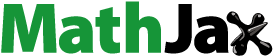
Abstract
Microplastic and metal waste from electronic industries are becoming a major threat to the environment and marine ecosystem. Green processing and employing natural derived materials are solutions to this issue. In this work, an electrical double-layer capacitor (EDLC) fabricated from green polymer electrolyte (GPE) sandwiched in between two microbial cellulose-based electrodes is characterized. The eco-friendly electrode films of interconnected cellulose and multiwalled carbon nanotube (MWCNT) are obtained via harmless, inexpensive, and simple procedure. The GPE consists of methylcellulose-potato starch blend as polymer blend and ammonium iodide (NH4I) is chosen as ion provider. Glycerol serves as plasticization agent for alternative pathways enabling ionic migration. The most optimum GPE possesses good ionic conductivity of ∼ 10−3 S/cm. Ions are the dominant charge carrier in the GPE as ion transference number shown to be close to unity. Linear sweep voltammetry (LSV) analysis illustrated that the GPE is electrochemically stable up to 2.4 V. The green EDLC stores energy through non-Faradaic mechanism and the specific capacitance from charge-discharge, Ccd is influenced by the sweep rates. The EDLC can be charged and discharged up to 2 V with a stable 1000 cyclability performance. This work implied the potential of microbial cellulose-based EDLC as ideal green-based energy storage device for low voltage applications such as smart electronic textiles.
1. Introduction
The demand for energy storage as well as power backup systems has increased the use and development of electronic devices that can accumulate electrical energy produced. The most popular and common energy storage devices are batteries, e.g. lithium-ion batteries and lead acid batteries (Gautham Prasad, Shetty, Thakur, Bommegowda, & Rakshitha, Citation2019). There is no doubt that the conventional lithium-ion batteries have outstanding performance and have been opted for technologies such as electric cars, laptops, and smartphones. Application of cobalt in lithium-ion batteries has become a threat to the environment. Other than that, lithium can be explosive (Qadir & Gulshan, Citation2018). Supercapacitors (SCs) are one of the alternatives because of their simplicity, low cost, and safe preparation procedures (Sahin, Blaabjerg, & Sangwongwanich, Citation2021). SCs can be categorized into three major types which are electrochemical double layer capacitor (EDLC), pseudocapacitor and hybrid capacitor. Each of these capacitors have different energy storage mechanism subject to different choices of electrode materials (Tshiani & Umenne, Citation2022).
The energy storage mechanism of EDLC includes non-Faradaic process as ions from the polymer electrolyte form double-layer on its carbon-based electrodes (Pan, Yang, Zhang, Gao, & Wang, Citation2020). EDLC has some unique features such as good thermal stability, large cycling stability, excellent reversibility, high power density, rapid charge-discharge rate, cost efficient, safe, and easy fabrication method (Joseph et al., Citation2020). One of the most suitable materials for EDLC applications is carbon, owing to its high mechanical strength, electrical conductivity, abundance, controllable structure, and low density. Numerous forms of carbon, e.g. carbon black, carbon nanotube (CNT), natural or artificial graphite, graphene, and activated carbon, are used in commercial SCs as active materials or conductive additives to form ionic polarization process (Li et al., Citation2018; Tiwari et al., Citation2021; Wu & Zhong, Citation2019). Among them, CNT shows superior electrical conductivity, intrinsic high-aspect ratio, excellent mechanical strength, and chemical stability especially when integrated into polymeric materials (Yang et al., Citation2021). Previous work by Deng et al. shows that inclusion of 6 wt.% CNT into fibrous cellulose acetate network resulted in 125% increment of electrochemical capacitance at current density of 10 A/g as porous SC electrode (Deng et al., Citation2013).
Microplastics are currently a global problem that can seriously affect human health and the ocean (Haque & Fan, Citation2022). These plastics travel through domestic water supply which end up in marine ecosystem in a form of pellet, scrubber, fibers, or fragments consisted of various non-biodegradable synthetic polymers, e.g. polyvinylidene fluoride (PVdF), polyacrylonitrile (PAN), polyurethane (PUR), polyvinyl chloride (PVC) and polymethyl methacrylate (PMMA) (Firdousie, Ahmed, Hussain, Nath, & Bhutia, Citation2022; Yuan, Nag, & Cummins, Citation2022). Batteries and SC containing PMMA or PVdF employed as polymer electrolyte or electrode binder are sources of the microplastics. Thus, to replace these expensive and non-biodegradable binders, green microbial cellulose synthesized from symbiotic culture of bacteria and yeast (SCOBY) has recently been explored as binder material for the electrodes. The web-like nanostructures of microbial cellulose enabled relatively easy doping of 2D active material like CNT to form a high surface area electrode. A viable way to fabricate a CNT-based electrode is by relying on synergistic effect of hydrogen bonding interactions between hydroxyl functional groups of CNT and microbial cellulose that consequently eliminates the need of toxic solvents in processing (Hamsan et al., Citation2022; Sa’aya, Demon, Abdullah, Azmi, & Halim, Citation2023). The microbial cellulose-CNT composite also works as metal-free electrode. It can be readily employed without the need to coat carbon paste onto a metal foil, as it always has been for commercial SC. Other advantageous characteristics of microbial cellulose are bendable, excellent mechanical strength and excellent film forming properties (Cazón, Puertas, & Vázquez, Citation2022; Landi et al., Citation2021).
shows proposed EDLC construction made by microbial cellulose-CNT based electrodes and green polymer electrolyte. The polymer electrolyte is another key component in achieving green, safe, and biodegradable SCs. The term green is used to describe methods and non-toxic materials that are safe to environment such as natural polymers. Natural polymers are in abundance, inexpensive and some of them can be found in our kitchen (Sohaimy & Isa, Citation2022). Two major sources of natural polymers are proteins (gelatin, zein, soy protein isolates etc.) and polysaccharides (chitosan, cellulose, pectin, carrageenan, dextran, starch, agar, etc.) can be easily obtained from animal- and plant-based food waste. Methylcellulose is one example of cellulose derivative which is extracted from methylation of alkali cellulose. Methylcellulose has good film forming properties, good solubility in water as well as good electrical and mechanical properties (Silva et al., Citation2022). Starch is another great abundance and cheap natural polymer. The structure of starch has linear amylose (poly-α-1,4-D-glucopyranoside) and branched amylopectin (poly-α-1,4-D-glucopyranoside and α-1,6-D-glucopyranoside) (Fu et al., Citation2022).
Figure 1. Proposed EDLC construction with green polymer electrolyte sandwiched in between microbial cellulose-CNT composite electrodes.

Polymer blending is an effective method to gain desired combination of electrical, thermal, and structural properties in composite material (Jordá-Reolid, Ibáñez-García, Catani, & Martínez-García, Citation2022). Both methylcellulose and potato starch have electron lone pairs in their oxygen-containing functional groups. These electron lone pairs are very crucial for ionic transportation as they form dative bond with each other (Dannoun et al., Citation2022). Protonic (H+) sources must be doped into methylcellulose-potato starch blend to form polymer electrolyte. In this case, H+ can be obtained either from inorganic acids or ammonium salt. The former, however, can cause chemical degradation that leads to incompatibility with practical applications. Lithium salt is the best choice to secure optimum conductivity of polymer electrolyte, but upon considering its thermal instability, the less reactive ammonium is the more environmentally friendly and sustainable option. Ammonium iodide, NH4I are chosen in this work due to its low lattice energy, ΔU of 637 kJ/mol (Jenkins & Morris, Citation1976). Other ammonium salts also can be used, e.g. ammonium bromide (NH4Br, ΔU = 682.0 kJ/mol), ammonium acetate (NH4CH3CO2, ΔU = 703.1 kJ/mol), ammonium fluoride (NH4F, ΔU = 834.0 kJ/mol), ammonium sulfate (NH4)2SO4, ΔU = 1754.7 kJ/mol) and ammonium phosphate ((NH4)3PO4, ΔU = 3334.0 kJ/mol).
In this work, methylcellulose-potato starch blend, NH4I and glycerol are chosen as the polymer host, ion provider and plasticizing agent, respectively. The polymer electrolyte will be used in the preparation of the EDLC. The electrodes of the EDLC are prepared with microbial cellulose as the binder and CNT as the active material for charge double-layer process. Past EDLC has metal foil and non-biodegradable binder, e.g. PVdF in their electrodes. Our EDLC eliminates both metal foil and binder with unique structure of microbial cellulose.
2. Experimental methods
2.1. Materials/chemicals details
Potato starch (quality: 200), glycerol (≥99.5%), NH4I (≥99.0%) and methylcellulose (viscosity of 3,500–5,600 cP, 2% in water (20 °C)) were purchased from Merck. Hydroxyl functionalized multiwalled carbon nanotubes (MWCNT-OH, ≥88.0%) and sodium dodecyl sulfate were procured from SYSTERM while glacial acetic acid was from Friendemmann Schmidt. Source of cellulose is a type of microbial cellulose (BC) hydrogel obtained from local market (Malaysia).
2.2. Material synthesis
2.2.1. Preparation of polymer electrolytes
An amount of 0.4 g of potato starch was dissolved in 100 mL of 1% acetic acid at 80 °C. Methylcellulose (0.6 g) was poured into the potato starch solution that was cooled down to room temperature and stirred until a homogeneous solution was obtained. Next, 0.43 g of NH4I was included into the potato starch-methylcellulose solution. To plasticize the film, 0.29 g of glycerol was added. The solution was casted in Petri dishes and left to dry in a drying cabinet at room temperature.
2.2.2. Preparation of microbial cellulose-based electrodes
The green electrodes were prepared using water only and without the need for high temperatures. A total of 3 g of microbial cellulose was filtered using manual filtration. Then, an amount of 0.05 mg of MWCNT-OH was stirred in 20 mL of deionized water. shows FESEM images of microbial cellulose and MWCNT-OH. Microbial cellulose and MWCNT-OH solution were then mixed together with deionized water for one day at 45 °C. The homogenous solution was vacuum filtered through a Buchner funnel. Lastly, the microbial cellulose-MWCNT-OH electrodes were stripped off and dried at room temperature. Detailed information on this electrode has been described in our previous publication (Hamsan et al., Citation2022). In short, the interconnected nanofibrils microbial cellulose-MWCNT-OH network form highly conductive and pliable structure with minimum thickness of 52 µm.
2.3. Characterizations
The conductivity, of the polymer electrolyte was studied using HIOKI 3532–50 LCR. The thickness of potato starch-methycellulose-NH4I-glycerol film, x, was measured using Mitutoyo micrometer screw gauge. Impedance measurement was carried out in a frequency range from 50 Hz to 5 MHz. The polymer electrolyte film was placed in a Teflon holder with two stainless steel electrodes. The
was calculated using the following equation:
(1)
(1)
where Rb stands for the bulk resistance of the electrolyte and A is cross-sectional area of electrodes. Transference number analysis (TNM) was performed using V&A instrument DP3003 digital DC power supply that was connected to a multimeter and to the polymer electrolyte film. The polymer electrolyte film was subjected to a working voltage of 0.2 V. Ion transference number, tionic and electron transference number, telec were calculated using the given equations:
(2)
(2)
(3)
(3)
where the beginning and constant current were set as Ib and Ic, respectively. The electrochemical steadiness of the polymer electrolyte film was determined using Digi-IVY DY2300 potentiostat via linear sweep voltammetry (LSV) measurement. The polymer electrolyte film was subjected to a linear voltage up to 3 V at a sweeping rate of 1 mV/s.
2.4. EDLC device fabrication
Microbial cellulose-MWCNT-OH composite electrodes were cut into an area of 2.01 cm2. The glycerolized polymer electrolyte was placed in between two identical microbial cellulose-MWCNT-OH electrodes to form a simple EDLC. The EDLC was packed in CR2032 coin cell which then pressed using a battery clamper.
2.5. Characterization of the EDLC
Cyclic voltammetry (CV) analysis was employed to verify the capacitive behaviour of the EDLC. The coin cell was packed in a Teflon holder and connected to a potentiostat (Digi-IVY DY2300). The sweeping rate of the CV analysis was measured from 10 to 100 mV/s. The specific capacitance from CV, Ccv of the constructed EDLC was calculated using following equation:
(4)
(4)
where Vf and Vi are the last and beginning voltages, respectively. I(V) represent the area of CV plot which was extracted using OriginPro 9.0 software. The mass of MWCNT-OH is set as m while x is the sweeping rate. The charge-discharge measurements were analyzed with the current density of 0.75 mA cm−2 using a battery cycler (NEWARE). Important parameters, e.g. Ccd, equivalent series resistance (ESR), power density (Pden) and energy density (Eden) and efficiency were obtained via these following equations:
(5)
(5)
(6)
(6)
(7)
(7)
(8)
(8)
(9)
(9)
where y is the gradient of discharge curve, Vd is the potential drop and i is the applied current (1.5 mA).
3. Results and discussion
3.1. Conductivity analysis
σ is the heart of polymer electrolyte study. It is very crucial for an electrolyte to have a high value of σ, for it to be useful enough for energy storage application. shows the room temperature σ of the polymer electrolyte system. The film with potato starch and methylcellulose possesses a very low σ of 10−11 S/cm. The low value of σ is due to the low ionic density in the polymer matrix. There is a small amount of H+ in potato starch-methylcellulose film due to the presence of 1 wt.% acetic acid (CH3COOH). The inclusion of 30 wt.% NH4I increases the conductivity by up to six orders. One of the hydrogens in NH4+ is loosely bound and can be used for ionic conduction in the presence of electric field (Maryam & Abdullah, Citation2020). The σ is optimized at (1.47 ± 0.10) × 10−3 S/cm with the existent of plasticizer, which in this case is glycerol. There are several reasons why addition of glycerol improves the σ; (i) due to its high dielectric constant (46.5), more ions can be dissociated into mobile ions and (ii) molecules of glycerol are smaller than polymer molecules (Navaratnam, Rahman, Idris, & Abidin, Citation2020). This situation enables the plasticizer molecules to travel in between polymer chains which reduces the cohesive forces that hold the polymer structure, resulting in easier ionic conduction (Kato, Hiraoka, & Seki, Citation2020; Sekhar et al., Citation2012).
Table 1. Polymer electrolyte with their respectively σ at room temperature.
3.2. TNM analysis
Typical energy storage mechanism of an EDLC is via charge double-layer. Thus, it requires a polymer electrolyte with high tionic for an efficient polarization process. TNM measurement is performed with a working voltage of 0.2 V at room temperature. depicted the polarization plot of potato starch-methylcellulose-NH4I-glycerol electrolyte. A high current of 6.9 µA can be observed at the beginning of plot. During this stage, ions in the bulk of the electrolyte instantly migrate towards the stainless electrodes and start to form electrostatic force with the electrons in the electrode. After a while, the current reduces and becomes constant. The stable and low current value signifies the polarization process is completed and only electrons flow into the electrode. Ions are verified as the main charge carrier as the calculated tionic and telec are 0.99 and 0.01, respectively. The outcome is comparable with other protonic-based studies. A glycerolized polymer blend of starch and chitosan with NH4Cl as the ion source is reported to achieve tionic ranging from 0.91 to 0.98 (Shukur & Kadir, Citation2015). As reported by Vijaya et al., the tionic for polyvinylpyrrolidone (PVP)-NH4Cl based electrolytes is 0.93–0.97 (Vijaya et al., Citation2013). NH4Cl has higher lattice energy than NH4I which is 708 kJ/mol. According to Yusof, NH4I based polymer electrolyte with σ of ∼ 10−3 S cm−1 has tionic of 0.99 (Yusof, Citation2017).
3.3. LSV analysis
Apart from high σ and tionic values, a polymer electrolyte must be electrochemically stable in the potential range depending on the types of applications. The electrochemical stability of the polymer electrolyte can be determined using LSV measurement. In this analysis, the potential is swept linearly, and the subsequent current response is monitored. shows the LSV plot of potato starch-methycellulose-NH4I-glycerol electrolyte. When the electrolyte is subjected to a voltage at a sweeping rate of 1 mV/s, it can be observed that there is absence in current response at potential below 2.0 V. The constant current is related with the capacitive current as ions-electrons double layer formed on the contact surface area between the electrodes and the electrolytes. The plot also verified that the starch-methycellulose-NH4I-glycerol electrolyte has degraded after the voltage surpasses 2.4 V. The sharp increase in current beyond that threshold value is attributed to the oxidation of oxide and other functional groups in the polymer chains which causing instability in current flow. From this outcome, it is surmised that potato starch-methycellulose-NH4I-glycerol electrolyte can be used in any suitable electrochemical application with voltage lower than 2.4 V.
3.4. CV measurement of the microbial cellulose-based EDLC
The verification of charge double layer in the fabricated EDLC with the cell arrangement of microbial cellulose-electrode | potato starch-methycellulose-NH4I-glycerol electrolyte | microbial cellulose-electrode is done via CV measurements. A capacitor is a scan rate dependent device hence different scan rates are applied to the EDLC ranging from 10 to 100 mV/s. The form of CV plot in varies from rectangular and leaf-like shapes with absence of large redox peaks. According to Jäckel et al., a peak in CV curve indicates the existence of Faradaic current corresponds to the intercalation/deintercalation mechanism which does not occur in a double-layer capacitor (Jäckel et al., Citation2016). It is noticeable that the form of CV plot at high scan rate is a leaf-like shape rather than a perfect rectangular. At high scan rate, the conduction of mobile ions occurs at rapid rate causing the recombination of ions or improve buildup of charge double-layer. Furthermore, this is due to the internal resistance as well as the porosity of the carbon which creates current dependence of voltage (Liew, Ramesh, & Arof, Citation2014). This explains the low value of Ccv (2.9 F/g) in , when 100 mV/s is applied. A high value of Ccv (4.4 F/g) is obtained when 5 mV/s is used. During slow scan rate, nearly perfect plateau region is observed in the CV plot of 5 mV/s which signifies that the free ions move at a constant rate and hence developing the accumulation of ions at the electrode’s surface with low ohmic resistance (Fattah et al., Citation2016; Pal et al., Citation2019).
3.5. Charge-discharge measurement of the microbial cellulose-based EDLC
shows the charge-discharge curve of the constructed EDLC at selected cycles. The EDLC is charged up to 2 V and discharged down to 0 V. A typical capacitor will show a triangle plot due to electrostatic force between ions of the electrolyte and electrons of the electrodes. This is unlike conventional batteries where the discharge curve is non-linear due to the process of inter-and de-intercalation. illustrates the Ccd and efficiency as a function of cycle number for the constructed microbial cellulose-based EDLC at 0.75 mA/cm2. The Ccd at the first cycle is found to be 3.7 F/g where this value is harmonized with the one in CV analysis. Ccd value decreases to 3.3 F/g at the 50th cycle, followed by almost consistent value after the 100th cycle upwards and achieved an average value of 3.0 F/g as 1000 cycles are completed. The decrement of Ccd at the beginning stage could be due to several pores of the electrodes could be permanently congested or blocked after several charge-discharge processes or formation of ion aggregates and pairs (Suleman et al., Citation2016).
Figure 5. Charge-discharge plot of the fabricated microbial cellulose-based EDLC at selected cycles.

Figure 6. Ccv and efficiency values of the microbial cellulose-based EDLC throughout 1000 charge-discharge cycles.

The large value of efficiency indicates that the discharging part is almost equal or longer than the charging time. The low efficiency of 88.4% is noticeable at the first cycle. Usually, the charging process lasts longer compared to discharging in the first cycle before stabilization can be obtained. By continuously applying current, anions and cations start to approach the electrodes and become familiar with the conduction pattern. The efficiency varied in the range of 90–97% beyond the 100th cycle. This outcome of the current work discloses that the EDLC has excellent cycling stability.
The ESR is another important parameter to understand the electrolyte-electrodes contact along with internal resistance of the EDLC. As seen in , there is a small voltage drop (Vd) before the discharging process kicks in. The average voltage drops of the EDLC ∼ 0.0997 V. shows the ESR values of the EDLC throughout the 1000 complete charge-discharge cycles. The average ESR value is 66 Ω. The existence of ESR is attributed to the components of the EDLC, e.g. polymer electrolyte and the space between electrodes and the electrolyte (Wojciechowski, Kolanowski, Bund, & Lota, Citation2017). Small values of ESR verify that microbial cellulose-electrodes has good contact with the polymer electrolytes, facilitating charge carrier conduction towards the surface of the electrodes, therefore creating an efficient electrical double-layer for via electrostatic force (Latif et al., Citation2022).
depicts the variation of Eden and Pden over 1000 cycles. The stored energy for the first cycle is calculated to be 1.84 Wh/kg and reduced to 1.61 Wh/kg at 100th cycle. As the number of cycles increased, Eden started to stabilize at an average value of 1.52 Wh/kg lasting throughout the 1000 charge-discharge cycles. The stabilization of Eden signifies that NH4+ or H+ and I- in the polymer matrix of potato starch-methylcellulose blend travel toward the electrodes with almost similar energy barrier (Aziz et al., Citation2021). According to Winie et al., a biopolymer-based EDLC has Eden from 0.57 to 2.8 Wh/kg as the current density changes from 2 to 0.6 mA/cm2, respectively (Winie, Jamal, Saaid, & Tseng, Citation2018). The stability observed in Eden and Ccd trends indicate the low occurrence of ionic aggregation. Based on EquationEquation (8)(8)
(8) , Pden of the EDLC is highly correlated with ESR indicated its charge transfer is linked to the electrode-electrolyte gap (Yassine & Fabris, Citation2017). Pden value at the first cycle is found to be 226 W/kg and found to fluctuate in a small range. The average Pden of the EDLC is 225 W/kg.
4. Conclusions
A metal-free EDLC based on microbial cellulose-based electrodes and nature-derived polymer electrolyte was successfully fabricated and characterized. NH4I protonic source and glycerol plasticization agent had significant roles in GPE conductivity analysis. The prepared potato starch-methycellulose-NH4I-glycerol has conductivity, ion transference number and oxidation voltage of (1.47 ± 0.10) × 10−3 S/cm, 0.99 and 2.54 V, respectively, making it beneficial for the EDLC application. From CV measurement, no obvious redox peaks were observed indicating capacitive behavior and the EDLC was found to be scan rate dependent where the Ccd varied from 4.4 to 3.0 F/g as the EDLC was swept with 5 to 100 mV/s. The charge-discharge measurement revealed that the microbial cellulose-based EDLC has specific capacitance, ESR, Eden and Pden of 3.7 F/g, 66 Ω, 1.61 Wh/kg and 226 W/kg, respectively. The EDLC showed a high cycling ability for 1000 complete charge discharge cycles. Despite these findings supporting good compatibility between the microbial cellulose-MWCNT-OH electrodes and the GPE, the EDLC performance can be further enhanced by improving the tight contact between the electrodes and the electrolytes such as by inclusions of nano-filler, polymer grafting, using different types for carbon, salt, and polymers. The total performance of the green-based EDLC in this work has proven that it is appropriate for low voltage applications.
Acknowledgements
We sincerely thanked Centre for Defence Foundation Studies, UPNM and Faculty of Science, Universiti Malaya for all characterization facilities.
Disclosure statement
No potential conflict of interest was reported by the author(s).
Additional information
Funding
References
- Aziz, S. B., Dannoun, E. M. A., Hamsan, M. H., Ghareeb, H. O., Nofal, M. M., Karim, W. O., … Kadir, M. F. Z. A. (2021). A polymer blend electrolyte based on cs with enhanced ion transport and electrochemical properties for electrical double layer capacitor applications. Polymers, 13(6), 930. doi:10.3390/polym13060930
- Cazón, P., Puertas, G., & Vázquez, M. (2022). Production and characterization of active bacterial cellulose films obtained from the fermentation of wine bagasse and discarded potatoes by Komagateibacter xylinus. Polymers, 14(23), 5194. doi:10.3390/polym14235194
- Dannoun, E. M. A., Aziz, S. B., Brza, M. A., Al-Saeedi, S. I., Nofal, M. M., Mishra, K., … Hadi, J. M. (2022). Electrochemical and ion transport studies of li + ion-conducting mc-based biopolymer blend electrolytes. International Journal of Molecular Sciences, 23(16), 9152. doi:10.3390/ijms23169152
- Deng, L., Young, R. J., Kinloch, I. A., Abdelkader, A. M., Holmes, S. M., Rio, D. A. D. H., & Eichhorn, S. J. (2013). Supercapacitance from cellulose and carbon nanotube nanocomposite fibers. ACS Applied Materials & Interfaces, 5(20), 9983–9990. doi:10.1021/am403622v
- Fattah, N. F. A., Ng, H. M., Mahipal, Y. K., Numan, A., Ramesh, S., & Ramesh, K. (2016). An approach to solid-state electrical double layer capacitors fabricated with graphene oxide-doped, ionic liquid-based solid copolymer electrolytes. Materials (Basel, Switzerland), 9(6), 450. doi:10.3390/ma9060450
- Firdousie, N., Ahmed, I., Hussain, I., Nath, S., & Bhutia, R. N. (2022). Impact of Covid-19 lockdown on the fisheries sector of India. Food and Scientific Reports, 2, 43.
- Fu, J., Alee, M., Yang, M., Liu, H., Li, Y., Li, Z., & Yu, L. (2022). Synergizing multi-plasticizers for a starch-based edible film. Foods (Basel, Switzerland), 11(20), 3254. doi:10.3390/foods11203254
- Hamsan, M. H., Halim, N. A., Demon, S. Z. N., Sa’aya, N. S. N., Kadir, M. F. Z., Abidin, Z. H. Z., … Nor, N. M. (2022). Multifunction web-like polymeric network bacterial cellulose derived from SCOBY as both electrodes and electrolytes for pliable and low-cost supercapacitor. Polymers, 14(15), e11048. doi:10.3390/polym14153196
- Haque, F., & Fan, C. (2022). Prospect of microplastic pollution control under the “New normal” concept beyond COVID-19 pandemic. Journal of Cleaner Production, 367, 133027. doi:10.1016/j.jclepro.2022.133027
- Jäckel, N., Weingarth, D., Schreiber, A., Krüner, B., Zeiger, M., Tolosa, A., … Presser, V. (2016). Performance evaluation of conductive additives for activated carbon supercapacitors in organic electrolyte. Electrochimica Acta, 191, 284–298. doi:10.1016/j.electacta.2016.01.065
- Jenkins, H. D. B., & Morris, D. F. C. (1976). A new estimation of the lattice energies of the ammonium halides and the proton affinity of gaseous ammonia. Molecular Physics, 32(1), 231–236. doi:10.1080/00268977600101741
- Jordá-Reolid, M., Ibáñez-García, A., Catani, L., & Martínez-García, A. (2022). Development of blends to improve flexibility of biodegradable polymers. Polymers, 14(23), 5223. doi:10.3390/polym14235223
- Joseph, N., Shafi, P. M., Bose, A. C., Joseph, N., Shafi, P. M., & Bose, A. C. (2020). Recent advances in 2D-MoS2 and its composite nanostructures for supercapacitor electrode application. Energy & Fuels, 34(6), 6558–6597. doi:10.1021/acs.energyfuels.0c00430
- Kato, M., Hiraoka, K., & Seki, S. (2020). Investigation of the ionic conduction mechanism of polyether/Li7La3Zr2O12 composite solid electrolytes by electrochemical impedance spectroscopy. Journal of the Electrochemical Society, 167(7), 070559. doi:10.1149/1945-7111/ab8478
- Landi, G., Notte, L. L., Palma, A. L., Sorrentino, A., Maglione, M. G., & Puglisi, G. A. (2021). A comparative evaluation of sustainable binders for environmentally friendly carbon-based supercapacitors. Nanomaterials (Basel, Switzerland), 12(1), 46. doi:10.3390/nano12010046
- Latif, F. A., Zailani, N. A. M., Al Shukaili, Z. S. M., Zamri, S. F. M., Kasim, N. A. M., Rani, M. S. A., & Norrrahim, M. N. F. (2022). Review of poly (methyl methacrylate) based polymer electrolytes in solid-state supercapacitors. International Journal of Electrochemical Science, 17(1), 22013. doi:10.20964/2022.01.44
- Li, X., Tang, Y., Song, J., Yang, W., Wang, M., Zhu, C., … Lin, Y. (2018). Self-supporting activated carbon/carbon nanotube/reduced graphene oxide flexible electrode for high performance supercapacitor. Carbon, 129, 236–244. doi:10.1016/j.carbon.2017.11.099
- Liew, C. W., Ramesh, S., & Arof, A. K. (2014). Investigation of ionic liquid-based poly(vinyl alcohol) proton conductor for electrochemical double-layer capacitor. High Performance Polymers, 26(6), 632–636. doi:10.1177/0954008314536212
- Maryam, A. M., & Abdullah, O. G. (2020). Effect of high ammonium salt concentration and temperature on the structure, morphology, and ionic conductivity of proton-conductor solid polymer electrolytes based PVA. Membranes, 10(10), 262. doi:10.3390/membranes10100262
- Navaratnam, S., Rahman, N. A. A., Idris, N. A., & Abidin, S. Z. Z. (2020). The effect of glycerol on Na + ion conductivity and dielectric properties of potato starch-chitosan blend biopolymer electrolyte. International Journal of Electroactive Materials, 8, 10.
- Pal, B., Yang, S., Ramesh, S., Thangadurai, V., & Jose, R. (2019). Electrolyte selection for supercapacitive devices: A critical review. Nanoscale Advances, 1(10), 3807–3835. doi:10.1039/c9na00374f
- Pan, Z., Yang, J., Zhang, Y., Gao, X., & Wang, J. J. (2020). Quasi-solid-state fiber-shaped aqueous energy storage devices: Recent advances and prospects. Journal of Materials Chemistry A, 8(14), 6406–6433. doi:10.1039/C9TA13887K
- Gautham Prasad, G., Shetty, N., Thakur, S., Bommegowda, K. B., & Rakshitha. (2019). Supercapacitor technology and its applications: A review. IOP Conference Series: Materials Science and Engineering, 561(1), 012105. doi:10.1088/1757-899X/561/1/012105
- Qadir, R., & Gulshan, F. (2018). Reclamation of lithium cobalt oxide from waste lithium ion batteries to be used as recycled active cathode materials. Materials Sciences and Applications, 09(01), 142–154. doi:10.4236/msa.2018.91010
- Sa’aya, N. S. N., Demon, S. Z. N., Abdullah, N., Azmi, A. F. M., & Halim, N. A. (2023). Amperometric study of P3HT/multi-walled carbon nanotubes composite for malathion sensing. In: Maleque, M.A., Ahmad Azhar, A.Z., Sarifuddin, N., Syed Shaharuddin, S.I., Mohd Ali, A., Abdul Halim, N.F.H. (Eds.) Proceeding of 5th International Conference on Advances in Manufacturing and Materials Engineering. Lecture Notes in Mechanical Engineering. Singapore: Springer. doi:10.1007/978-981-19-9509-5_9
- Sahin, M. E., Blaabjerg, F., & Sangwongwanich, A. (2021). Modelling of supercapacitors based on simplified equivalent circuit. CPSS Transactions on Power Electronics and Applications (CPSS TPEA), 6, 31–39.
- Sekhar, P. C., Kumar, P. N., Sasikala, U., Rao, V. V. R. N., & Sharma, A. K. (2012). Investigations on lithium ion complexed polyvinyl alcohol (PVA) solid polymer electrolyte films. IRACST-IRACST-Engineering Science and Technology: An International Journal (ESTIJ), 2, 908–912.
- Shukur, M. F., & Kadir, M. F. Z. (2015). Hydrogen ion conducting starch-chitosan blend based electrolyte for application in electrochemical devices. Electrochimica Acta, 158, 152–165. doi:10.1016/j.electacta.2015.01.167
- Silva, R. R. A., Marques, C. S., Arruda, T. R., Teixeira, S. C., de Oliveira, T. V., Stringheta, P. C., … de Fátima Ferreira Soares, N. (2022). Ionic strength of methylcellulose-based films: An alternative for modulating mechanical performance and hydrophobicity for potential food packaging application. Polysaccharides, 3(2), 426–440. doi:10.3390/polysaccharides3020026
- Sohaimy, M. I. H., & Isa, M. I. N. (2022). Proton-conducting biopolymer electrolytes based on carboxymethyl cellulose doped with ammonium formate. Polymers, 14(15), 3019. doi:10.3390/polym14153019
- Suleman, M., Deraman, M., Othman, M. A. R., Omar, R., Hashim, M. A., Basri, N. H., … Hamdan, E. (2016). Electric double-layer capacitors with tea waste derived activated carbon electrodes and plastic crystal based flexible gel polymer electrolytes. Journal of Physics: Conference Series, 739, 012086. doi:10.1088/1742-6596/739/1/012086
- Tiwari, A. P., Mukhiya, T., Muthurasu, A., Chhetri, K., Lee, M., Dahal, B., … Kim, H. Y. (2021). A review of electrospun carbon nanofiber-based negative electrode materials for supercapacitors. Electrochem, 2(2), 236–250. doi:10.3390/electrochem2020017
- Tshiani, C. T., & Umenne, P. (2022). The characterization of the electric double-layer capacitor (EDLC) using Python/MATLAB/Simulink (PMS)-hybrid model. Energies, 15(14), 5193. doi:10.3390/en15145193
- Vijaya, N., Selvasekarapandian, S., Malathi, J., Iwai, Y., Nithya, H., & Kawamura, J. (2013). 1H NMR study on PVP-NH4Cl based-proton conducting polymer electrolyte. Indian Journal of Applied Research, 3, 506. doi:10.15373/2249555X/DEC2013/154
- Winie, T., Jamal, A., Saaid, F. I., & Tseng, T.-Y. (2018). Hexanoyl chitosan/ENR25 blend polymer electrolyte system for electrical double layer capacitor. Polymers Advanced Technologies, 30, 1. doi:10.1002/pat.4510
- Wojciechowski, J., Kolanowski, Ł., Bund, Ł. A., & Lota, G. (2017). The influence of current collector corrosion on the performance of electrochemical capacitors. Journal of Power Sources, 368, 18–29. doi:10.1016/j.jpowsour.2017.09.069
- Wu, D., & Zhong, W. J. (2019). A new strategy for anchoring a functionalized graphene hydrogel in a carbon cloth network to support a lignosulfonate/polyaniline hydrogel as an integrated electrode for flexible high areal-capacitance supercapacitors. Journal of Materials Chemistry A, 7(10), 5819–5830. doi:10.1039/C8TA11153G
- Yang, R., Chang, Y., Yang, X., Dai, J., Chen, Y., Chang, W., & Xiong, W. (2021). Electromechanical sorting method for improving the sensitivity of micropyramid carbon nanotube film flexible force sensor. Composites Part B: Engineering, 217, 108818. doi:10.1016/j.compositesb.2021.108818
- Yassine, M., & Fabris, D. (2017). Performance of commercially available supercapacitors. Energies, 10(9), 1340. doi:10.3390/en10091340
- Yuan, Z., Nag, R., & Cummins, E. (2022). Human health concerns regarding microplastics in the aquatic environment – From marine to food systems. The Science of the Total Environment, 823(1016), 153730. doi:10.1016/j.scitotenv.2022.153730
- Yusof, Y. M. (2017). Characteristics of corn starch/chitosan blend green polymer electrolytes complexed with ammonium iodide and its application in energy devices [Doctoral thesis]. Universiti Malaya. http://studentsrepo.um.edu.my/7494/.