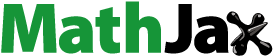
ABSTRACT
Herein, succinic acid functionalized Fe3O4@AMPA nanocomposite was synthesized as an effective adsorbent and used for the efficient removal of Cd(II) ions from aqueous media. Zeta potential, BET, TEM, TGA, and FTIR spectra were applied to characterize the Fe3O4@AMPA@SA nanocomposite. According to the BET analysis, the surface area of Fe3O4@AMPA@SA was found to be 43 m2/g. The influence of pH value, initial Cd(II) concentration, adsorbent dosage, temperature, and contact time on the adsorption process was achieved via the batch experiment method. The result showed that the optimal parameters were 6, 250 mg/L, 0.03 g/50 mL, 150 min, and 25°C, respectively. The experimental data fitted well to the Langmuir isotherm and the pseudo-first-order models. The maximum monolayer adsorption capacity of Cd(II) ions was 265 mg g−1. The adsorption mechanism of Cd(II) on magnetic nanocomposite is physical adsorption. The Fe3O4@AMPA@SA nanocomposite shows excellent reusability with a removal efficiency of 81.4% after three cycles.
GRAPHICAL ABSTRACT

1. Introduction
Cadmium (Cd(II)) is one of the most toxic heavy metals introduced into the environmental system via different industrial processes such as the chemical industry, battery manufacturing, mining and electroplating [Citation1]. Cd(II) is non-biodegradable and carcinogenic and it can damage the human immune system, kidneys, digestive system and nervous system, leading to hypertension, anemia, human hepatitis, cancer and other diseases [Citation2,Citation3]. Owing to the dangerous effects of Cd(II) on humans, the WHO has set the permissible limit of Cd(II) in drinking water is 5 μg/L [Citation4]. Therefore, the remediation of toxic Cd(II) ions from the environmental water before discharge into the environment is an important task. Therefore, it is essential to develop an effective and eco-friendly technology for remediation of toxic Cd(II) ions from the aqueous environment before discharging into the receiving water body.
There are many techniques available to eliminate heavy metal ions from wastewater, including chemical precipitation, ion exchange, electrochemical removal, solvent extraction, adsorption, etc. [Citation5–12]. Among these, adsorption is a unique and promising technique for the elimination of Cd(II) and other pollutants from an aqueous environment owing to its simplicity of design, environmentally friendly, low-cost, ease of recovery, high efficiency, easy operation and does not produce secondary pollutants in water. Many adsorbents have been applied for the adsorptive elimination of Cd(II) ions from wastewater under different operating conditions like CaO-SA [Citation13], NaOH-FA [Citation14], MgO-ATP [Citation15], MnS-fBC [Citation16], zeolite [Citation17], bentonite-fly ash [Citation18], industrial chili seeds (CS) [Citation19], biochars [Citation20]. Exploring the excellent adsorbent with easy separation of the analyte metal from the adsorbent surface and easy regeneration with high recovery efficiency would improve the adsorption process. Adsorbent materials based on magnetic nanoparticles have received extensive attention due to their magnetic properties and exhibit high removal capacity of pollutants and low toxicity [Citation21–23]. It can easily separate the pollutants from the aqueous solution via a magnet. Various adsorbent materials were coated magnetic nanoparticles to improve the adsorption process toward pollutant. Alqadami et al. prepared Fe3O4 @TSC nanocomposites for the removal of Cd(II) from an aqueous media [Citation1]. Elham Sadati Behbahani et al. synthesized Fe3O4/FeMoS4/MgAl-LDH adsorbent for removal of Cd(II), Pb(II) and Cu(II) ions [Citation24]. They found that the adsorption capacities of the adsorbent were 140.50, 190.75 and 110.25 mg g−1, respectively. Introducing various functional groups such as amino, carboxyl, hydroxyl, amide, and thiol will improve the adsorption process of pollutants. 2-Amino-3-mercaptopropionic acid (L-Cysteine) (AMPA) is a neutral organosulfur that consists of sulfhydryl, amino, and carboxyl groups on the side chain. These functional groups were used as chelate mercury (Hg (II)) [Citation24–26]. Succinic acid (SA) has a dicarboxylic group that serves as an adsorption active site for heavy metal ions [Citation27,Citation28]. Karnitz Jr. et al. introduced succinic anhydride in sugarcane bagasse using succinylation reaction for Cu(II), Pb(II), and Cd(II). They found that a good adsorption capacity for Cu(II), Pb(II), and Cd(II) [Citation27]. Therefore, the SA-modified surface of Fe3O4@AMPA could create a new magnetic nanocomposite for the removal of Cd(II) from aqueous media. To our knowledge, no work has been reported on the introduction of succinic acid to Fe3O4@AMPA.
This work aimed to synthesize a new magnetic nanocomposite (Fe3O4@AMPA@SA) by in situ co-precipitation and amidition methods. The Fe3O4@AMPA@SA adsorbent was applied for the elimination of toxic Cd(II) from an aqueous environment. The synthesized adsorbent was characterized using Zeta potential, BET surface area, TEM, FTIR and TGA analysis. The influence of efficient factors such as initial Cd(II) concentrations, adsorbent amount, solution pH, temperature, and contact time was investigated. The nonlinear isotherm and kinetic adsorption models were used to analyze experimental data. Thermodynamic parameters were also calculated. The mechanism and reusability of Cd(II) removal were studied.
2. Experiment
2.1. Chemicals and reagents
Succinic acid (SA) (C4H6O4), Dimethylformamide (DMF) was purchased from Panreac, UK 2-Amino-3-mercapto-propionic acid (AMPA) or (L-Cysteine, C3H7NO2S) (C3H7NO2S), Ferrous chloride tetrahydrate (FeCl2.4H2O), 1-ethyl-3-(3 dimethylaminopropyl) carbodiimide (EDC), and Ferric chloride hexahydrate (FeCl3.6H2O) were purchased from Sigma-Aldrich. NaOH, 25% NH4OH, and HCl were obtained from Merck, Germany. Cd(NO3)2 4H2O were purchased from BDH, England.
2.2. Synthesis of Fe3O4 @AMPA
Amino-3-mercaptopropionic (AMPA) modified Fe3O4 nanoparticles (Fe3O4@AMPA) were prepared by in situ co-precipitation method as follows: 10.81 g of FeCl3.6H2O and 3.98 g of FeCl2.4H2O were dissolved in beakers containing 100 ml of 0.01 M HCl under ultra-sonication and N2 gas, separately. Next, 1 g of AMPA was added in beaker containing 50 ml of D.I water under ultra-sonication for 3 h. Then the salt solution was transferred to a three-neck flask (500 ml). After that, the mixture was heated at 75°C under mechanical stirring and N2 gas for 10 min. Then, 35 mL of NH4OH solution was added into the mixture at the seam time with 50 mL of AMPA under mechanical stirring and N2 gas until it reached pH 10. Next, the mixture was kept under stirring N2 gas at room temperature for 2 h. The black precipitate was isolated from the solution by a magnet and washed several times with deionized water and EtOH. The black precipitate was dried overnight at 50°C ().
2.3. Synthesis of Fe3O4 @AMPA@SA nanocomposite
Fe3O4@AMPA@SA nanocomposite was synthesized in the following procedure: 1.15 g of succinic acid (SA) was dissolved in 30 mL DMF under ultra-sonication for 10 min. Then, 0.9 g of EDC was added to the solution of SA to activation of a carboxylic group under mechanical stirring for 30 min. After that, 1.5 g of Fe3O4@AMPA was added into the solution of SA. The reaction mixture was stirred vigorously under N2 gas at 25°C for 24 h. Finally, the Fe3O4@AMPA@SA was isolated via a magnet and then washed three times with DMF several times with D.I. water ().
2.4. Adsorption and desorption studies
The adsorption process of Cd(II) using Fe3O4@AMPA@SA nanocomposite in an aqueous solution was conducted in batch method at room temperature. The effect of different factors on the adsorption process, such as adsorbent dosage, pH solution, initial Cd(II) concentration, equilibrium time, and the temperature was accomplished in the range of (0.01–0.07 mg/L), (2.3–9), (25–350 mg/L), (5–240 min), and (25–45°C), respectively. In each experiment, a given load of Fe3O4@AMPA@SA (0.03 g/50 mL) was added to 50 mL of 25 mg/L Cd(II) aqueous solution; then, the sample was adjusted to pH = 7.4 using 0.1 HNO3 or NaOH. Next, the sample was shaken in a thermostatic water bath oscillator for 120 min at 25°C. after that, the sample was isolated via a magnet. Finally, the concentration of Cd(II) in the aqueous solution was quantified by AAS. By EquationEquations (1)(1)
(1) and (Equation2
(2)
(2) ) the adsorption capacity and percentage efficiency of Cd(II) ions were calculated, respectively.
where C0 and Ce (mg/L) are the concentration of Cd(II) at initial time, respectively; m (g) and V(L) are the mass of Fe3O4@AMPA@SA adsorbent and volume of Cd(II) solution, respectively.
For each adsorption-desorption cycle, 0.03 g Fe3O4@AMPA@SA was used for 50 mL of 25 mg/L Cd(II) solution and the sample was adjusted to pH = 7.4 using 0.1 HNO3 or NaOH. Then, the sample was shaken for 150 min at 25°C. After that the sample was separated with a magnet to collect the Cd(II) loaded Fe3O4@AMPA@SA and the concentration of Cd(II) in the aqueous solution was quantified by AAS. The attained Cd(II) loaded Fe3O4@AMPA@SA was rinsed with deionized water to remove unabsorbed traces of Cd(II) and then its added to conical flask containing 50 ml of 0.01 M HNO3 or 0.01 HCl solution, separately, shaking at 25°C for 150 min. The sample was separated with a magnet and the concentration of Cd(II) in the aqueous solution was quantified by AAS. The obtained Fe3O4@AMPA@SA nanocomposite was washed with deionized water and dried at 50°C for successive cycles. This cycle was repeated three times. The % desorption was calculated using EquationEquation 3(3)
(3)
where Cm (mg/L) and Ce (mg/L) refer to the Cd(II) concentrations released in the solution and the initially adsorbed Cd(II) concentration, respectively.
3. Results and discussion
3.1. Characterization of adsorbents
The TGA measurements of Fe3O4 nanoparticles and nanocomposite were performed using TGA: Mettler Toledo TGA/SDTA851, USA. The Zeta potential was measured at a range of 3–9 using Nano Plus Series, USA. The morphologies of the Fe3O4@AMPA@SA were analyzed using a transmission electron microscope (TEM, PHILIPS CM120). Fourier transform infrared spectroscopy (FTIR, Nicolet 6700, Thermo Scientific, USA) was used to determine the functional groups of Fe3O4@AMPA@SA, Fe3O4, SA, and Fe3O4@AMPA@SA//Cd(II). The BET surface area of Fe3O4@AMPA@SA nanocomposite was determined using nitrogen adsorption-desorption measurements. TEM images of Fe3O4@AMPA@SA nanocomposite are shown in ). It can be seen that the image of Fe3O4@AMPA@SA nanocomposite reveals spherical particles in shape with an average diameter of 12.6 nm as presented in ) inset. A grey layer at the edges of the magnetite nanoparticles may be assigned to organic compounds (AMPA and SA). To confirm the existence of sulfhydryl, amide, carboxyl groups on the surface of Fe3O4@AMPA@SA, FT-IR of the structure of Fe3O4@AMPA@SA, Fe3O4, SA, and Fe3O4@AMPA@SA//Cd(II) were tested was examined and as displayed in ). For magnetite nanoparticles, the characteristic peak of magnetite nanoparticles appeared at 572 cm−1 refer to the Fe-O bond. In the case of Fe3O4@AMPA@SA nanocomposite, the board peaks at 3439 cm−1 refers to the stretching vibration of the O-H/N-H group, the peaks at 2861 cm−1 and 2925 cm−1 is due to ν(C-H) stretching in AMPA and SA. The characteristic peak for Fe-O bond appeared at 572 cm−1, confirmed the formation of magnetic nanocomposite [Citation23], in addition, the characteristic peak for vibration stretching of S–H appeared at 2548 cm−1, indicates the presence of AMPA on the surface of magnetic nanocomposite [Citation29–31]. The weak peak at 1686 cm−1, a strong peak at 1635 cm−1, a weak peak at 1557 cm−1 may refer to the stretching of carbonyl of carboxylic, amide I, and amide II vibrations, respectively which are consistent with the literature [Citation32–34], indicated the formation of Fe3O4@AMPA@SA nanocomposite successfully. The peaks at 1517 cm−1, 1398 cm−1, (1322–1233 cm−1), and (1186–1090 cm−1), attributed to the stretching of N-H, COO−, C-N, C-O groups, respectively [Citation35,Citation36]. For SA, the ν(-C = O) of the stretch of COOH revealed at 1710 cm−1, ν(C–H), ν(O–H), and anti-symmetric (COO-) at around 2939 cm−1, 2648 cm−1, and 2529 cm−1, respectively [Citation37]. Remarkable alters are observed in the FTIR of spectrum Fe3O4@AMPA@SA nanocomposite after Cd(II) adsorption. A peak of sulfhydryl (S-H) at 2548 cm−1 was disappeared, indicated the bending of Cd(II) ion with S-H bond by electrostatic interaction on the surface of Fe3O4@AMPA@SA nanocomposite [Citation29]. In addition, the intensity of peak at1398 cm−1 was increased and shifted to 1395 cm−1 due to binding Cd(II) with COO- group on the surface of Fe3O4@AMPA@SA nanocomposite. The intensity of peaks in the range of 1322 − 1090 cm−1 was reduced and shifted to 1304–1080 cm−1 due to binding Cd(II) with C-N and C-O groups, respectively. The peaks of the O-H bond and NHCO− were shifted to 3418 and 1620 cm−1, respectively [Citation38]. Therefore, it is clearly that the Cd(II) ions are combined with functional groups onto Fe3O4@AMPA@SA nanocomposite
Figure 2. (a) TEM image of Fe3O4@AMPA@SA with size particles distribution (inset) and (b) FTIR spectrum of Fe3O4, SA, Fe3O4@AMPA@SA, and Fe3O4@AMPA@SA/Cd(II).

) exhibited thermal stability of the Fe3O4 and Fe3O4@AMPA@SA adsorbents. It was noticed that the magnetite nanoparticles and Fe3O4@AMPA@SA were degraded in two and three stages, respectively. In the first stage, 4% and 9% of initial weight loss at temperature up to 150°C for Fe3O4 and Fe3O4@AMPA@SA, respectively, which can be related to the elimination of adsorbed water and impurities [Citation39,Citation40]. In the second stage, a 5% of weight loss was observed in the range of 150°C to 750°C for pure magnetite owing to the conversion of some hydroxide to oxide [Citation41]. For the Fe3O4@AMPA@SA, 9% and 10% of weight loss from (150 to 250°C) and (250 to 800°C) were observed attributed to the decomposition of the organic structure of SA and AMPA [Citation29]. 9% and 28% are the total weight loss for Fe3O4 and Fe3O4@AMPA@SA nanocomposite, respectively, confirmed synthesized Fe3O4@AMPA@SA successfully.
Figure 3. (a) TGA analysis of Fe3O4 and Fe3O4@AMPA@SA, (b) N2 adsorption-desorption isotherms of Fe3O4@AMPA@SA nanocomposite, and (c) BJH pore-size distribution graph.

The mesoporous structure of the adsorbent material is critical for the fast and effective adsorption of heavy metals from aqueous solutions due to their large surface area, pore structure, and tunable pore sizes [Citation42]. Therefore, the heavy metal ions are adsorbed not only on the external surface area but also within the adsorbents’ mesoporous. ) displays the N2 adsorption-desorption analysis of Fe3O4@AMPA@SA nanocomposite which exhibited type IV isotherms. The BET surface area was calculated by using the Brunauer-Emmett-Teller (BET) method in the relative pressure (P/P0) range from 0.05 to 0.15 at 77 K in liquid nitrogen. The BET surface area of Fe3O4@AMPA@SA was found to be 43 m2/g. The values of C constant and correlation coefficient (R2) were 92.018 and 0.999921, respectively. The pore size distribution of Fe3O4@AMPA@SA was determined using the Barrett-Joyner-Halenda (BJH) method and found to be 12.3 nm as depicted in , which allows internal and external diffusion of Cd(II) by physical adsorption. The diameter of the Cd(II) ions is 0.15 nm [Citation42], enabling the pore-filling sorption mechanism. Thus, Cd(II) ions could get into the pore network of Fe3O4@AMPA@SA nanocomposite and promote pore-dependent adsorption by physical adsorption. Compared to the BET surface area of pure magnetite, the BET surface area is less than that reported in the literature [Citation43]. The decrease in surface area after functionalizing with AMPA and SA may be due to disruption in the aggregation after surface modification and also may be present a slight layer of SA and AMPA onto the magnetic surface. Therefore, the interaction between N2 molecules and the particle surface of Fe3O4@AMPA@SA nanocomposite is decreased. A similar result has been reported by Ghosh et al [Citation44]., Mohapatra and Pramanik [Citation45].
3.2. Adsorption performance of Fe3O4@AMPA@SA
3.2.1. Effect of pH and Zeta potential
The initial pH is a factor parameter affecting the adsorption performance behavior of adsorbents which influences the adsorbate ionization state and the surface charge of adsorbents, Therefore, the influence of initial pH on the adsorption process was tested at different pH (pH = 2.3–9) with at parameters fixed [Co: 25 mg/L, temperature: 25°C, dose: 0.03 g/50 mL, time: 24 h, and agitated: 100 rpm] as represented in ). It can be observed that the adsorption capacity of Fe3O4@AMPA@SA toward Cd(II) was increased from 4.6 to 40 mg g−1 as the solution pH value rising from 2.3 to 7, respectively. The maximum removal efficiency and adsorption capacity were 96% and 40 mg g−1, respectively at pH 7. Slightly decrease in the adsorbed Cd(II) was observed from (pH = 7–9). The removal efficiency was only 11.2% at strong acidic pH = 2.3 due to the competition between Cd(II) ions and hydronium ion (H3O+) for adsorption sites onto Fe3O4@AMPA@SA resulting in reduced the adsorption capacity of adsorbent and removal efficiency of Cd(II). After the further increase in pH from 2 to 7, a good removal efficiency was observed due to the increase of charge negatively on the surface of Fe3O4@AMPA@SA nanocomposite, resulting in higher adsorption capacity. The results of the Zeta potential test reveal that the isoelectric point (IEP) of the Fe3O4@AMPA@SA nanocomposite was 5, which means the Fe3O4@AMPA@SA nanocomposite was protonated at pHzpc<5 and the Cd(II) adsorption was reduced via the electrostatic repulsion ()). After pHzpc >5, the active sites of the Fe3O4@AMPA@SA surface became a negative charge, resulting in higher removal efficiency due to the electrostatic attraction between negative Fe3O4@AMPA@SA surface and positive Cd(II) ions. At a pH higher than 7, the Cd(II) ions suffer hydrolysis, reducing the adsorption process and forming hydroxide precipitates (Cd(OH)2) [Citation46]. Thus, the optimal pH = 7 was selected as optimal pH for all adsorption experiments.
3.2.2. Effect of adsorbent dosage
The influence of adsorbent dose on the removal efficiency of Cd(II) was studied in the range between 0.01 to 0.07 g/50 mL (T: 298 K; Co: 25 mg/L; pH: 7; agitation: 100 rpm) as presented in ). The elimination efficiency of Cd(II) was increased from nearly 48.64% to 95.54% as the adsorbent dose of the Fe3O4@AMPA@SA improving from 0.01 g to 0.07 g/50 mL, respectively owing to improve the active sites on the Fe3O4@AMPA@SA surface, which resulted in higher removal efficiency. Behind 0.03 g/50 mL, no change in removal efficiency was observed. On the other hand, the adsorption capacity of Fe3O4@AMPA@SA was reduced to 39.61 mg g−1 with improving the adsorbent to 0.03 g/50 mL due to the initial Cd(II) concentration is still constant in Equationequation (1)(1)
(1) with increasing adsorbent dose, leading to the reduction of adsorption capacity. Also, the reason for the decrease in adsorption capacity may be due to the aggregation of adsorption sites [Citation47]. A similar effect is achieved by Aldawsari et al [Citation35]. Therefore, 0.03 g/50 mL of Fe3O4@AMPA@SA was chosen as a proper dose for all adsorption experiments.
3.2.3. Effect of contact time
The effect of equilibrium time on the Cd(II) adsorption onto Fe3O4@AMPA@SA surface was performed by varying the time between 5 and 240 min [T: 298 K; Co: 25 mg/L; pH: 7; agitation: 100 rpm] as shown in ). The outcomes result was revealed that the adsorption capacity of Fe3O4@AMPA@SA and percentage adsorption of Cd(II) ion was increased gradually from (2.5 to 39.58 mg g−1) and (6% to 96%), respectively with rising equilibrium time from 5 min to 150 min and then remained constant. Fast adsorption was observed in the first 15 and 30 min with 50% and 74% removal, respectively due to the high availability of functional groups on the surface of Fe3O4@AMPA@SA nanocomposite which binding of Cd(II) ions easily. Therefore, 150 min was the desirable choice for all adsorption experiments.
3.2.4. Effect of initial MG concentration and temperatures
The effect of the initial Cd(II) concentration (25–350 mg/L) on adsorption capability at various temperatures was performed [Co: 25 mg/L; time: 150 min; pH: 7; agitation: 100 rpm] as shown in ). It’s clear that the adsorption capacities of Fe3O4@AMPA@SA were augmented from 37.5 to 250 mg g−1 as the initial Cd(II) concentration improving from 25 mg/L to 350 mg/L, respectively, which might be elucidated that the increasing Cd(II) concentration gradient acts as a driving force for the mass transfer of Cd(II) to Fe3O4@AMPA@SA surface and enhance the effective collision between the Cd(II) ions and the active sites onto Fe3O4@AMPA@SA surface which leading to the improve in adsorption capacity. A similar result has been reported by Xiaohui Zhou et al. [Citation48]. The adsorption capacity of Fe3O4@AMPA@SA adsorbent toward Cd(II) was reduced with a rise in the temperature from 25 to 45°C as displayed in ), which means that the adsorption of Cd(II) onto Fe3O4@AMPA@SA was exothermic.
3.3. Adsorption modeling
3.3.1. Isotherm studies
To evaluate the adsorption capacity of the Fe3O4@AMPA@SA toward Cd(II) adsorption, four nonlinear isotherm adsorption include Langmuir EquationEquation (4)(4)
(4) [Citation49], Freundlich EquationEquation (5)
(5)
(5) , Timken EquationEquation (6)
(6)
(6) and Dubinin–Radushkviech (D–R) EquationEquations 7
(7)
(7) –Equation9
(9)
(9) [Citation50] isotherm was applied.
where KL and KF, and KD-R represent the Langmuir, Freundlich and D–R isotherm constants; n is the Freundlich intensity parameter; E (kJ/mol) is the energy of adsorption; AT is the equilibrium binding constant and BT is the heat of adsorption (J/mol). summarizes the analysis of various parameters of isotherm models. The fitting curves are shown in ). Among the isotherm models, the Langmuir isotherm model can fit the data well and had a highest R2(0.98891) compared to other models Timken (0.9498), Freundlich (0.9104), and D–R (0.9000), indicating that the adsorption process of Cd(II) ions were monolayer adsorption with maximum qe (265 mg g−1) at 25°C. Compared to the recently reported magnetic nanocomposite for Cd(II) adsorption, the adsorption capacity of Fe3O4@AMPA@SA toward Cd(II) adsorption is the highest [Citation46,Citation48,Citation51–57](). Therefore, these results confirmed that the Fe3O4@AMPA@SA is a promising adsorbent for the effective removal of Cd(II) ions from contaminated water. From the D-R isotherm (), the values of E were in the range (0.0842–0.0614 kJ /mol) at various temperatures from 25°C to 45°C, indicating that physical adsorption was dominant in the adsorption process [Citation58,Citation59].
Figure 5. Nonlinear isotherm adsorption of Cd(II) by Fe3O4@AMPA@SA nanocomposite at various temperature (a) 25°C, (b) 35°C, and (c) 45°C, (d) Nonlinear kinetic adsorption of Cd(II) by Fe3O4@AMPA@SA nanocomposite.

Table 1. Isotherm parameters for Cd(II) adsorption on Fe3O4@AMPA@SA nanocomposite.
Table 4. Comparison of Cd(II) adsorption on various magnetic adsorbents.
3.3.2. Kinetics studies
To investigate the controlling mechanisms of Cd(II) adsorption on Fe3O4@AMPA@SA, nonlinear pseudo-first-order (PFO) EquationEquation (10)(10)
(10) , pseudo-second-order (PSO) EquationEquation (11)
(11)
(11) [Citation60] and Elovich kinetic models (EKM) EquationEquation (12)
(12)
(12) were applied [Citation61].
where k1 and k2 refer to the constants of adsorption rates PFO and PSO model, respectively; α is the constant (desorption) and represent surface coverage, and α is the initial adsorption rate. summarizes the analysis of various parameters of kinetic models. The fitting curves are presented in ). As shown in , the R2 of the PFO kinetic model (0.9625) were much better than those of PSO (0.9308) and EKM (0.875). The experimental (39.83 mg g−1) values are very close to the calculated qe. cal (39.35 mg g−1) by PFO kinetic model, indicating that the adsorption process was dominated by physisorption. This result is consistent with the literature reported by Pawar et al. for Pb(II), Cd(II) and As(V) adsorption using magnetic composite beads [Citation62].
Table 2. Kinetics parameters for Cd(II) adsorption on Fe3O4@AMPA@SA nanocomposite.
3.3.3. Thermodynamic of Cd(II) adsorption
To determine the thermodynamic properties of the Cd(II) adsorption onto Fe3O4@AMPA@SA, energy changes such as Gibbs free energy change (ΔG°) EquationEquation (13)(13)
(13) , enthalpy (ΔH°) EquationEquation (14)
(14)
(14) , and entropy change (ΔS°) was analyzed:
Where Kc is equal (qe/Ce) which refers to an equilibrium constant at various temperatures [Citation63]. The ΔHo and ΔSo were calculated from the plot of ln Kc versus 1/T. shows the thermodynamics factors for the Cd(II) adsorption on Fe3O4@AMPA@SA nanocomposite. The outcomes showed that at all temperatures the ΔGo values were negative, which depicted that the spontaneous and feasibility of the adsorption process. The values of ΔSo and ΔHo were negative at all temperatures, suggesting decreasing disorder of the interface during the adsorption process and the adsorption process was exothermic, respectively [Citation35].
Table 3. Thermodynamics factors for the Cd(II) adsorption on Fe3O4@AMPA@SA nanocomposite.
3.4. Desorption study
Recycling performance is an important index for evaluating an adsorbent. To achieve the reuse performance of Fe3O4@AMPA@SA nanocomposite, 0.01 M HNO3 and 0.01 M HCl solution were applied as eluents for recovery of Cd(II) from Fe3O4@AMPA@SA adsorbent. The outcomes revealed that the removal recovery of Cd(II) using 0.01 M HNO3 (91%) was better than HCl (79%). The reusability of Fe3O4@AMPA@SA nanocomposite was achieved using determining the percentage elimination of 25 mg/L of Cd(II) with recovered adsorbent in different cycles. After three recycle, the removal efficiency was reduced to 81.21%, indicating the excellent potential of Fe3O4@AMPA@SA nanocomposite as reusable adsorbent ()).
3.5. Adsorption mechanism
exhibits the mechanism of Cd(II) adsorption onto Fe3O4@AMPA@SA nanocomposite. It can be observed that a large number of S-H, -COOH, and -CONH functional groups incorporating on the surface of Fe3O4@AMPA@SA nanocomposite. Isotherm, kinetic adsorption, thermodynamic parameters and FTIR spectrum were used to evaluate the mechanism of Cd(II) adsorption. As shown in ), after Cd(II) adsorption onto Fe3O4@AMPA@SA nanocomposite, the intensity of peaks in the range (1322–1090 cm−1) was reduced and shifted to (1304 to 1080 cm−1) due to binding Cd(II) with C-N and C-O groups. The peaks of the O-H bond and -CONH were moved to 3418 cm−1 and 1620 cm−1, respectively. In addition, a peak of sulfhydryl (S-H) at 2548 cm−1 was disappeared, indicated the bending of Cd(II) ion with S-H bond by electrostatic interaction on the surface of Fe3O4@AMPA@SA nanocomposite [Citation29]. All these changes indicate the adsorption of Cd(II) onto the surface of Fe3O4@AMPA@SA nanocomposite [Citation38]. In addition, the isotherm, kinetic adsorption and thermodynamic parameters confirm the physical adsorption between the active site on magnetic adsorption and Cd(II) ions.
Conclusion
In this study, a new magnetic nanocomposite (Fe3O4@AMPA@SA) was synthesized for the elimination of toxic Cd(II) from aqueous media. The synthesized adsorbent was characterized using Zeta potential, FT-IR, TEM, TGA and BET surface area analysis. Based on the TEM image, the average particle size of Fe3O4@AMPA@SA nanocomposite was 12.6 nm. The adsorption of Cd(II) onto Fe3O4@AMPA@SA was performed using a batch method and the results reveal that the best optimized experimental conditions were dose: 0.03 g: contact time: 150 min; pH: 7; initial Cd(II) concentration: 250 mg/L; and temperature: 25°C. Kinetic and isotherm data exhibited a good correlation to pseudo-first-order and Langmuir with maximum monolayer adsorption capacity 265 mg g−1 at 25°C. Based on the D-R model, the activation energy (Ea) was found in the range (0.0842–0.0614 kJ /mol) at various temperatures, confirming the mechanism adsorption of Cd(II) on magnetic nanocomposite is physical adsorption. Based on thermodynamic results, the adsorption process was exothermic and spontaneous. Fe3O4@AMPA@SA nanocomposite shows excellent reusability with a removal efficiency of 81.4% after three cycles. In conclusion, the characteristic advantages of use Fe3O4@AMPA@SA nanocomposite show great potential for use as an effective adsorbent for the removal of toxic Cd(II)) from aqueous media.
Acknowledgments
The authors express their appreciation for the search and innovation agency, the Ministry of Education in Saudi Arabia to finance this research work through the project number (UB-27-1442).
Disclosure statement
No potential conflict of interest was reported by the author(s).
Additional information
Funding
References
- Alqadami, Naushad M, Ahamad T, et al. Removal of highly toxic Cd(II) metal ions from aqueous medium using magnetic nanocomposite: adsorption kinetics, isotherm and thermodynamics. Desalin Water Treat. 2020;181:355–13.
- Sobhanardakani S, Tayebi L, Hosseini SV. Health risk assessment of arsenic and heavy metals (Cd, Cu, Co, Pb, and Sn) through consumption of caviar of Acipenser persicus from Southern Caspian Sea. Environ Sci Pollut Res [Internet]. 2018;25(3):2664–2671.
- Saravanan A, Kumar PS, D-VN V, et al. Ultrasonic assisted agro waste biomass for rapid removal of Cd(II) ions from aquatic environment: mechanism and modelling analysis. Chemosphere [Internet]. 2021;271:129484. Available from:https://www.sciencedirect.com/science/article/pii/S0045653520336821
- Karunanayake AG, Todd OA, Crowley M, et al. Lead and cadmium remediation using magnetized and nonmagnetized biochar from Douglas fir. Chem Eng J. 2018;331:480–491.
- Zhu W, Li X, Wu D, et al. Synthesis of spherical mesoporous silica materials by pseudomorphic transformation of silica fume and its Pb2+ removal properties. Microporous Mesoporous Mater [Internet]. 2016;222:192–201. Available from: http://www.sciencedirect.com/science/article/pii/S1387181115005582
- Wang N, Xu X, Li H, et al. Preparation and application of a xanthate-modified thiourea chitosan sponge for the removal of Pb(II) from aqueous solutions. Ind Eng Chem Res [Internet]. 2016;55(17):4960–4968.
- Di Palma L, Ferrantelli P, Merli C, et al. Treatment of industrial landfill leachate by means of evaporation and reverse osmosis. Waste Manage [Internet]. 2002;22(8):951–955. Available from: http://www.sciencedirect.com/science/article/pii/S0956053X0200079X
- Ghasemi M, Naushad M, Ghasemi N, et al. Adsorption of Pb(II) from aqueous solution using new adsorbents prepared from agricultural waste: adsorption isotherm and kinetic studies. J Ind Eng Chem [Internet]. 2014;20:2193–2199. Available from: http://www.sciencedirect.com/science/article/pii/S1226086X1300470X.
- Matlock MM, Howerton BS, Atwood DA. Chemical precipitation of heavy metals from acid mine drainage. Water Res [Internet]. 2002;36:4757–4764. Available from: http://www.sciencedirect.com/science/article/pii/S0043135402001495
- Fu F, Wang Q. Removal of heavy metal ions from wastewaters: a review. J Environ Manage [Internet]. 2011;92:407–418. Available from: http://www.sciencedirect.com/science/article/pii/S0301479710004147
- Burke DM, Morris MA, Holmes JD. Chemical oxidation of mesoporous carbon foams for lead ion adsorption. Sep Purif Technol [Internet]. 2013;104:150–159. Available from: http://www.sciencedirect.com/science/article/pii/S1383586612005916
- Naushad M. Surfactant assisted nano-composite cation exchanger: development, characterization and applications for the removal of toxic Pb2+ from aqueous medium. Chem Eng J [Internet]. 2014;235:100–108. Available from: http://www.sciencedirect.com/science/article/pii/S1385894713011844
- Gu H, Lin W, Sun S, et al. Calcium oxide modification of activated sludge as a low-cost adsorbent: preparation and application in Cd(II) removal. Ecotoxicol Environ Saf [Internet]. 2021;209:111760. Available from: https://www.sciencedirect.com/science/article/pii/S0147651320315967
- Huang X, Zhao H, Zhang G, et al. Potential of removing Cd(II) and Pb(II) from contaminated water using a newly modified fly ash. Chemosphere Internet]. 2020;242:125148. Available from: http://www.sciencedirect.com/science/article/pii/S0045653519323872
- Huang R, Lin Q, Zhong Q, et al. Removal of Cd(II) and Pb(II) from aqueous solution by modified attapulgite clay. Arabian J Chem Internet]. 2020;13:4994–5008. Available from: http://www.sciencedirect.com/science/article/pii/S1878535220300344DOI.
- Fan Z, Zhang Q, Li M, et al. Removal behavior and mechanisms of Cd(II) by a novel MnS loaded functional biochar: influence of oxygenation. J Clean Prod [Internet]. 2020;256:120672. Available from: https://www.sciencedirect.com/science/article/pii/S0959652620307198
- He K, Chen Y, Tang Z, et al. Removal of heavy metal ions from aqueous solution by zeolite synthesized from fly ash. Environ Sci Pollut Res [Internet]. 2016;23(3):2778–2788.
- Mwamulima T, Zhang X, Wang Y, et al. Novel approach to control adsorbent aggregation: iron fixed bentonite-fly ash for Lead (Pb) and Cadmium (Cd) removal from aqueous media. Front Environ Sci Eng. 2018;12(2):1–12.
- Medellin-Castillo NA, Padilla-Ortega E, Regules-Martínez MC, et al. Single and competitive adsorption of Cd(II) and Pb(II) ions from aqueous solutions onto industrial chili seeds (Capsicum annuum) waste. Sustainable Environ Res [Internet]. 2017;27:61–69. Available from: https://www.sciencedirect.com/science/article/pii/S2468203916301704.
- Bandara T, Xu J, Potter ID, et al. Mechanisms for the removal of Cd(II) and Cu(II) from aqueous solution and mine water by biochars derived from agricultural wastes. Chemosphere [Internet]. 2020;254:126745. Available from: https://www.sciencedirect.com/science/article/pii/S0045653520309383
- Alqadami AA, Khan MA, Siddiqui MR, et al. Development of citric anhydride anchored mesoporous MOF through post synthesis modification to sequester potentially toxic lead (II) from water. Microporous Mesoporous Mater. 2018;261:198–206.
- Alqadami AA, Khan MA, Siddiqui MR, et al. A facile approach to develop industrial waste encapsulated cryogenic alginate beads to sequester toxic bivalent heavy metals. J King Saud Univ Sci. 2020;32(2):1444–1450.
- Alsuhybani M, Alshahrani A, Algamdi M, et al. Highly efficient removal of Pb(II) from aqueous systems using a new nanocomposite: adsorption, isotherm, kinetic and mechanism studies. J Mol Liq [Internet]. 2020;301:112393. Available from: http://www.sciencedirect.com/science/article/pii/S0167732219357149
- Behbahani ES, Dashtian K, Ghaedi M. Fe3O4-FeMoS4: promise magnetite LDH-based adsorbent for simultaneous removal of Pb (II), Cd (II), and Cu (II) heavy metal ions. J Hazard Mater [Internet]. 2021;410:124560. Available from: https://www.sciencedirect.com/science/article/pii/S0304389420325504
- Li W, Ju B, Zhang S. A green l-cysteine modified cellulose nanocrystals biosorbent for adsorption of mercury ions from aqueous solutions. RSC Adv. 2019;9(12):6986–6994.
- Bansal M, Ram B, Chauhan GS, et al. L-Cysteine functionalized bagasse cellulose nanofibers for mercury (II) ions adsorption. Int J Biol Macromol. 2018;112:728–736.
- Karnitz O, Gurgel LVA, de Melo JCP, et al. Adsorption of heavy metal ion from aqueous single metal solution by chemically modified sugarcane bagasse. Bioresour Technol [Internet]. 2007;98:1291–1297. Available from: https://www.sciencedirect.com/science/article/pii/S0960852406002100
- Gurgel LVA, Júnior OK, Gil Rp de F, et al. Adsorption of Cu(II), Cd(II), and Pb(II) from aqueous single metal solutions by cellulose and mercerized cellulose chemically modified with succinic anhydride. Bioresour Technol [Internet]. 2008;99:3077–3083. Available from: https://www.sciencedirect.com/science/article/pii/S0960852407005056
- Fan L, Deng M, Lin C, et al. A multifunctional composite Fe 3O4/MOF/l-cysteine for removal, magnetic solid phase extraction and fluorescence sensing of Cd(ii). RSC Adv [Internet]. 2018;8(19):10561–10572.
- Anirudhan TS, Manjusha V, Shainy F. Magnetically retrievable cysteine modified graphene oxide@nickelferrite@titanium dioxide photocatalyst for the effective degradation of chlorpyrifos from aqueous solutions. Environ Technol Innovation [Internet]. 2021;23:101633. Available from: https://www.sciencedirect.com/science/article/pii/S2352186421002819
- Devi S, Singh B, Paul AK, et al. Highly sensitive and selective detection of trinitrotoluene using cysteine-capped gold nanoparticles. Anal Methods [Internet]. 2016;8(22):4398–4405.
- Abd Razak NF, Shamsuddin M, Lee SL. Adsorption kinetics and thermodynamics studies of gold(III) ions using thioctic acid functionalized silica coated magnetite nanoparticles. Chem Eng Res Des [Internet]. 2018;130:18–28. Available from: https://www.sciencedirect.com/science/article/pii/S0263876217306688
- Verma S, Dutta RK. Development of cysteine amide reduced graphene oxide (CARGO) nano-adsorbents for enhanced uranyl ions removal from aqueous medium. J Environ Chem Eng [Internet]. 2017;5:4547–4558. Available from: https://www.sciencedirect.com/science/article/pii/S2213343717304347
- Abdelhalim AOE, V SV, Meshcheriakov AA, et al. Reduction and functionalization of graphene oxide with L-cysteine: synthesis, characterization and biocompatibility. Nanomedicine [Internet]. 2020;29:102284. Available from: https://www.sciencedirect.com/science/article/pii/S1549963420301386
- Aldawsari AM, Alsohaimi IH, Al-Kahtani AA, et al. Adsorptive performance of aminoterephthalic acid modified oxidized activated carbon for malachite green dye: mechanism, kinetic and thermodynamic studies. Sep Sci Technol [Internet]. 2021;56(5):835–846.
- Amiri A, Shanbedi M, Savari M, et al. Cadmium ion sorption from aqueous solutions by high surface area ethylenediaminetetraacetic acid- and diethylene triamine pentaacetic acid-treated carbon nanotubes. RSC Adv [Internet]. 2015;5(87):71144–71152.
- Krishnan S, Raj CJ, Robert R, et al. Growth and characterization of succinic acid single crystals. Cryst Res Technol [Internet]. 2007;42(11):1087–1090.
- Zaidi NAHM, Lim LBL, Usman A. Enhancing adsorption of malachite green dye using base-modified Artocarpus odoratissimus leaves as adsorbents. Environ Technol Innovation [Internet]. 2019;13:211–223. Available from: https://www.sciencedirect.com/science/article/pii/S2352186418302906
- Alqadami AA, Naushad M, ALOthman ZA, et al. Excellent adsorptive performance of a new nanocomposite for removal of toxic Pb(II) from aqueous environment: adsorption mechanism and modeling analysis. J Hazard Mater [Internet]. 2020;389:121896. Available from: http://www.sciencedirect.com/science/article/pii/S0304389419318503
- Viñambres M, Filice M, Marciello M. Modulation of the catalytic properties of lipase B from Candida antarctica by immobilization on tailor-made magnetic iron oxide nanoparticles: the key role of nanocarrier surface engineering. Polymers [Internet]. 2018;10:615. Available from: https://pubmed.ncbi.nlm.nih.gov/30966649
- Alqadami A, Naushad M, Abdalla MA, et al. Synthesis and characterization of Fe3O4@TSC nanocomposite: highly efficient removal of toxic metal ions from aqueous medium. RSC Adv [Internet]. 2016;6:22679–22689. Available from: http://pubs.rsc.org/en/content/articlehtml/2016/ra/c5ra27525c
- Hassan M, Liu Y, Naidu R, et al. Mesoporous biopolymer architecture enhanced the adsorption and selectivity of aqueous heavy-metal ions. ACS Omega [Internet]. 2021;6(23):15316–15331.
- Liu Y, Fu R, Sun Y, et al. Multifunctional nanocomposites Fe3O4@SiO2-EDTA for Pb(II) and Cu(II) removal from aqueous solutions. Appl Surf Sci Internet]. 2016;369:267–276. Available from: http://www.sciencedirect.com/science/article/pii/S0169433216302033
- Ghosh S, Badruddoza AZM, Uddin MS, et al. Adsorption of chiral aromatic amino acids onto carboxymethyl-β-cyclodextrin bonded Fe3O4/SiO2 core–shell nanoparticles. J Colloid Interface Sci [Internet]. 2011;354:483–492. Available from: https://www.sciencedirect.com/science/article/pii/S002197971001338X
- Mohapatra S, Pramanik P. Synthesis and stability of functionalized iron oxide nanoparticles using organophosphorus coupling agents. Colloids Surf A Physicochem Eng Asp [Internet]. 2009;339:35–42. Available from: https://www.sciencedirect.com/science/article/pii/S0927775709000417
- Bao S, Yang W, Wang Y, et al. One-pot synthesis of magnetic graphene oxide composites as an efficient and recoverable adsorbent for Cd(II) and Pb(II) removal from aqueous solution. J Hazard Mater [Internet]. 2020;381:120914. Available from: http://www.sciencedirect.com/science/article/pii/S0304389419308672
- Ghasemi M, Mashhadi S, Asif M, et al. Microwave-assisted synthesis of tetraethylenepentamine functionalized activated carbon with high adsorption capacity for Malachite green dye. J Mol Liq [Internet]. 2016;213:317–325. Available from: https://www.sciencedirect.com/science/article/pii/S0167732215303457
- Zhou X, Zhou J, Liu Y, et al. Preparation of iminodiacetic acid-modified magnetic biochar by carbonization, magnetization and functional modification for Cd(II) removal in water. Fuel [Internet]. 2018;233:469–479. Available from: https://www.sciencedirect.com/science/article/pii/S0016236118311281
- Wallis A, Dollard MF. Local and global factors in work stress - The Australian dairy farming examplar. Scand J Work Environ Health Suppl. 2008;(6):66–74.
- Dubinin LVR MM. Equation of the characteristic curve of activated charcoal. Proc Academy Sci USSR, Phys Chem Section. 1947;1:857.
- Fu W, Huang Z. Magnetic dithiocarbamate functionalized reduced graphene oxide for the removal of Cu(II), Cd(II), Pb(II), and Hg(II) ions from aqueous solution: synthesis, adsorption, and regeneration. Chemosphere [Internet]. 2018;209:449–456. Available from: http://www.sciencedirect.com/science/article/pii/S0045653518311627
- Metin AÜ, Doğan D, Can M. Novel magnetic gel beads based on ionically crosslinked sodium alginate and polyanetholesulfonic acid: synthesis and application for adsorption of cationic dyes. Mater Chem Phys [Internet]. 2020;256:123659. Available from: https://www.sciencedirect.com/science/article/pii/S0254058420310208
- Tang Y, Liang S, Wang J, et al. Amino-functionalized core-shell magnetic mesoporous composite microspheres for Pb(II) and Cd(II) removal. J Environ Sci [Internet]. 2013;25:830–837. Available from: http://www.sciencedirect.com/science/article/pii/S1001074212601417.
- Fu H, He H, Zhu R, et al. Phosphate modified magnetite@ferrihydrite as an magnetic adsorbent for Cd(II) removal from water, soil, and sediment. SciTotal Environ [Internet]. 2021;764:142846. Available from: https://www.sciencedirect.com/science/article/pii/S0048969720363762
- Liu F, Zhou K, Chen Q, et al. Preparation of magnetic ferrite by optimizing the synthetic pH and its application for the removal of Cd(II) from Cd-NH3-H2O system. J Mol Liq [Internet]. 2018;264:215–222. Available from: https://www.sciencedirect.com/science/article/pii/S0167732218316398
- Deng J-H, Zhang X-R, Zeng G-M, et al. Simultaneous removal of Cd(II) and ionic dyes from aqueous solution using magnetic graphene oxide nanocomposite as an adsorbent. Chem Eng J [Internet]. 2013;226:189–200.
- Luo X, Guo B, Wang L, et al. Synthesis of magnetic ion-imprinted fluorescent CdTe quantum dots by chemical etching and their visualization application for selective removal of Cd(II) from water. Colloids Surf A Physicochem Eng Asp [Internet]. 2014;462:186–193. Available from: https://www.sciencedirect.com/science/article/pii/S0927775714007274
- Dada AO, Adekola FA, Odebunmi EO. A novel zerovalent manganese for removal of copper ions: synthesis, characterization and adsorption studies. Appl Water Sci [Internet]. 2017;7(3):1409–1427.
- Dada OA, Adekola FA, Odebunmi EO. Kinetics and equilibrium models for sorption of Cu(II) onto a novel manganese nano-adsorbent. J Dispers Sci Technol [Internet]. 2016;37(1):119–133.
- Lagergren S. About the theory of so-called adsorption of soluble substances. Handlingar. 1898;24:1–39.
- Roginsky S, Ybz. The catalytic oxidation of carbon monoxide on manganese dioxide. Acta Phys Chem USSR. 1934;1:554.
- Pawar RR, Lalhmunsiama, Kim M, et al. Efficient removal of hazardous lead, cadmium, and arsenic from aqueous environment by iron oxide modified clay-activated carbon composite beads. Appl Clay Sci [Internet]. 2018;162:339–350. Available from: http://www.sciencedirect.com/science/article/pii/S0169131718302680
- Wan X, Zhan Y, Long Z, et al. Core@double-shell structured magnetic halloysite nanotube nano-hybrid as efficient recyclable adsorbent for methylene blue removal. Chem Eng J [Internet]. 2017;330:491–504.