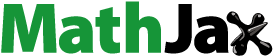
Abstract
Bacterial cellulose (BC) is becoming a promising biopolymeric material due to its potential applications in biomedicine and emerging industrial technologies, among other exciting new uses. In the current work, BC nanofibres were produced by Gluconacetobacter xylinus using alternative sources of nutrients derived from agricultural by-products, pineapple peel and banana extracts, which are cheaper and very abundant. The production yield and thickness of BC produced in a non-conventional culture medium from pineapple peel extract with additional yeast extract and peptone was higher than that produced from Hestrin and Schramm’s medium. Three prominent and characteristic diffraction peaks from X-ray diffraction patterns corresponding to the crystal planes of (110) and (200) indicate a typical cellulose
structure. The Fourier transform infrared spectroscopy of BC fabrics also shows evidence of peaks characteristic of cellulose
Scanning electron microscopy analysis of BC fabrics reveals ribbon-like microfibrils with widths less than 100 nm in all media. Thermogravimetric analysis shows the degradation of BC with similar characteristics among the samples. Alternative sources of nutrients derived from agricultural by-products found in this work could be utilized as a new culture medium to produce suitable BC for industrial applications and its composites in future work.
1. Introduction
The emergence of nanotechnology has led to the new challenges in various areas of research toward the design, development, synthesis and manipulation of functional materials and technological processes. Composite nanomaterials are engineered solid materials that occur when two or more distinct constituent nanomaterials with different physical and chemical properties are mixed to construct new materials in order to produce a new opportunity for their application such as electrodes for supercapacitor, photodegradation, magneto-optical device, magnetotransport and photocatalytic (Dasri & Chingsungnoen, Citation2017; Chen, Younis, Chu, & Li, Citation2019; Chen, Younis, Chu, et al., Citation2019; Sagar et al., Citation2021; Sehar et al., Citation2021). For example, cellulose based bionanocomposites find wide applications in biomedical, electrical and optical devices (Núñez-Carmona et al., Citation2019; Li, Wang, Ma, & Wang, Citation2018; Soemphol et al., Citation2020; Audtarat et al., Citation2022). Cellulose is a natural material that is the most abundant biopolymer. It has become extensively investigated as a promising sustainable matrix with versatile uses. The basic chemical structure of cellulose shows unbranched polymers of D-glucose units covalently linked by β-1,4 glucosidic bonds and arranged in linear chains. The high number of hydroxyl groups of each monomer in the chains enables cellulose to form a crystalline structure. Cellulose can be produced naturally by plants, bacteria, fungi, cellular slime moulds, amoebae and green algae (Ross, Mayer, & Benziman, Citation1991; Harrington & Raper, Citation1968; Itoh, Citation1990). However, the same chemical composition may have a different structural organization. Consequently, the different physical properties are realized. Cellulose extracted from plants has various impurities such as lignin, hemicellulose and pectin that also exist naturally in plant cell walls. Consequently, these impurities can result in a drastic decrease in fibre strength affecting subsequent composites (Li et al., Citation2018). To obtain plant cellulose as a pure material, a purification process is first used to separate these impurities (Menon, Selvakumar, Kumar, & Ramakrishna, Citation2017). In contrast, bacterial cellulose (BC) does not need additional purification steps owing to its very pure nanosized fibrils that form naturally. BC is produced through fermentation by aerobic bacteria, such as acetic acid bacteria, as a pure component of their biofilms (Li et al., Citation2018; Soemphol et al., Citation2020). Due to its unique structural and mechanical properties, the crystallinity, surface area and porosity, water holding capacity and mechanical strength of BC are greater than cellulose from plants (Soemphol et al., Citation2020; Soemphol, Hongsachart, & Tanamool, Citation2018). As a result, BC has received substantial interest for a variety of applications in medicine, biomedical applications and other related fields for which plant cellulose cannot be used. For example, BC has been applied for sensing materials and in electronic devices (Núñez-Carmona et al., Citation2019; Li et al., Citation2018). Moreover, the recent progress in nanotechnology as well as the existing basic knowledge on the BC and BC based composites productions allows the further adaptation of BC fabric, constructing novel BC based biomaterials for many applications (Cacicedo et al., Citation2016; Urbina, Corcuera, Gabilondo, Eceiza, & Retegi, Citation2021).
The aim of this work was to characterize the effects of different carbon and nitrogen sources to produce a general medium of simple and using agricultural waste as low-cost materials in Nong Khai province, north-eastern Thailand, for the BC production when compared to the standard medium of HS. The utilize of these materials could enhance the sustainability of BC production as well as deduce environmental pollution related with the disposal of waste products of agriculture. The potential of BC could go beyond existing applications, especially if constructed in industrial-scale from low-cost feedstock. These applications may include specialty wound dressings in biomedicine field, food packaging, gelling agent in some foods and advanced functional materials as previously presented. Analyses of BC produced in the selected medium were performed of the yield, morphological and structural characterizations by using scanning electron microscopy (SEM), X-ray diffraction (XRD), Fourier transform infrared spectroscopy (FTIR) analysis and thermogravimetric analysis (TGA). This study will contribute to a better understanding of BC as raw material for fabrication or synthesis of this material for potential future applications.
2. Materials and methods
2.1. Bacterial strains and growth conditions
The BC produced by G. xylinus BNKC 19 is used in this study. A stock culture of this strain was streaked on HS culture medium (containing glucose 20 Na2HPO4 6.75
yeast extract 5
peptone 5
and citric acid 1.15
with pH adjusted to 6 and incubation at 30 °C for 3 days. A single colony is then inoculating into 3
of HS culture medium and incubated for 5 days at 30 °C under static conditions. A preculture previously prepared in HS medium was transferred to a screw-cap vial containing 25 mL of HS medium and other alternative media as described below. After cultivation at 30 °C for 7 days, pellicles were collected and boiled in a 0.5 N sodium hydroxide (NaOH) solution for 10 min. The neutralized pellets were then dried at 70 °C for 24 hours, until a constant weight was reached.
2.2. Preparation of culture media for BC production
Pineapple peels and over-ripened bananas were obtained from a local market in Nong Khai Province. These two raw materials were extracted using hot water according to Tanamool, Chantarangsee, and Soemphol (Citation2020). Three culture medium was prepared with total sugar contents corresponding the level of glucose in HS (20 ). These alternative media are designated as whole pineapple peel extract (PP), whole banana extract (BB) and mixed pineapple peel and banana extracts (PB) with each component contributing equally to the level of total sugars. Additionally, supplementation with yeast extract (5
) and peptone (5
) to a culture medium is denoted by the (+) symbol (see Supporting Material, Table S1).
Table 1. Calculated -spacings (
) and crystal dimensions (
) in BC ribbons synthesized in various media compared with HS-Ref (Tokoh et al., Citation2002).
2.3. Production yield and thickness of BC
The production of BC fabric was calculated by the formula Equation(1)(1)
(1) :
(1)
(1)
where
is the dry weight of the BC fabric [in
], and
is the volume of culture medium [in
]. While, the thickness of BC was obtained by using a micrometer (No. 2046 F, Mitutoyo Co., Tsukuba, Japan) in accordance with the KS K ISO 5084 standard.
2.4. Characteristics of BC prepared from various culture media
2.4.1. Scanning electron microscopy (SEM)
SEM was used to observe the microorganism morphology of the membrane. BC was first lyophilized and mounted on SEM grids. Gold particles were coated to the sample surfaces and the specimens observed with a JEOL Model JSM-7800F microscope operated at 3 kV.
2.4.2. X-ray diffraction (XRD)
XRD was performed to reveal the crystallographic nature of BC using a Philips PANalytical X’Pert Powder diffractometer with an operating voltage of 40 kV at 15 mA employing filtered Cu (
) (
) radiation. The XRD spectra were measured using 2θ angles from 5 to
with a step size of 0.2°. The infrared spectra of all samples were analyzed to determine the organic functional groups of the BC. The
-spacings (
) between the crystal planes were determined using Bragg’s law:
(2)
(2)
The apparent crystal dimensions () were calculated using Scherrer’s formula:
(3)
(3)
where
is the crystal dimension,
is the X-ray radiation wavelength,
is full width at half maximum of a specific phase (
) and θ is the Bragg angle.
2.4.3. Fourier transform infrared spectroscopy (FTIR)
FTIR analysis was done using a Shimadzu FTIR spectrometer in an attenuated total reflection mode over a spectral range of 4000–400 cm−1 and with a resolution of 4 cm−1.
2.4.4. Thermogravimetric analysis (TGA)
TGA was carried out using a TA Instruments Model Q5000 TGA. The samples were heated from to
at a rate of
under a nitrogen atmosphere.
3. Results and discussion
In the present study, BC nanofibres were produced by G. xylinus BNKC 19 in alternative media. Several studies reported that BC yields are affected by the available carbon and nitrogen sources (Ruka, Simon, & Dean, Citation2012; Mikkelsen, Flanagan, Dykes, & Gidley, Citation2009; Aswini, Gopal, & Uthandi, Citation2020). Therefore, the carbon sources of alternative media were determined. It was found that there were two sugars, glucose and fructose, in pineapple peel extract (PP) at concentrations of 4.834 and 6.910
respectively. Banana extract (BB) had three major sugars in significant concentrations, glucose, fructose, and sucrose at respective concentrations of 19.393
22.741
and 5.921
(raw data not shown). The total sugar levels of all alternative media were calculated and found to be equal to the glucose content of the HS medium. The effects of the alternative media compared with HS medium on the production yield and the thickness of BC fabric mats are shown in . The BC production yield of G. xylinus BNKC 19 in BB and PP media were 0.27 and 0.29
respectively, lower than for the HS medium (0.48
), while the BP medium was 0.54
The thickness of BC fabric mats showed trends that matched BC production yields. Considering the carbon source, BB and PP alternative media contained total sugar contents same as HS medium therefore, it was suggested that both media had insufficient nutrients for BC production. However, the BB medium differed from the performance reported by Molina-Ramírez et al. (Molina-Ramírez, Castro, Zuluaga, & Gañán, Citation2018), who reported that a rotted banana medium promoted higher BC production than the HS medium. It is noteworthy that BP medium (a mixture of BB and PP media) showed good production and thicker BC fabric mats than the HS medium. This BP medium contained glucose, fructose and sucrose at 40.8%, 53% and 6.2% of the total sugars, respectively. This shows that a carbon source comprised of various sugars promotes BC production. Although, glucose is necessary for cellulose synthesis, several reports found that additional fructose and sucrose provided excellent results and enabled production of BC by the genus, Gluconacetobacter (Mikkelsen et al., Citation2009; Sijabat, Nuruddin, Aditiawati, & Sunendar Purwasasmita, Citation2020). In addition to the different sugar contents, there are other factors that may affect BC production. It has been reported that pineapple waste contains a low mineral content, but it has reducing sugars and proteins, which are nutrients for the growth of microorganism (Hemalatha & Anbuselvi, Citation2013; Omwango, Njagi, Orinda, & Wanjau, Citation2013; Suwannasing, Imai, & Kaewkannetra, Citation2015). There are several reports that bananas contain vitamins, minerals, amino acids and proteins that can be used in culture media (Ashokkumar, Elayabalan, Shobana, Sivakumar, & Pandiyan, Citation2018; Emaga, Andrianaivo, Wathelet, Tchango, & Paquot, Citation2007; Molina-Ramírez, Cañas-Gutiérrez, Castro, Zuluaga, & Gañán, Citation2020). Although, the carbon source in a culture medium is an important component, other nutrients such as mineral ions, vitamins and amino acids are also essential for cellulose bacteria because they are required in enzymatic cellulose synthesis (Almeida, Prestes, Fonseca, Woiciechowski, & Wosiacki, Citation2013; Mohite, Kamalja, & Patil, Citation2012; Raghavendran, Asare, & Roy, Citation2020; Son et al., Citation2003).
Figure 1. Effects of various extract culture media on BC production, (a) the production yield and (b) thickness of dried BC sheets.

To investigate the effect of nitrogen source, yeast extract and peptone were added in alternative media at a 0.5% level, as in the HS medium. As shown in , all alternative media with supplemental nitrogen sources (+) produced a significantly higher BC yields and fabric mat thicknesses. Among all the alternative media, the + BB sample presented the highest production yield, 1.54, followed by + BP (1.49 ) and + PP (0.91
). Similarly, as shown in , the thickest BC sheet was obtained from the + BB sample (0.065 mm), while + PP and + BP presented sheet thicknesses of 0.055 mm and 0.042 mm, respectively. Yeast extract and peptone addition to the alternative media caused a several fold increase in BC production yield and BC thickness. Nitrogen is the main component of some precursors, such as nitrogenous bases, nucleosides and nucleotides. They play important roles in the cellulose biosynthesis (Ross et al., Citation1991). These results are similar to those of several authors who found that yeast extract and peptone were indispensable nitrogen sources for cell growth and BC production (Soemphol et al., Citation2018; Aswini et al., Citation2020; Sijabat et al., Citation2020; Mohammadkazemi, Azin, & Ashori, Citation2015). BC production from various media were observed and the results are shown in . It was found that BC gels from all the alternative media with yeast extract and peptone were more yellowish to orange in colour. This colour may have been caused by metabolites or pigments in the cellulose biosynthesis process of G. xylinus BNKC19. The BC gels were quite soft more than those produced in HS medium. This may be due to an incomplete cellulose biosynthesis process in the absence of a supplemental nitrogen source. After the BC pellicles were cleaned in NaOH solutions to remove G. xylinus and all associated biomass, purified white pellicles were observed (see ).
Figure 2. Images of BCs produced by (a) HS medium, (b) BB medium, (c) PP medium, (d) BP medium, (e) +BB medium, (f) +PP medium, (g) + BP and (h) after treatment in NaOH.

The surface morphology of freeze-dried BC samples by SEM display formation of ribbon-like nanofibrils forming a highly fibrous structure. In addition, most of the nanofibrils were found to have wide ranging from 40 to 70 nm. While nanofibrils lengths could not be determined since their ends not be seen, even at high magnification (×30K). G. xylinus strain of bacteria can convert glucose and other organic substrates to cellulose. The BC produced from different agricultural waste media and carbon sources have almost the same chemical and physical properties as those produced from standard HS media (). Meanwhile, three are research that provides an idea of the potential of waste products for the BC production of environmentally friendly and inexpensive (Revin, Liyaskina, Nazarkina, Bogatyreva, & Shchankin, Citation2018; Li et al., Citation2015; Waleed, Taous, Mazhar, Fazli, & Joong, Citation2015). The images revealed some differences in the BC surface structure modified by carbon and nitrogen sources. It shows the dense tangle of cellulose fibres. This may be from various sugars from agricultural waste and nitrogen added to the culture medium as they affect the potential for cellulose production (Ruka et al., Citation2012; Mikkelsen et al., Citation2009; Aswini et al., Citation2020). However, it retains its weaving properties. During cultivation, the morphology of BC changes from floccus to film. Microfibres intertwine and merge, forming an irregular mesh (Lahiri et al., Citation2021). The crystal dimensions of each BC sample could be determined from the XRD results ().
Figure 3. SEM of freeze-dried BC from cultures of (a) HS medium, (b) BB medium, (c) PP medium, (d) BP medium, (e) +BB medium, (f) +PP medium and (g) + BP medium after treatment in NaOH.

Figure 4. X-ray diffraction diagrams of freeze-dried BCs produced on (a) HS medium, (b) BB medium, (c) PP medium, (d) BP medium, (e) +BB medium, (f) +PP medium and (g) + BP medium.

The XRD patterns obtained from the BC samples, collected over the 2θ ranging from to
are shown in . All BC samples had three prominent characteristic diffraction peaks at
values in the ranges of
and
corresponding to the crystal planes
(110) and (200), respectively (Castro et al., Citation2011; Vazquez, Foresti, Cerrutti, & Galvagno, Citation2013; Tokoh, Takabe, Sugiyama, & Fujita, Citation2002; Sugiyama, Persson, & Chanzy, Citation1991). These peaks indicate a typical cellulose
structure (Soemphol et al., Citation2018). Diffraction peaks at 2θ values of below
should be associated with a sequence of chemical reaction products formed from organic and other compounds in the in the composition of the sample. No peaks are found around
and 12.1° which correspond to the characteristics of cellulose II (Soemphol et al., Citation2018; Tokoh et al., Citation2002). X-ray diffraction results on BC samples in were used to calculate the crystal dimensions (
) and
-spacings (
) of the
(110) and (200) planes using EquationEqs. (2)
(2)
(2) and Equation(3)
(3)
(3) , respectively. The results are presented in . It was observed that
-spacing values of the (200) plane were narrower than for the
plane. However, the
-spacings were narrower than in the
plane. The obtained
-spacing values were found to correspond to those reported by Tokoh et al. (Tokoh et al., Citation2002). It was reported that
-spacing values of 0.621 nm, 0.528 nm, and 0.397 nm are those of cellulose type
while values of 0.607 nm, 0.535 nm, and 0.397 nm are of cellulose type
(Sugiyama et al., Citation1991). Therefore, the obtained
-spacing values of the (200) plane in
were the same as the corresponding plane in
while those of the
plane were narrower. Those of the (110) plane were wider than the corresponding plane in
It was found that cellulose I has two polymorphs, a triclinic structure (
) and a monoclinic structure (
). The
unit cell contains two parallel chains, whereas
contains a single chain (Soemphol et al., Citation2018). It has been reported that cellulose
forms smaller sized microfibrils than cellulose
(Keshk, Citation2014). Furthermore, cellulose
can be formed irreversibly from cellulose Iα (Keshk, Citation2014).
FT-IR was utilized to characterize the functional groups and examine the various structures of the BC samples. The results are shown in . The FT-IR spectra of BC samples prepared from the various media were compared with the standard HS medium. It was observed that the FT-IR spectra of the BC fabric mats were similar to that of material from the HS medium, suggesting that BC cellulose has the same chemical structure. Various characteristic BC peaks were observed at around
and
corresponding to the complex nature of this biological material. The broad peak at
corresponds to the O-H stretching of the cellulose triclinic
allomorph and the monoclinic
form. (Tokoh et al., Citation2002; Sugiyama et al., Citation1991; Keshk, Citation2014; Costa, Almeida, Vinhas, & Sarubbo, Citation2017; Stumpf, Pértile, Rambo, & Porto, Citation2013). A distinguishing peak of the BC fabric was observed at around
that is attributed to CH2 stretching, and the peak at around
originated from the H–O–H bending vibrations of absorbed water molecules. The spectrum showed a strong band at around
that could be associated with ether C–O–C functionalities (Tokoh et al., Citation2002; Sugiyama et al., Citation1991; Keshk, Citation2014; Costa et al., Citation2017; Stumpf et al., Citation2013). The spectrum band at around
corresponds to C–O bond stretching of cellulose. A band of the BC fabric observed around
is typical of
-linked glucose polymers. Moreover, the weak band peaks located at around
and
correspond to O–H in plane vibrations, C–H bending, CH2 wagging, O–H in-plane bending and either CH2 symmetrical bending or surface carboxylate groups, respectively (Sugiyama et al., Citation1991).
Figure 5. FTIR of freeze-dried BC from cultures of (a) HS medium, (b) BB medium, (c) PP medium, (d) BP medium, (e) +BB medium, (f) +PP medium and (g) + BP medium.

The mass loss of the dried BC pellicles, examined by TGA, was shown in . The TG curves in each sample reveals the significant stages of mass loss with a rising temperature from 30 °C to 550 °C of the samples. First was observed during the initial thermal treatment, starting at room temperature up to 150 °C. This stage causes of water losses (Mohammadkazemi et al., Citation2015). The mass loss slightly decreases and no significant differences between BC samples were observed. It is suggested that there is no difference in moisture content between these BC samples. The second mass loss in the temperature region between and
and it is assigned to a decomposition process. At around
the decomposition of the dried BC pellicles resulted in a significant mass loss of 70–80%. This may be associated with a cellulose degradation process such as depolymerization, dehydration and decomposition of glucosyl units followed by the formation of lower molecular weight gaseous products from breakdown of the charred residue (Gea, Reynolds, Roohpour, & Wirjosentono, Citation2011; George, Ramana, Bawa, & Siddaramaiah, Citation2011; Roman & Winter, Citation2004). Since the thermal degradation behavior has been known to be affected by some structural parameters such as such as crystallinity, orientation and molecular weight of the fibre (Um et al., Citation2004). Therefore, the decrease in weight of BC at all stages could be observed due to its higher crystallinity, degree of polymerization and compact interweaving structure.
Figure 6. TGA of freeze-dried BC from cultures of (a) HS medium, (b) BB medium, (c) PP medium, (d) BP medium, (e) +BB medium, (f) +PP medium and (g) + BP medium.

It was found that the BC nanofibres can be produced by G. xylinus cultivated in non-conventional media derived from the agricultural waste products, pineapple peel and banana extracts. Characterization by means of SEM, XRD, FTIR and TGA analysis suggests that the suitability of using pineapple peel and banana extracts as carbon and nitrogen sources for production of BC nanofibrils. The resulting materials have similar characteristics to those obtained using more expensive carbon and nitrogen sources such as glucose or commercial glycerol. Alternatively, the low cost of non-conventional media derived from agricultural waste products also contributes to the economic feasibility of this bioprocess.
4. Conclusions
This work demonstrates the feasibility of using a new isolate, G. xylinus BNKC 19, in the production of BC from pineapple peel and banana extracts. It is an alternative utilization of agricultural waste products that has potential economic and environmental benefits. The production yield was of sufficient value due to the low cost of raw materials. The BC samples were characterized using SEM, XRD, FTIR and TGA analysis. SEM images show a reticulated structure consisting of ultrafine nanofibrils and no significant difference in size. FTIR spectra illustrate that the chemical structure of the nanofibrils obtained from the different culture medium sources assayed was unaffected. Crystalline parameters investigated by XRD indicate triclinic structure () and monoclinic structure (
) crystalline form in the BC pellicles. The production of BC was of relatively high yield with properties similar to those of material produced in HS medium. These results suggest that it is possible to produce BC from low-cost resources on a larger scale for industrial applications and its composites in future work.
Supplemental Material
Download MS Word (13.6 KB)Acknowledgements
The authors acknowledge the Faculty of Interdisciplinary Studies, Khon Kaen University, for supporting facilities. And, we special thank Asst. Dr. Wichai Soemphol for all advising.
Disclosure statement
No potential conflict of interest was reported by the author(s).
Additional information
Funding
References
- Almeida, D. M., Prestes, R. A., Fonseca, A. F., Woiciechowski, A. L., & Wosiacki, G. (2013). Minerals consumption by Acetobacter xylinum on cultivation medium on coconut water. Brazilian journal of Microbiology: [Publication of the Brazilian Society for Microbiology], 44(1), 197–206. doi:10.1590/S1517-83822013005000012
- Ashokkumar, K., Elayabalan, S., Shobana, V. G., Sivakumar, P., & Pandiyan, M. (2018). Nutritional value of cultivars of Banana (Musa spp.) and its future prospects. Journal of Pharmacognosy and Phytochemistry, 7(3), 2972–2977.
- Aswini, K., Gopal, N. O., & Uthandi, S. (2020). Optimized culture conditions for bacterial cellulose production by Acetobacter senegalensis MA1. BMC biotechnology, 20(1), 46. doi:10.1186/s12896-020-00639-6
- Audtarat, S., Hongsachart, P., Dasri, T., Chio-Srichan, S., Soontaranon, S., Wongsinlatam, W., & Sompech, S. (2022). Green synthesis of silver nanoparticles loaded into bacterial cellulose for antimicrobial application. Nanocomposites, 8(1), 34–46. doi:10.1080/20550324.2022.2055375
- Cacicedo, M. L., Castro, M. C., Servetas, I., Bosnea, L., Boura, K., Tsafrakidou, P., … Castro, G. R. (2016). Progress in bacterial cellulose matrices for biotechnological applications. Bioresource Technology, 213, 172–180. doi:10.1016/j.biortech.2016.02.071
- Castro, C., Zuluaga, R., Putaux, J.-L., Caro, G., Mondragon, I., & Gañán, P. (2011). Structural characterization of bacterial cellulose produced by Gluconacetobacter swingsii sp. from Colombian agroindustrial wastes. Carbohydrate Polymers, 84(1), 96–102. doi:10.1016/j.carbpol.2010.10.072
- Chen, N., Younis, A., Chu, D., & Li, S. (2019). Controlled fabrication of Pr(OH)3 nanowires for enhanced photocatalytic activities. Journal of Rare Earths, 37(1), 60–67. doi:10.1016/j.jre.2018.03.035
- Chen, N., Younis, A., Huang, S., Chu, D., & Li, S. (2019). Advanced three-dimensional hierarchical Pr6O11@Ni-Co oxides-based core-shell electrodes for supercapacitance application. Journal of Alloys and Compounds, 783, 772–778. doi:10.1016/j.jallcom.2018.12.247
- Costa, A. F. S., Almeida, F. C. G., Vinhas, G. M., & Sarubbo, L. A. (2017). Production of bacterial cellulose by Gluconacetobacter hansenii using corn steep liquor as nutrient sources. Frontiers in Microbiology, 8, 2027. doi:10.3389/fmicb.2017.02027
- Dasri, T., & Chingsungnoen, A. (2017). Enhancement of the magneto-optical Kerr effect signal using bilayer metallic structures consisting of Co/noble metal. Materials Research Express, 4(12), 126203. doi:10.1088/2053-1591/aa9c8b
- Emaga, T. H., Andrianaivo, R. H., Wathelet, B., Tchango, J. T., & Paquot, M. (2007). Effects of the stage of maturation and varieties on the chemical composition of banana and plantain peels. Food Chemistry, 103(2), 590–600. doi:10.1016/j.foodchem.2006.09.006
- Gea, S., Reynolds, C. T., Roohpour, N., & Wirjosentono, B. (2011). Investigation into the structural, morphological, mechanical and thermal behavior of bacterial cellulose after a two-step purification process. Bioresource Technology, 02(19), 9105–9110. doi:10.1016/j.biortech.2011.04.077
- George, J., Ramana, K. V., Bawa., & A. S., Siddaramaiah. (2011). Bacterial cellulose nanocrystals exhibiting high thermal stability and their polymer nanocomposites. International Journal of Biological Macromolecules, 48(1), 50–57., doi:10.1016/j.ijbiomac.2010.09.013
- Harrington, B. J., & Raper, K. B. (1968). Use of a fluorescent brightener to demonstrate cellulose in the cellular slime molds. Applied Microbiology, 16(1), 106–113. doi:10.1128/am.16.1.106-113.1968
- Hemalatha, R., & Anbuselvi, S. (2013). Physicohemical constituents of pineapple pulp and waste. Journal of Chemical and Pharmaceutical Research, 5(2), 240–242.
- Itoh, T. (1990). Cellulose synthesizing complexes in some giant marine algae. Journal of Cell Science, 95(2), 309–319. doi:10.1242/jcs.95.2.309
- Keshk, S. M. (2014). Bacterial cellulose production and its industrial applications. Journal of Bioprocessing & Biotechniques, 4(2), 150. doi:10.4172/2155-9821.1000150
- Lahiri, D., Nag, M., Dutta, B., Dey, A., Sarkar, T., Pati, S., … Ray, R. R. (2021). Bacterial cellulose: Production, characterization, and application as antimicrobial agent. International Journal of Molecular Sciences, 22(23), 12984. doi:10.3390/ijms222312984
- Li, Z., Wang, L., Hua, J., Jia, S., Zhang, J., & Liu, H. (2015). Production of nano bacterial cellulose from waste water of candied jujube processing industry using Acetobacter xylinum. Carbohydrate Polymers, 120, 115–119. doi:10.1016/j.carbpol.2014.11.061
- Li, Y.-Y., Wang, B., Ma, M.-G., & Wang, B. (2018). Review of recent development on preparation, properties, and applications of cellulose-based functional materials. International Journal of Polymer Science, 2018, 8973643. doi:10.1155/2018/8973643
- Menon, M. P., Selvakumar, R., Kumar, P. S., & Ramakrishna, S. (2017). Extraction and modification of cellulose nanofibers derived from biomass for environmental application. RSC Advances, 7(68), 42750–42773. doi:10.1039/C7RA06713E
- Mikkelsen, D., Flanagan, B. M., Dykes, G. A., & Gidley, M. J. (2009). Influence of different carbon sources on bacterial cellulose production by Gluconacetobacter xylinus strain ATCC 53524. Journal of Applied Microbiology, 107(2), 576–583. doi:10.1111/j.1365-2672.2009.04226.x
- Mohammadkazemi, F., Azin, M., & Ashori, A. (2015). Production of bacterial cellulose using different carbon sources and culture media. Carbohydrate Polymers, 117, 518–523. doi:10.1016/j.carbpol.2014.10.008
- Mohite, B. V., Kamalja, K. K., & Patil, S. V. (2012). Statistical optimization of culture conditions for enhanced bacterial cellulose production by Gluconacetobacter hansenii NCIM 2529. Cellulose, 19(5), 1655–1666. doi:10.1007/s10570-012-9760-y
- Molina-Ramírez, C., Cañas-Gutiérrez, A., Castro, C., Zuluaga, R., & Gañán, P. (2020). Effect of production process scale-up on the characteristics and properties of bacterial nanocellulose obtained from overripe banana culture medium. Carbohydrate polymers, 240(2), 116341. doi:10.1016/j.carbpol.2020.116341
- Molina-Ramírez, C., Castro, C., Zuluaga, R., & Gañán, P. (2018). Physical characterization of bacterial cellulose produced by Komagataeibacter medellinensis using food supply chain waste and agricultural by-products as alternative low-cost feedstocks. Journal of Polymers and the Environment, 26(2), 830–837. doi:10.1007/s10924-017-0993-6
- Núñez-Carmona, E., Bertuna, A., Abbatangelo, M., Sberveglieri, V., Comini, E. G., & Sberveglieri, G. (2019). BC-MOS: The novel bacterial cellulose based MOS gas sensors. Materials Letters, 237, 69–71. doi:10.1016/j.matlet.2018.11.011
- Omwango, E. O., Njagi, E. N. M., Orinda, G. O., & Wanjau, R. N. (2013). Nutrient enrichment of pineapple waste using Aspergillus niger and Trichoderma viride by solid state fermentation. African Journal of Biotechnology, 12(43), 6193–6196. doi:10.5897/AJB2013.12992
- Raghavendran, V., Asare, E., & Roy, I. (2020). Bacterial cellulose: Biosynthesis, production, and applications. Advances in Microbial Physiology, 77, 89–138. doi:10.1016/bs.ampbs.2020.07.002
- Revin, V., Liyaskina, E., Nazarkina, M., Bogatyreva, A., & Shchankin, M. (2018). Cost-effective production of bacterial cellulose using acidic food industry by-products. Biotechnology and Industrial Microbiology, 49(1), 151–159. doi:10.1016/j.bjm.2017.12.012
- Roman, M., & Winter, W. T. (2004). Effect of sulfate groups from sulfuric acid hydrolysis on the thermal degradation behavior of bacterial cellulose. Biomacromolecules, 5(5), 1671–1677. doi:10.1021/bm034519+
- Ross, P., Mayer, R., & Benziman, M. (1991). Cellulose biosynthesis and function in bacteria. Microbiological Reviews, 55(1), 35–58. doi:10.1128/mr.55.1.35-58.1991
- Ruka, D. R., Simon, G. P., & Dean, K. M. (2012). Altering the growth conditions of Gluconacetobacter xylinus to maximize the yield of bacterial cellulose. Carbohydrate Polymers, 89(2), 613–622. doi:10.1016/j.carbpol.2012.03.059
- Sagar, R. U. R., Shabbir, B., Hasnain, S. M., Mahmood, N., Zeb, M. H., Shivananju, B. N., … Younis, A. (2021). Shape-controlled synthesis of cerium oxide nanoparticles for efficient dye photodegradation and antibacterial activities. Applied Organometallic Chemistry, 35(1), e6069. doi:10.1002/aoc.6069
- Sehar, S.,Naz, I.,Rehman, A.,Sun, W.,Alhewairini, S.S.,Zahid, M.N., &Younis, A. (2021). Shape-controlled synthesis of cerium oxide nanoparticles for efficient dye photodegradation and antibacterial activities. Applied Organometallic Chemistry, 35(1), e6069.
- Sijabat, E. K., Nuruddin, A., Aditiawati, P., & Sunendar Purwasasmita, B. (2020). Optimization on the synthesis of bacterial nano cellulose (BNC) from banana peel waste for water membrane applications. Materials Research Express, 7(5), 055010. doi:10.1088/2053-1591/ab8df7
- Soemphol, W., Charee, P., Audtarat, S., Sompech, S., Hongsachart, P., & Dasri, T. (2020). Characterization of a bacterial cellulose-silica nanocomposite prepared from agricultural waste products. Materials Research Express, 7(1), 015085. doi:10.1088/2053-1591/ab6c25
- Soemphol, W., Hongsachart, P., & Tanamool, V. (2018). Production and characterization of bacterial cellulose produced from agricultural by-product by Gluconacetobacter strains. Materials Today: Proceedings, 5(5), 11159–11168. doi:10.1016/j.matpr.2018.01.036
- Son, H. J., Kim, H. G., Kim, K. K., Kim, H. S., Kim, Y. G., & Lee, S. J. (2003). Increased production of bacterial cellulose by Acetobacter sp. V6 in synthetic media under shaking culture conditions. Bioresource Technology, 86(3), 215–219. doi:10.1016/S0960-8524(02)00176-1
- Stumpf, T. R., Pértile, R. A. N., Rambo, C. R., & Porto, L. M. (2013). Enriched glucose and dextrin mannitol-based media modulates fibroblast behavior on bacterial cellulose membranes. Materials Science & Engineering C, Materials for Biological Applications, 33(8), 4739–4745. doi:10.1016/j.msec.2013.07.035
- Sugiyama, J., Persson, J., & Chanzy, H. (1991). Combined infrared and electron diffraction study of the polymorphism of native celluloses. Macromolecules, 24(9), 2461–2466. doi:10.1021/ma00009a050
- Suwannasing, W., Imai, T., & Kaewkannetra, P. (2015). Potential utilization of pineapple waste streams for polyhydroxyalkanoates (PHAs) production via batch fermentation. Journal of Water and Environment Technology, 13(5), 335–347. doi:10.2965/jwet.2015.335
- Tanamool, V., Chantarangsee, M., & Soemphol, W. (2020). Simultaneous vinegar fermentation from a pineapple by-product using the co-inoculation of yeast and thermotolerant acetic acid bacteria and their physiochemical properties. 3 Biotech, 10(3), 115. doi:10.1007/s13205-020-2119-4
- Tokoh, C., Takabe, K., Sugiyama, J., & Fujita, M. (2002). CP/MAS 13C NMR and electron diffraction study of bacterial cellulose structure affected by cell wall polysaccharides. Cellulose, 9(3/4), 351–360. doi:10.1023/A:1021150520953
- Um, I. C., Ki, C. S., Kweon, H. Y., Lee, K. G., Ihm, D. W., & Park, Y. H. (2004). Wet spinning of silk polymer II. Effect of drawing on the structure characteristics and properties of filament. International journal of Biological Macromolecules, 34(1-2), 107–119. doi:10.1016/j.ijbiomac.2004.03.011
- Urbina, L., Corcuera, M. A., Gabilondo, N., Eceiza, A., & Retegi, A. (2021). A review of bacterial cellulose: Sustainable production from agricultural waste and applications in various fields. Cellulose, 28(13), 8229–8253. doi:10.1007/s10570-021-04020-4
- Vazquez, A., Foresti, M. L., Cerrutti, P., & Galvagno, M. (2013). Bacterial cellulose from simple and low cost production media by Gluconacetobacter xylinus. Journal of Polymers and the Environment, 21(2), 545–554. doi:10.1007/s10924-012-0541-3
- Waleed, A.-K., Taous, K., Mazhar, U.-I., Fazli, W., & Joong, K.-P. (2015). Production, characterization and physicomechanical properties of bacterial cellulose from industrial wastes. Journal of Polymers and the Environment, 23, 45–53.