted in calculating the value of ω = /2 η = 0.28723 eV for an electrophilicity index.
The energy gap associated (ΔE= LUMO − HOMO) and EHOMO, ELUMO, of molecule 5 (Inh. 5) were calculated with values of 0.04057 eV, −0.21317 eV, and −0.1726 eV, respectively. Additionally, the negative energies of the LUMO and HOMO are characterized by descriptors of electron affinity (EA) and ionization potential (Ip).
Moreover, the chemical potential () is considered the negative descriptor of electronegativity, which can be estimated through
= − (Ip+EA)/2= −0.165215 eV, and the value of electronegativity (χ) is represented by a factor such as (Ip+EA)/2 = 0.165215 eV. Both estimated values are calculated through the estimations of EA and Ip values.
In addition, (η) is used to determine the chemical hardness as = (Ip−EA)/2 = 0.047955 eV, and the value of chemical softness (S) is shown as S = 20.85288 eV, which is the inverse of chemical hardness (η). The additional movement of electrons between the HOMO donor and LUMO acceptor causes a reduction in the energy level, which is referred to as the electrophilicity index ω = /2 η = 0.2846001 eV, which is the electrophilicity index of molecule 5 (Inh. 5).
represents the energy diagrams for Inh. 4 and Inh. 5.
4. Conclusion
A group of new inhibitors; E-octadec-9-enoic acid derivatives were synthesized at high yields (> 90%) from E-octadec-9-enoic acid with primary aromatic amines for the first time. The main objective of the study is to synthesize new inhibitor molecules from monounsaturated fatty acids that can provide effective protection from corrosion of mild steel in acidic media.
Inhibitors Inh. (1–5) show %IE at 86.58, 83.10, 89.63, 91.88, and 77.98 at 1000 μM, respectively by gravimetric methods.
The adsorption of inhibitor molecules onto the surface of mild steel followed a Langmuir adsorption isotherm and the values for the free energy of adsorption ΔGºads indicated that the inhibitors adsorb through a mix of physisorption and chemisorption mechanisms.
Inh.4 showed a mixed type of adsorption with cathodic prevalence, as revealed by the PDP studies. The lowering in the corrosion current densities with the addition of the successively increasing concentrations inhibitor supporting the adsorption and inhibition behavior.
The SEM studies supported the experimentally obtained results by revealing a considerably smooth morphology of the carbon steel surface containing the corrosion inhibitors both in the static as well as in the dynamic condition.
The results are indeed very promising and pave the way to exploit the excellent ability of synthesized compounds to inhibit corrosion of mild steel.
Supplemental Material
Download MS Word (1.8 MB)Disclosure statement
No potential conflict of interest was reported by the authors.
Data availability statement
All data will be available upon request to corresponding author.
Additional information
Funding
References
- Abd-Elaal, A. A., Elbasiony, N. M., Shaban, S. M., & Zaki, E. G. (2018). Studying the corrosion inhibition of some prepared nonionic surfactants based on 3-(4-hydroxyphenyl) propanoic acid and estimating the influence of silver nanoparticles on the surface parameters. Journal of Molecular Liquids. 249, 304–317. doi:10.1016/j.molliq.2017.11.052
- Abdulazeez, I., Zeino, A., Kee, C. W., Al-Saadi, A. A., Khaled, M., Wong, M. W., & Al-Sunaidi, A. A. (2019). Mechanistic studies of the influence of halogen substituents on the corrosion inhibitive efficiency of selected imidazole molecules: A synergistic computational and experimental approach. Applied Surface Science, 471, 494–505. doi:10.1016/j.apsusc.2018.12.028
- Aiad, I., Shaban, S. M., Elged, A. H., & Aljoboury, O. H. (2018). Cationic surfactant based on alignate as green corrosion inhibitors for the mild steel in 1.0 M HCl. Egyptian Journal of Petroleum, 27(4), 877–885. doi:10.1016/j.ejpe.2018.01.003
- Aiad, I., Shaban, S. M., El-Sukkary, M., El-Awady, M., & Soliman, E. (2017). Electrical and gravimetric estimation the corrosion inhibition of three synthesized cationic surfactants N-(3-(butylidene amino) propyl)-N,N-dimethyl alkan-1-ammomium bromide. Materials Performance and Characterization, 6(1), 20170001–20170450. doi:10.1520/MPC20170001
- Aiad, I., Shaban, S. M., Tawfik, S. M., Khalil, M. M. H., & El-Wakeel, N. (2018). Effect of some prepared surfactants on silver nanoparticles formation and surface solution behavior and their biological activity. Journal of Molecular Liquids, 266, 381–392. doi:10.1016/j.molliq.2018.06.089
- Ali, S. A., Al-Muallem, H. A., Rahman, S. U., & Saeed, M. T. (2008a). Bis isoxazolidines: A new class of corrosion inhibitors of mild steel in acidic media. Corrosion Science, 50(11), 3070–3077. doi:10.1016/j.corsci.2008.08.011
- Ali, S. A., Al-Muallem, H. A., Saeed, M. T., & Rahman, S. U. (2008b). Hydrophobic-tailed bicycloisoxazolidines: A comparative study of the newly synthesized compounds on the inhibition of mild steel corrosion in hydrochloric and sulfuric acid media. Corrosion Science, 50(3), 664–675. doi:10.1016/j.corsci.2007.10.010
- Ali, S. A., & El-Sharif, A. M. (2012). Novel class of bisquaternary ammonium salts in inhibition of mild steel corrosion in HCl and H2SO4. Corrosion Engineering, Science and Technology, 47(4), 265–271. doi:10.1179/1743278212Y.0000000004
- Ali, S. A., Haladu, S. A., & El-Sharif, A. M. (2016a). Diallylbis(3-ethoxycarbonylpropyl) ammonium chloride: A symmetrically substituted monomer for the synthesis of an alternate zwitterionic-anionic cyclopolymer. Macromolecular Research, 24(2), 163–169. doi:10.1007/s13233-016-4024-6
- Ali, S. A., Haladu, S. A., & El-Sharif, A. M. (2016b). Synthesis and application of a cyclopolymer bearing a propylphosphonic acid and a propyl carboxylic acid pendants in the same repeating unit. Journal of Polymer Research, 23(8), 167. doi:10.1007/s10965-016-1006-5
- Ali, S. A., Saeed, M. T., & El-Sharif, A. M. (2012). Diallyl-1,12-diaminododecane-based cyclopolymers and their use as inhibitors for mild steel corrosion. Polymer Engineering & Science, 52(12), 2588–2596. doi:10.1002/pen.23224
- Al-Saadi, A. A. (2020). Understanding the influence of electron-donating and electron-withdrawing substituents on the anticorrosive properties of imidazole: A quantum-chemical approach. Arabian Journal for Science and Engineering, 45(1), 153–166. doi:10.1007/s13369-019-04167-0
- Ashassi-Sorkhabi, H., Shaabani, B., & Seifzadeh, D. (2005). Effect of some pyrimidinic Shciff bases on the corrosion of mild steel in hydrochloric acid solution. Electrochimica Acta, 50(16-17), 3446–3452. doi:10.1016/j.electacta.2004.12.019
- Aslam, R., Mobin, M., Aslam, J., Lgaz, H., Chung, I. M., & Zehra, S. (2021). Synergistic inhibition behavior between rhodamine blue and cationic gemini surfactant on mild steel corrosion in 1 M HCl medium. Journal of Molecular Structure, 1228, 129751. doi:10.1016/j.molstruc.2020.129751
- Badr, E. A., Bedair, M. A., & Shaban, S. M. (2018). Adsorption and performance assessment of some imine derivatives as mild steel corrosion inhibitors in 1.0 M HCl solution by chemical, electrochemical and computational methods. Materials Chemistry and Physics, 219, 444–460. doi:10.1016/j.matchemphys.2018.08.041
- Badr, E. A., Hefni, H. H. H., Shafek, S. H., & Shaban, S. M. (2020). Synthesis of anionic chitosan surfactant and application in silver nanoparticles preparation and corrosion inhibition of steel. International Journal of Biological Macromolecules, 157, 187–201. doi:10.1016/j.ijbiomac.2020.04.184
- Badr, E. A., Shafek, S. H., Hefni, H. H., Elsharif, A. M., Alanezi, A. A., Shaban, S. M., & Kim, D. H. (2021). Synthesis of Schiff base-based cationic Gemini surfactants and evaluation of their effect on in-situ AgNPs preparation: Structure, catalytic, and biological activity study. Journal of Molecular Liquids, 326, 115342. doi:10.1016/j.molliq.2021.115342
- Bahlakeh, G., Dehghani, A., Ramezanzadeh, B., & Ramezanzadeh, M. (2019). Highly effective mild steel corrosion inhibition in 1M HCl solution by novel green aqueous Mustard seed extract: Experimental, electronic-scale DFT and atomic-scale MC/MD explorations. Journal of Molecular Liquids, 293, 111559. doi:10.1016/j.molliq.2019.111559
- Beattie, C., North, M., & Villuendas, P. (2011). Proline-catalyzed amination reactions in cyclic carbonate solvents. Molecules, 16(4), 3420–3432. doi:10.3390/molecules16043420
- Dandigunta, B., Karthick, A., Chattopadhyay, P., & Dhoble, A. S. (2021). Impact of temperature and surfactant addition on milk foams. Journal of Food Engineering, 299, 110509. doi:10.1016/j.jfoodeng.2021.110509
- Dehghani, A., Bahlakeh, G., & Ramezanzadeh, B. (2019). Green eucalyptus leaf extract: A potent source of bio-active corrosion inhibitors for mild steel. Bioelectrochemistry (Amsterdam, Netherlands), 130, 107339. doi:10.1016/j.bioelechem.2019.107339
- Dennington, R. K., & Millam, T. (2009). J. GaussView, Version 5. Shawnee Mission, KS: Semichem Inc.
- El-Ashry, E. H., El Nemr, A., & Ragab, S. (2012). Quantitative structure activity relationships of some pyridine derivatives as corrosion inhibitors of steel in acidic medium. Journal of Molecular Modeling, 18(3), 1173–1188. doi:10.1007/s00894-011-1148-7
- Elged, A. H., Shaban, S. M., Eluskkary, M. M., Aiad, I., Soliman, E. A., Elsharif, A. M., & Kim, D. H. (2021). Impact of hydrophobic tails of new phospho-zwitterionic surfactants on the structure, catalytic, and biological activities of AgNPs. Journal of Industrial and Engineering Chemistry, 94, 435–447. doi:10.1016/j.jiec.2020.11.017
- Elsharif, A. M. (2017). Effect of organo-nitrogen compounds on inhibition efficiency of mild steel corrosion in acidic media. Asian Journal of Chemistry, 29(8), 1779–1784. doi:10.14233/ajchem.2017.20631
- Elsharif, A. M., Abubshait, S. A., Abdulazeez, I., & Abubshait, H. A. (2020a). Synthesis of a new class of corrosion inhibitors derived from natural fatty acid: 13-Docosenoic acid amide derivatives for oil and gas industry. Arabian Journal of Chemistry, 13(5), 5363–5376. doi:10.1016/j.arabjc.2020.03.015
- Elsharif, A. M., Abubshait, S. A., Abdulazeez, I., & Abubshait, H. A. (2020b). Synthesis, characterization and corrosion inhibition studies of polyunsaturated fatty acid derivatives on the acidic corrosion of mild steel: Experimental and computational studies. Journal of Molecular Liquids, 319, 114162. doi:10.1016/j.molliq.2020.114162
- Elsherif, A. M., Elged, A. H., & Shaban, S. M. (2021). Controlling effect of hydroxyl phenyl aminopropyl cationic surfactants on the catalytic and biological performance of AgNPs. Surfaces and Interfaces, 27, 101530. doi:10.1016/j.surfin.2021.101530
- Fawzy, A., Abdallah, M., Zaafarany, I. A., Ahmed, S. A., & Althagafi, I. I. (2018). Thermodynamic, kinetic, and mechanistic approach to the corrosion inhibition of carbon steel by new synthesized amino acids-based surfactants as green inhibitors in neutral and alkaline aqueous media. Journal of Molecular Liquids, 265, 276–291. doi:10.1016/j.molliq.2018.05.140
- Feng, L., Yin, C., Zhang, H., Li, Y., Song, X., Chen, Q., & Liu, H. (2018). Cationic gemini surfactants with a bipyridyl spacer as corrosion inhibitors for carbon steel. ACS Omega, 3(12), 18990–18999. doi:10.1021/acsomega.8b03043
- Flores, E. A., Olivares, O., Likhanova, N. V., Domínguez-Aguilar, M. A., Nava, N., Guzman-Lucero, D., & Corrales, M. (2011). Sodium phthalamates as corrosion inhibitors for carbon steel in aqueous hydrochloric acid solution. Corrosion Science. 53(12), 3899–3913. doi:10.1016/j.corsci.2011.07.023
- Fouda, A. S., Elmorsi, M. A., Shaban, S. M., Fayed, T., & Azazy, O. (2018). Evaluation of N-(3-(dimethyl hexadecyl ammonio)propyl) palmitamide bromide as cationic surfactant corrosion inhibitor for API N80 steel in acidic environment. Egyptian Journal of Petroleum, 27(4), 683–694. doi:10.1016/j.ejpe.2017.10.004
- Frisch, M. J., Trucks, G. W., & Schlegel, H. B. (2009). Gaussian 09 Revision C 01 Gaussian 09 , Revision B.01. Wallingford CT: Wallingford.
- Gao, M., Zhang, J., Liu, Q., Li, J., Zhang, R., & Chen, G. (2019). Effect of the alkyl chain of quaternary ammonium cationic surfactants on corrosion inhibition in hydrochloric acid solution. Comptes Rendus Chimie, 22(5), 355–362. doi:10.1016/j.crci.2019.03.006
- Heakal, F. E., & Elkholy, A. (2017). Gemini surfactants as corrosion inhibitors for carbon steel. Journal of Molecular Liquids, 230, 395–407. doi:10.1016/j.molliq.2017.01.047
- Hiremath, S. M., Suvitha, A., Patil, N. R., Hiremath, C. S., Khemalapure, S. S., Pattanayak, S. K., … Obelannavar, K. (2018). Molecular structure, vibrational spectra, NMR, UV, NBO, NLO, HOMO-LUMO and molecular docking of 2-(4, 6-dimethyl-1-benzofuran-3-yl) acetic acid (2DBAA): Experimental and theoretical approach. Journal of Molecular Structure, 1171, 362–374. doi:10.1016/j.molstruc.2018.05.109
- Kamal, M. S., Hussein, I., Mahmoud, M., Sultan, A. S., Saad, M., & A., S. (2018). Oilfield scale formation and chemical removal: A review. Journal of Petroleum Science and Engineering, 171, 127–139. doi:10.1016/j.petrol.2018.07.037
- Khalil, N. (2003). Quantum chemical approach of corrosion inhibition. Electrochim. Acta, 48(18), 2635–2640. doi:10.1016/S0013-4686(03)00307-4
- Lei, Z., Gao, H., Chang, X., Zhang, L., Wen, X., & Wang, Y. (2020). An application of green surfactant synergistically metal supported cordierite catalyst in denitration of Selective Catalytic Oxidation. Journal of Cleaner Production, 249, 119307. doi:10.1016/j.jclepro.2019.119307
- Liang, Y., Li, H., Li, M., Mao, X., Li, Y., Wang, Z., … Hao, X. (2019). Synthesis and physicochemical properties of ester-bonded gemini pyrrolidinium surfactants and a comparison with single-tailed amphiphiles. Journal of Molecular Liquids, 280, 319–326. doi:10.1016/j.molliq.2019.02.018
- Liu, D., Quan, X., Zhu, H., Huang, Q., & Zhou, L. (2020). Evaluation of modified waste concrete powder used as a novel phosphorus remover. Journal of Cleaner Production, 257, 120646. doi:10.1016/j.jclepro.2020.120646
- Liu, J., Wang, D., Gao, L., & Zhang, D. (2016). Synergism between cerium nitrate and sodium dodecylbenzenesulfonate on corrosion of AA5052 aluminium alloy in 3 wt.% NaCl solution. Applied Surface Science, 389, 369–377. doi:10.1016/j.apsusc.2016.07.107
- Liu, Y., Zhang, H., Liu, Y., Li, J., & Li, W. (2019). Inhibitive effect of quaternary ammonium-type surfactants on the self-corrosion of the anode in alkaline aluminium-air battery. The Journal of Power Sources, 434, 226723. doi:10.1016/j.jpowsour.2019.226723
- McCafferty, E. (2005). Validation of corrosion rates measured by the Tafel extrapolation method. Corrosion Science, 47(12), 3202–3215. doi:10.1016/j.corsci.2005.05.046
- McCafferty, E. (2010). Introduction to corrosion science, Springer Science & Business Media, LLC.
- Migahed, M. A., Alsabagh, A. M., Abdou, M. I., Abdel-Rahman, A. A. H., Aboulrous,., & A., A. (2019). Synthesis a novel family of phosphonate surfactants and their evaluation as corrosion inhibitors in formation water. Journal of Molecular Liquids, 281, 528–541. doi:10.1016/j.molliq.2019.02.093
- Migahed, M. A., EL-Rabiei, M. M., Nady, H., Elgendy, A., Zaki1, E. G., Abdou, M. I., & Noamy, E. S. (2017). Novel ionic liquid compound act as sweet corrosion inhibitors for X-65 carbon tubing steel: Experimental and theoretical studies. Journal of Bio- and Tribo-Corrosion, 3, 31.
- Mishrif, M. R., Noor El-Din, M. R., & Khamis, E. A. (2018). Utilization of ethoxylated pentamine oleamide as new Gemini surfactants for corrosion inhibition effectiveness in 1 M HCl solution. Egyptian Journal of Petroleum, 27(4), 1357–1370. doi:10.1016/j.ejpe.2018.09.004
- Mobin, M., & Aslam, R. (2018). Experimental and theoretical study on corrosion inhibition performance of environmentally benign non-ionic surfactants for mild steel in 3.5% NaCl solution. Process Safety and Environmental Protection, 114, 279–295. doi:10.1016/j.psep.2018.01.001
- Nezhad, A. K., Sarikhani, S., Shahidzadeh, E. S., & Panahi, F. (2012). L-Proline-promoted three component reaction of anilines, aldehydes and barbituric acids/malononitrile: Regioselective synthesis of 5-arylpyrimido[4,5-b] quinoline-dionesand2-amino-4-arylquinoline-3 carbonitriles in water. Green Chemistry, 14(10), 2876–2884. doi:10.1039/c2gc35765h
- Pakiet, M., Tedim, J., Kowalczyk, I., & Brycki, B. (2019). Functionalised novel gemini surfactants as corrosion inhibitors for mild steel in 50 mM NaCl: Experimental and theoretical insights. Colloids and Surfaces A: Physicochemical and Engineering Aspects, 580, 123699. doi:10.1016/j.colsurfa.2019.123699
- Pearson, R. G. (1988). Absolute electronegativity and hardness: Application to inorganic chemistry. Inorganic Chemistry, 27(4), 734–740. doi:10.1021/ic00277a030
- Prabhu, R. A., Venkatesha, T. V., Shanbhag, A. V., Kulkarni, G. M., & Kalkhambkar, R. G. (2008). Inhibition effects of some Schiff’s bases on the corrosion of mild steel in hydrochloric acid solution. Corrosion Science, 50(12), 3356–3362. doi:10.1016/j.corsci.2008.09.009
- Ramezanzadeh, M., Bahlakeh, G., & Ramezanzadeh, B. (2019). Study of the synergistic effect of Mangifera indica leaves extract and zinc ions on the mild steel corrosion inhibition in simulated seawater: Computational and electrochemical studies. Journal of Molecular Liquids, 292, 111387. doi:10.1016/j.molliq.2019.111387
- Saad, M. A., Abdurahman, N. H., & Yunus, R. M. (2021). Synthesis, characterization, and demulsification of water in crude oil emulsion via a corn oil-based demulsifier. Mater Today: Proceedings, 42, 251–258. doi:10.1016/j.matpr.2021.01.145
- Shaban, S. M., Aiad, I., El-Sukkary, M. M., Soliman, E. A., & El-Awady, M. Y. (2015). Surface and biological activity of N-(((dimethoxybenzylidene)amino)propyl)-N,N-dimethylalkyl-1-ammonium derivatives as cationic surfactants. Journal of Molecular Liquids. 207, 256–265. doi:10.1016/j.molliq.2015.03.043
- Shaban, S. M., Aiad, I., Moustafa, A. H., & Aljoboury, O. H. (2019). Some alginates polymeric cationic surfactants; surface study and their evaluation as biocide and corrosion inhibitors. Journal of Molecular Liquids, 273, 164–176. doi:10.1016/j.molliq.2018.10.017
- Shaban, S. M., Aiad, I., Yassin, F. A., & Mosalam, A. (2019). The tail effect of some prepared cationic surfactants on silver nanoparticle preparation and their surface, thermodynamic parameters, and antimicrobial activity. Journal of Surfactants and Detergents, 22(6), 1445–1460. doi:10.1002/jsde.12318
- Shaban, S., M., Elbhrawy, M. F., Fouda, A. S., Rashwan, S., M., Ibrahim, H. E., & Elsharif, A. M. (2021). Corrosion inhibition and surface examination of carbon steel 1018 via N-(2-(2-hydroxyethoxy) ethyl)-N, N-dimethyloctan-1-aminium bromide in 1.0 M HCl. Journal of Molecular Structure, 1227, 129713. doi:10.1016/j.molstruc.2020.129713
- Shaban, S. M., Elsamad, S. A., Tawfik, S. M., Abdel-Rahman, A. A. H., & Aiad, I. (2020). Studying surface and thermodynamic behavior of a new multi-hydroxyl Gemini cationic surfactant and investigating their performance as corrosion inhibitor and biocide. Journal of Molecular Liquids, 316, 113881. doi:10.1016/j.molliq.2020.113881
- Shaban, S. M., Elsharif, A. M., Elged, A. H., Eluskkary, M. M., Aiad, I., & Soliman, E. A. (2021). Some new phospho-zwitterionic Gemini surfactants as corrosion inhibitors for carbon steel in 1.0 M HCl solution. Environmental Technology & Innovation, 24, 102051. doi:10.1016/j.eti.2021.102051
- Shaban, S. M., El-Sherif, R. M., & Fahim, M. A. (2018). Studying the surface behavior of some prepared free hydroxyl cationic amphipathic compounds in aqueous solution and their biological activity. Journal of Molecular Liquids, 252, 40–51. doi:10.1016/j.molliq.2017.12.105
- Shamsheera, K. O., Prasad, A. R., Jaseela, P. K., & Joseph, A. (2021). Effect of surfactant addition to Guar Gum and protection of mild steel in hydrochloric acid at high temperatures: Experimental and theoretical studies. Journal of Molecular Liquids, 331, 115807. doi:10.1016/j.molliq.2021.115807
- Sheldon, R. A. (2005). Green solvents for sustainable organic synthesis: State of the art. Green Chemistry, 7(5), 267–278. doi:10.1039/b418069k
- Tabatabaei, M., Asaldous, S., Bahlakeh, G., Ramezanzadeh, B., & Ramezanzadeh, M. (2019a). Green method of carbon steel effective corrosion mitigation in 1M HCl medium protected by Primula vulgaris flower aqueous extract via experimental, atomic-level MC/MD simulation and electronic-level DFT theoretical elucidation. Journal of Molecular Liquids, 284, 658–674. doi:10.1016/j.molliq.2019.04.037
- Tabatabaei, M., Bahlakeh, G., Dehghani, A., Ramezanzadeh, B., & Ramezanzadeh, M. (2019b). Combined molecular simulation, DFT computation and electrochemical studies of the mild steel corrosion protection against NaCl solution using aqueous Eucalyptus leaves extract molecules linked with zinc ions. Journal of Molecular Liquids, 294, 111550. doi:10.1016/j.molliq.2019.111550
- Tabatabaei, M., Ramezanzadeh, M., Ramezanzadeh, B., & Bahlakeh, G. (2020). Production of an environmentally stable anti-corrosion film based on Esfand seed extract molecules-metal cations: Integrated experimental and computer modeling approaches. Journal of Hazardous Materials, 382, 121029.
- Tariq, Z., Kamal, M. S., Mahmoud, M., Alade, O., & Al-Nakhli, A. (2021). Self-destructive barite filter cake in water-based and oil-based drilling fluids. Journal of Petroleum Science and Engineering, 197, 107963. doi:10.1016/j.petrol.2020.107963
- Toghan, A., Abo-Bakr, A. M., Rageh, H. M., & Abd-Elsabour, M. (2019). Green electrochemical strategy for one-step synthesis of new catechol derivatives. RSC Advances, 9(23), 13145–13152. doi:10.1039/c9ra01206k
- Tomasi, J., Menucci, B., & Cammi, R. (2005). Quantum mechanical continuum solvation models. ChemInform, 36(42), 2999–3093. doi:10.1002/chin.200542292
- Tung, N. T., Tran, C. S., Nguyen, T. L., Pham, T. M. H., Chi, S. C., Nguyen, H. A., … Tran, T. Q. (2021). Effect of surfactant on the in vitro dissolution and the oral bioavailability of a weakly basic drug from an amorphous solid dispersion. European Journal of Pharmaceutical Sciences: official Journal of the European Federation for Pharmaceutical Sciences, 162, 105836. doi:10.1016/j.ejps.2021.105836
- Zhang, M., Guo, L., Zhu, M., Wang, K., Zhang, R., He, Z., … Zheng, X. (2021). Akebia trifoliate koiaz peels extract as environmentally benign corrosion inhibitor for mild steel in HCl solutions: Integrated experimental and theoretical investigations. Journal of Industrial and Engineering Chemistry, 101, 227–236. doi:10.1016/j.jiec.2021.06.009
- Zhu, Y., & Free, M. L. (2016). Experimental investigation and modeling of the performance of pure and mixed surfactant inhibitors: Micellization and corrosion inhibition. Colloids and Surfaces A: Physicochemical and Engineering Aspects, 489, 407–422. doi:10.1016/j.colsurfa.2015.11.005